DOI:
10.1039/D0RA00023J
(Paper)
RSC Adv., 2020,
10, 10666-10672
Evaluation of antioxidant modification on the functional and structural properties of EWP conjugates
Received
2nd January 2020
, Accepted 5th March 2020
First published on 12th March 2020
Abstract
The aim of this present study was to improve the oxidative stability of egg white protein (EWP) through catechin (CT) and epigallocatechin gallate (EGCG) covalent modification via an alkaline method at pH 9.0. Effects of CT and EGCG conjugation on the antioxidant activities, physicochemical and structural properties of EWP were comprehensively studied. The results indicated that CT and EGCG modification altered the isoelectric point value of EWP to lower pH, thus the solubility of EWP conjugates at pH 3.8 decreased, especially after EGCG conjugation. In addition, the antioxidant activities of EWP–CT and EWP–EGCG conjugates were 2.88 and 3.52 fold (2-diphenyl-1-picrylhydrazyl radical scavenging activities), 2.60 and 7.91 fold (ferric reducing powers) higher than that of the unmodified EWP. Moreover, the CT or EGCG conjugation resulted in an increase in alpha-helix formation with a decrease in the β-sheet formation, indicating that the secondary structure of EWP became more compact after CT or EGCG modification, and Trp and Tyr residues were involved into the conjugation reaction of EWP with CT or EGCG. Furthermore, CT and EGCG conjugation obviously improved the emulsifying stability of EWP, due to the improvement of the antioxidant activity after being modified by the CT or EGCG. In conclusion, CT or EGCG conjugation with EWP via an alkaline method was an effective way to improve the utilization value of EWP.
1 Introduction
Egg white protein (EWP) is valuable food ingredient due to its wonderful nutritional value and functional properties, such as high solubility over a wide pH range, good foaming, emulsifying and gel properties.1,2 Thus it has received great interest in the food processing industry. However, the application of the EWP in food processing is limited due to its weak antioxidant stability.3 As reported, polyphenols, widely distributed in natural plants, are widely accepted as natural antioxidants, which have numerous bio-activities and can delay or prevent oxidative damage by reactive phenolic hydroxyl groups.4 And the antioxidant properties of protein could be improved through an interaction of protein with polyphenol, especially through covalent linkage, which can lead to the changes in structure and functionality of proteins.5
Catechin (CT) and epigallocatechin gallate (EGCG) are the two main active antioxidant polyphenol components in tea, because they can effectively balance free radicals in the body by removing reactive oxygen species (ROS), NO, or reacting with ROS to form stable compounds.6 However, CT and EGCG are susceptible to oxidative degradation due to the external environment, such as light, high temperature and pH value. In addition, the antioxidant activity of CT and EGCG only persist for a relatively short time after ingestion due to rapid metabolism within the human body.7 To avoid the automatic oxidation and degradation of CT and EGCG, improve their stability and bio-availability, the research found that the CT/EGCG-biomolecular complex could prolong and improve the antioxidant activity of CT and EGCG.8,9 Therefore, the construction of the EWP–CT and EWP–EGCG conjugates might not only improve the oxidative stability of EWP, but also improve the bio-availability of CT and EGCG.
As reported, protein can react covalently with polyphenol through enzymatic, alkaline, and free radical methods. The free radical method has been studied extensively,10–12 and the results show that the reaction under neutral or mildly acidic condition could lead to the covalent binding of the protein and polyphenol. However, the free radical method might has the negative effects on the functional properties and toxicological safety of the modified proteins.12 In addition, enzymatic method can also be used to prepared the protein–polyphenol conjugates with strong scavenging activity against superoxide anion radicals, the popularization and application of the high-cost enzymatic method are limited. By contrast, protein–polyphenol conjugates, which synthesized via the alkaline method, have been reported to exhibit improved antioxidant activity, compared with the free radical method.13 However, to our best knowledge, no study has focused on the effect of CT or EGCG conjugation via alkaline method on the antioxidant, structure and physicochemical properties of EWP.
Therefore, this study aimed to evaluate how CT or EGCG conjugation would affect the antioxidant, structure and physicochemical properties of EWP under alkaline condition. Initially, EWP was covalent cross-linking with CT or EGCG via alkaline method, then the antioxidant activity of the EWP conjugates were characterized using 2-diphenyl-1-picrylhydrazyl (DPPH) radical scavenging activity and ferric reducing power. And the structural properties of the EWP conjugates were analyzed using surface hydrophobicity (H0), circular dichroism (CD) spectroscopy and fluorescence spectroscopy analysis. Moreover, to better understand the CT or EGCG conjugation on the physicochemical properties of EWP in food, the solubility and emulsion properties were also evaluated. This study could provide and enlarge the new applications of EWP in functional foods industry.
2 Materials and methods
2.1 Materials
Desugared egg white protein (EWP) powders were obtained from Rongda Poultry Co., Ltd. (Anhui province, China). Catechin (CT, purity ≥ 98%) and epigallocatechin gallate (EGCG, purity ≥ 98%) were purchased from Macklin Co., Ltd. (Shanghai, China). 2-Diphenyl-1-picrylhydrazyl (DPPH), 8-anilino-1-naphthalenesulfonic acid (ANS), Coomassie Brilliant Blue R-250 and dialysis bags (MWCO 10000 Da) were purchased from Sigma Chemical Co. (St. Louis, USA). All the other chemical reagents were of analytical grade and purchased from Sinopharm Chemical Reagent Co., Ltd. (Shanghai, China).
2.2 Pre-treatment of EWP
Firstly, the 5% EWP solution was stirred magnetically in an ice water bath for 6 h, followed by centrifugation at 5000 rpm for 10 min. The resulting supernatant was collected and lyophilized in a FD-1A-50 freeze dryer (Bo Yi Kang Experimental Instrument Co., Ltd., Beijing, China) and subsequently stored in air- and water-tight containers at room temperature for further modification and analysis. The protein content of the treated EWP powders was determined by the Kjeldahl method to be 88.93% ± 1.18%.
2.3 Synthesis of EWP–CT and EWP–EGCG conjugates
The EWP conjugates, which modified by CT or EGCG were prepared according to the alkaline method described by Rawel et al. (2001) with slight modifications.13 The prepared EWP (1%) and CT or EGCG solutions were mixed at a ratio of 10
:
1 (w/w), and the pH of the mixture was adjusted to 9.0 using NaOH (0.1 mol L−1), then this mixture was maintained at 25 °C under continuous stirring (70 rpm) for 24 h. Un-reacted CT or EGCG was removed by dialysis at room temperature for 48 h. The water was changed six times until no free CT or EGCG existed in the system, which determined by an 1800 UV-vis spectrophotometer (Shimadzu, Kyoto, Japan). Finally, the resulting solutions were freeze-dried and then kept in a desiccator for further analysis. The EWP conjugates which modified by CT and EGCG were labeled as EWP–CT conjugates and EWP–EGCG conjugates, respectively. EWP without CT or EGCG modification was used as the control.
2.4 Characterization of EWP–CT and EWP–EGCG conjugates
2.4.1 Total CT and EGCG contents in the EWP conjugates. The Folin–Ciocalteu method was used to measure the total CT or EGCG contents in the EWP conjugates according to the operating steps described by Vatai et al. (2009) with some modifications.14 Briefly, 0.5 mL of sample (0.8 mg mL−1) was mixed with 2.5 mL of Folin–Ciocalteu reagent (0.2 mol L−1) for 5 min. Subsequently, 4 mL of Na2CO3 (7.5%, w/v) was added into the mixture and allowed to rest for 2 h in the dark. The absorbance of this mixture was measured at 760 nm using an 1800 UV-vis spectrophotometer (Shimadzu, Kyoto, Japan). The control solution was prepared under the same conditions without sample. The total CT or EGCG contents in the conjugates were expressed using the equation y = 8.9775x − 0.00005 (EGCG) and y = 10.188x − 0.008 (CT), respectively. The results are expressed as mg g−1 conjugates.
2.4.2 ζ potential determination. ζ potential of EWP, EWP–CT and EWP–EGCG conjugates were analyzed by dynamic light scattering (Zeta Nano ZS-90, Worcestershire, UK). Prior to each measurement, the samples were re-dissolved (1 mg mL−1) and centrifuged. And the pH of sample solutions after centrifugation was adjusted to 3, 4, 5 and 6 respectively.
2.4.3 Solubility. The solubility of the EWP, EWP–CT and EWP–EGCG conjugates was determined according to the method described by Salvador et al. (2009).15
2.4.4 Antioxidant analysis.
DPPH radical scavenging activity. The DPPH radical scavenging activity was determined according to the method described by Blois (1958) with some modifications.16 A 2 mL aliquot of each sample (0, 0.02, 0.04, 0.08, and 0.2 mg mL−1) was mixed with 2 mL of DPPH solution (0.125 mM) and kept in the dark at 25 °C for 30 min. The absorbance of the remaining DPPH was determined colorimetrically at 517 nm. The scavenging activities of EWP, EWP–CT and EWP–EGCG conjugates are expressed using the following equation (eqn (1)): |
 | (1) |
where A0 is the absorbance of a standard prepared under the same conditions but without sample added, and A1 and A2 are the absorbances of polymeric samples, but the former is without DPPH solution.
Ferric reducing power. The abilities of the samples to reduce iron were determined using the method described by Yildirim et al. (2001) with some modifications.17 Briefly, a 10 mL aliquot of each sample (0.1, 0.2, 0.3, 0.4, and 0.5 mg mL−1) was mixed with 2.5 mL of phosphate buffer solution (PBS, 0.2 mol L−1, pH 6.6) and 2.5 mL of potassium ferricyanide (1%, w/v), and the mixtures were incubated at 50 °C for 20 min. Subsequently, 2.5 mL of trichloroacetic acid (10%, w/v) were added, and the reaction mixtures were centrifuged at 5000 rpm for 10 min. Finally, 2.5 mL of the supernatant was mixed with 2.5 mL of distilled water and 0.5 mL of FeCl3 (0.1%, w/v) and reacted for 10 min, and the absorbance at 700 nm was measured using an 1800 UV-vis spectrophotometer as described above. A higher absorbance indicated a higher ferric reducing power.
2.4.5 Surface hydrophobicity (H0). The H0 of each sample was determined using the method described by Kato and Nakai (1980).18
2.4.6 Emulsion properties. The emulsifying activity index (EAI) and emulsifying stability index (ESI) were determined according to the method described by Liu et al. (2015).19
2.4.7 Circular dichroism (CD) spectroscopy. The CD spectra of the samples were recorded in the range 190–250 nm using a Jasco J-815 CD spectrometer (Jasco Co., Tokyo, Japan) with a quartz cylindrical cell in 1 mm path length. The concentrations of the samples were maintained at 0.2 mg mL−1. The spectra were scanned at a speed of 100 nm min−1 and 0.1 nm bandwidth at room temperature under nitrogen flow.
2.4.8 Fluorescence spectroscopy.
Intrinsic fluorescence spectroscopy. The intrinsic fluorescence spectra were measured using an F-4500 Fluorophotometer (Hitachi, Ltd, Japan) at room temperature. EWP, EWP–CT and EWP–EGCG conjugates were dissolved in PBS (pH 7.4) to obtain 1 mg mL−1 sample solutions. The fluorescence was measured by exciting the protein at 280 nm and recording its emission spectra in the wavelength range of 300–500 nm, the widths of the excitation and emission slits were 5 and 2.5 nm respectively.
Synchronous fluorescence spectroscopy. Samples were prepared as described for intrinsic fluorescence spectroscopy. The synchronous fluorescence spectra were carried out using a setting of Δλ = 15 nm in the range of 260–340 nm (both excitation and emission slit widths were 5 nm) and a setting of Δλ = 60 nm in the range of 220–360 nm excitation (slit width was 10 nm, emission slit width was 2.5 nm).
2.5 Statistical analysis
All experimental data are presented as the mean values of data from two parallel experiments. A one-way ANOVA was used to identify the differences between the samples (P < 0.05).
3 Results and discussion
3.1 Modification of EWP by CT and EGCG
It is note that polyphenols are easily prone to oxidation under alkaline condition (pH 9.0) in the presence of oxygen to form semi-quinone radicals, which subsequently rearrange to quinones. These reactive intermediate products readily react with nucleophilic residues in protein side chains.5 Thus, in order to improve the oxidative stability of EWP, the covalent cross-linking of CT or EGCG to EWP by alkaline method was achieved in this study.
Firstly, the concentration of CT or EGCG in the EWP covalent reaction has also been optimized. When the concentration of CT or EGCG added is too low, the corresponding CT or EGCG bounding is too low, thus leading to the lower antioxidant activity of EWP. By contrast, when the concentration of CT or EGCG is too high, the protein will be denatured and precipitated (data not provided). Therefore, in order to prepare EWP conjugates with strong antioxidant activity, as many CT or EGCG as possible were added under the condition that no EWP precipitation occurred. By optimizing, the EWP and CT or EGCG were mixed at a ratio of 10
:
1 (w/w).
The total CT and EGCG contents in the EWP conjugates were shown in Table 1. As seen, the total CT content in the EWP–CT conjugates was 43.37 mg g−1 polymer, while the EGCG content in the EWP–EGCG conjugates was 80.40 mg g−1 polymer, indicating that EGCG is more easily covalent cross-linking with EWP compared to CT through the alkaline method. Dubeau et al. (2010)20 found that the binding affinity of polyphenols with proteins mainly depend on their molecular size, the larger molecular size of the polyphenol showed higher binding affinity for reaction towards proteins because of higher binding sites available for interaction in case of large phenolic compounds, consistent with the results of our research.
Table 1 Some characteristics of EWP before or after conjugation with CT and EGCGa
|
Total phenolic contents (mg g−1 sample) |
pI values |
Solubility |
H0 |
pH 3.8 |
pH 7.0 |
Values are means SD (n = 2). Different superscript letters in the table indicate a significant difference (p < 0.05). |
EWP |
— |
4.71 ± 0.29b |
68.23 ± 3.29b |
87.32 ± 2.85a |
313.96 ± 3.09c |
EWP–CT |
43.37 ± 4.01a |
4.51 ± 0.36b |
62.83 ± 2.64b |
90.27 ± 1.37b |
227.65 ± 7.78b |
EWP–EGCG |
80.40 ± 5.23b |
3.90 ± 0.24a |
28.24 ± 3.76a |
96.23 ± 2.53c |
102.92 ± 4.11a |
In addition, the influence of CT and EGCG conjugation on the surface charge of EWP was investigated by measuring the isoelectric point (pI) of EWP, EWP–CT and EWP–EGCG conjugates. As seen in Table 1, the pI value of the EWP was 4.71, and the pI values of the EWP–CT and EWP–EGCG conjugates was 4.51 and 3.90, respectively. It indicated that the CT and EGCG conjugation altered the surface charge of the EWP. This finding was consistent with the results observed when grafting chlorogenic acid to bovine serum albumin.21 Moreover, the pI values of the EWP–CT and EWP–EGCG conjugates were slightly lower than that of the EWP, which may be attributed to the introduction of the phenolic hydroxyl groups to the EWP,5,22 and the more phenolic hydroxyl groups introduced into the EWP conjugates, the lower pI of the conjugates was. For the alkaline method, the quinoid structures resulting from the oxidation of the phenolic compounds can undergo covalent reactions with the nucleophilic side chains, such as lysine, cysteine and tryptophan residues,23–25 which may also change the number of ionisable residues and decrease the pI of the EWP.
Protein solubility, as a prerequisite for other functional properties such as emulsifying properties is the most important physicochemical property of protein. As proved above, the conjugation of CT and EGCG has altered the pI value of the EWP, which could change their pH-solubility profiles.26 Thus the effects of CT and EGCG conjugation on the solubility of EWP were also evaluated. In this study, the pH of 3.8 and 7.0, which the pH values of the most beverage and egg products, were chosen to assess the solubility of the EWP and EWP–polyphenol conjugates. As seen, the solubility of EWP–CT and EWP–EGCG conjugates were lower than that of the EWP at pH of 3.8, while slightly higher than that of the EWP at pH of 7.0, which attributed to the lower pI values of EWP after CT or EGCG conjugation. In addition, as we all known that a protein usually has the least solubility at pI value, thus the solubility of the EWP–CT and EWP–EGCG conjugates were different from that of the EWP at different pH values.
Moreover, the H0 of proteins can impact the physicochemical and structural properties of protein. Table 1 shows the effect of CT and EGCG conjugation on H0 of the EWP. As seen, the CT or EGCG conjugation has inevitably decreased the H0 of EWP. In addition, the H0 of EWP–EGCG conjugate was 102.92, which lower than that of the EWP–CT conjugate (227.65), it may be due to the covalent binding of polyphenols to hydrophobic amino acids such as tryptophan, and the introduction of polyphenols brought hydrophilic groups such as hydroxyl and carboxyl groups, thus leading to the decrease of the H0. Ju et al. (2020)27 also obtained the similar conclusions in soy protein–anthocyanin complex, which prepared by alkaline method. However, Bao et al. (2019)28 reported an increased H0 of flaxseed protein isolate after conjugation with ferulic acid via alkaline method. This difference might due to exposure of the different number of hydrophobic/hydrophilic amino acid on the surface of the protein after conjugation with polyphenol.
3.2 Antioxidant activity analysis
In this study, DPPH radical scavenging activity and ferric reducing power were used to determined the antioxidant activities of EWP before and after CT or EGCG conjugation. The DPPH reagent is a stable organic free radical with the maximum absorption wavelength of 517 nm, and its absorbance has a linear relationship with the concentration. When a free radical scavenger is added thereto, DPPH can accept an electron or hydrogen radical to become a stable diamagnetic molecule, thus reduce the absorbance, and the antioxidant ability is proportional to the scavenging of DPPH.29 As shown in Fig. 1(A), the DPPH radical scavenging activity of EWP was 25.08% at a protein concentration of 0.20 mg mL−1, while that of EWP–CT and EWP–EGCG conjugates were 72.19% and 88.24%, respectively under the same concentration, 2.88 and 3.52 fold higher than that of the EWP, suggesting that the antioxidant capacity of EWP was markedly enhanced by the CT or EGCG conjugation under the alkaline condition. Furthermore, it is interesting to note that the DPPH radical scavenging activity of EWP–EGCG conjugates was much higher than that of EWP–CT conjugates at the same concentration, which may be due to the higher content of EGCG in the EWP–polyphenol conjugates than that of CT.
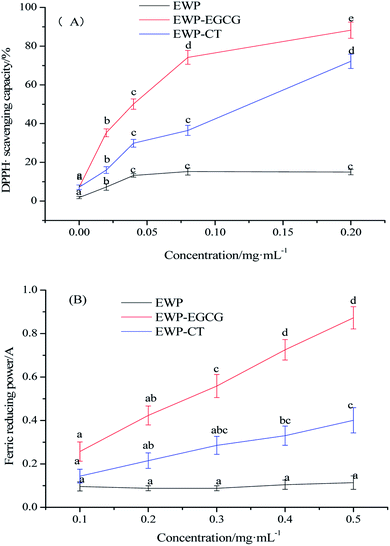 |
| Fig. 1 Antioxidant activities of EWP and EWP–polyphenols conjugates ((A) DPPH˙ scavenging capacity/%; (B) ferric reducing power). | |
Ferric reducing power of EWP and EWP conjugates were also evaluated. The antioxidant in the sample can reduce the ferric iron of potassium ferricyanide to ferrous iron. The ferrous iron further reacts with ferric chloride to produce Prussian blue with the maximum absorbance at 700 nm. Therefore, measuring the absorbance at 700 nm can indirectly reflect the reducing ability of the antioxidant. The greater the absorbance, the stronger the ferric reducing ability.30 As shown in Fig. 1(B), the ferric reducing power of the EWP, EWP–CT and EWP–EGCG conjugates were 0.11, 0.40, 0.87, respectively at a protein concentration of 0.50 mg mL−1. The activities of the EWP–CT and EWP–EGCG conjugates were 2.60 to 7.91 fold higher than the unmodified EWP at a protein concentration of 0.1–0.50 mg mL−1, suggesting that the EGCG conjugation improved the ferric reducing power of the EWP. In addition, the ferric reducing power of EWP–EGCG conjugates was more than two times higher than that of EWP–CT conjugates at the same concentration. It indicated that the reducing power of EWP was remarkably enhanced by EGCG modification than that of CT.
According to the results of DPPH radical scavenging activity and ferric reducing power, the antioxidant activities of EWP were significantly improved after CT or EGCG conjugation, especially EWP–EGCG conjugates. As reported, a greater number of hydroxyl group substituents in a phenol structure is thought to yield superior antioxidant activity.31 In addition, there are eight phenolic hydroxyl groups of each EGCG and four phenolic hydroxyl groups and one alcoholic hydroxyl group of each CT. Due to the difference in hydrophobicity and the number and position of hydroxyl groups,32 EGCG shows a better antioxidant power than CT,33 which is also beneficial to have higher antioxidant activities of EWP–EGCG conjugates. Thus, the conjugation of antioxidant CT or EGCG onto the EWP was a potential method for the preparation of antioxidative EWP.
3.3 Emulsifying activity and stability analysis
Emulsions have poor thermodynamic stability due to the large interfacial area between the oil phase and water phase. EWP, as the main emulsifying agents in food industry, can localize itself at the oil/water interface to reduce the interfacial tension, are usually incorporated in the formulations to assure stable emulsions.34 As reported, the conjugation of protein and polyphenol can enhance the emulsifying properties of a protein. Thus in this study, the EAI and ESI of unmodified EWP and EWP–polyphenol conjugates are shown in Fig. 2. EAI and ESI are important parameters generally used to investigate the emulsifying properties of proteins. EAI, the relative surface coverage of a protein on an oil globules was used to evaluate the activity of a protein to lower the interfacial tension at the oil–water interface. ESI mainly estimate its relative stability of a emulsion system. In general, the smaller size of the emulsion, the higher of the stability. As seen, the EAI of the unmodified EWP was 178.94 m2 g−1, the EAI was decreased to 161.33 m2 g−1 for EWP–EGCG conjugate compared with the unmodified EWP, and EAI of EWP–CT conjugate was decreased to 139.22 m2 g−1, which might attribute to the decrease of the H0 (shown in Table 1) after modified by CT and EGCG via alkaline method. In addition, compared with the unmodified EWP, ESI of the EWP–CT and EWP–EGCG conjugates were all increased, suggesting that the emulsifying stability of EWP was obviously improved after CT and EGCG conjugation. Wang et al. (2016)35 indicated that the zein hydrolysate incorporated with tannic acid could significantly enhance the stability of the oil–water emulsions, which was mainly attributed to the improvement of the antioxidant activity after modified by the polyphenols.
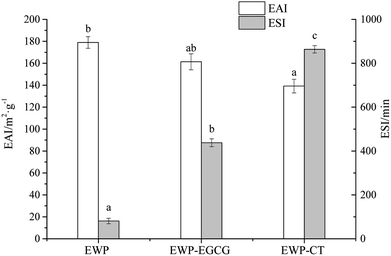 |
| Fig. 2 EAI and ESI of EWP and EWP–polyphenols conjugates. | |
3.4 CD analysis
The far-UV (190–250 nm) spectra of CD is a fast and accurate technique can be used to study the secondary structural changes of EWP in the solution before and after modified by CT or EGCG. As shown in Fig. 3, the far-UV spectra of EWP exhibited a negative peak in the region of 207–210 nm, which is mainly characteristic of α-helix of proteins, and the CT or EGCG conjugation caused a change in band intensity and wavelengths in the α-helix of EWP, and the same changes in the other peak intensity and shape in the far-UV CD of EWP, indicating that the secondary structure of EWP was changed after CT or EGCG conjugation. In addition, the secondary structure (α-helix, β-sheet, β-turn and random coil) contents of the EWP and EWP–polyphenol conjugates were analyzed by a curve-fitting programme software CDpro using CONTIN method. Table 2 shows the secondary structure contents of the EWP and EWP–polyphenol conjugates. As seen, the CT and EGCG conjugation resulted in an increase in α-helix with a decrease in the contents of the β-sheet, indicating that the secondary structure of the EWP–polyphenol conjugates became more compact after CT or EGCG modification. Similar results were also observed when grafting EGCG and chlorogenic acid (CA) to lactoferrin.19 However, Zhou et al. (2020)36 reported that the conjugation of EGCG with soybean protein isolate through alkaline method would cause a decrease in the α-helix structure, which might be due to the different properties of the protein and polyphenol, as well as the methods which chosen to synthesize the protein–polyphenol conjugates.
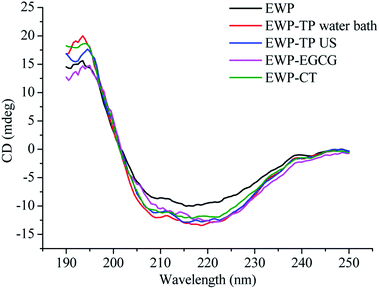 |
| Fig. 3 Far-UV CD spectra of EWP and EWP–polyphenol conjugates. | |
Table 2 Secondary structure of EWP and EWP–polyphenol conjugatesa
Secondary structural contents/% |
α-Helix |
β-Sheet |
β-Turn |
Random coil |
Values are means SD (n = 2). Different superscript letters in the table indicate a significant difference (p < 0.05). |
EWP |
15.5 ± 0.39a |
33.3 ± 2.75a |
20.8 ± 2.07a |
30.5 ± 2.71a |
EWP–CT |
17.5 ± 1.03ab |
30.9 ± 2.27a |
21.1 ± 1.93a |
30.5 ± 2.64a |
EWP–EGCG |
18.6 ± 0.31b |
29.0 ± 1.98a |
21.6 ± 2.12a |
30.7 ± 1.89a |
3.5 Intrinsic fluorescence spectroscopy analysis
EWP is an endogenous fluorescent substance, tryptophan (Trp), tyrosine (Tyr), and phenylalanine (Phe) which in EWP molecular structure, are the main fluorescent amino acids. Trp (279 nm), Tyr (275 nm) and Phe (257 nm) have different fluorescence excitation and emission spectra due to the different chromophores in their side chains. Therefore, intrinsic fluorescence spectra at a excited wavelength of 280 nm was used to detect changes in the microenvironment of Trp and to judge the conformational changes of EWP and EWP–polyphenol conjugates. As shown in Fig. 4, the FIs of the EWP decreased significantly after CT or EGCG conjugation, it may attribute to the interactions between aromatic rings in polyphenols and Trp from the EWP, which leading to Trp fluorescence quenching,37 indicating that Trp was most likely to be involved in the covalent conjugation of the EWP with CT or EGCG. In addition, the maximum emission wavelength for the unmodified EWP was 335 nm, and the maximum emission was red shifted to 337 nm and 342 nm in EWP–CT and EWP–EGCG conjugates, respectively. The red shift is indicative of the microenvironment around the Trp of the EWP becoming more hydrophilic.38 In summary, from the Trp fluorescence quenching and the red shift observed in the maximum fluorescence emission of EWP and EWP–polyphenol conjugates, which suggesting that the CT or EGGC conjugation might lead to the tertiary structure changes and denaturation of EWP.
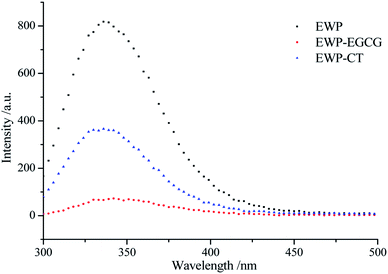 |
| Fig. 4 Intrinsic fluorescence of EWP and EWP–polyphenols conjugates. | |
3.6 Synchronous fluorescence spectroscopy analysis
Synchronous fluorescence spectra which can provide the tertiary structure changes of EWP before and after CT and EGCG conjugation were characterized using a fluorometer. The Δλ values of 15 and 60 nm represent for Tyr and Trp, respectively. The synchronous fluorescence spectra of EWP and EWP–polyphenol conjugates are shown in Fig. 5A and B. As seen, the FIs of Trp residue is stronger than that of Tyr, which indicates that Trp contents in the EWP are higher than that of Tyr. when Δλ = 15 nm, the synchronous FIs of EWP–CT and EWP–EGCG conjugates decreased by 53.11% and 93.93%, respectively, indicating that the involvement of Tyr residue in the covalent reaction between EWP and CT and EGCG, especially in the covalent reaction of EWP with EGCG. In addition, the λmax of EWP was 292 nm, and there was red shifts in the EWP–CT conjugate (2 nm) and EWP–EGCG conjugate (5 nm), suggesting the polarity around Tyr residues in EWP–polyphenol conjugates increased and more Tyr residues exposed to the surrounding water, especially the Tyr in the EWP–EGCG conjugate.
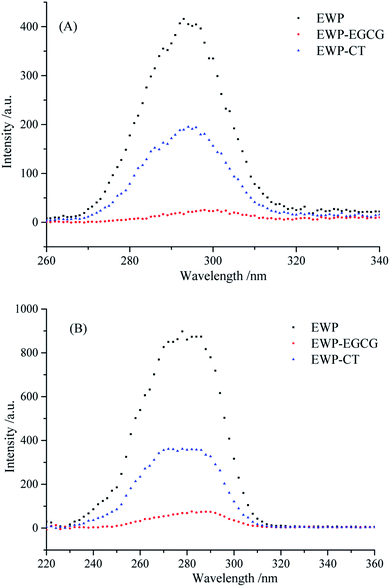 |
| Fig. 5 Synchronous fluorescence of EWP and EWP–polyphenols conjugates ((A) Δλ = 15 nm; (B) Δλ = 60 nm). | |
Similarly, as seen in Fig. 5B, when Δλ = 60 nm, the synchronous FIs of EWP–CT and EWP–EGCG conjugates decreased by 59.26% and 91.47%, respectively, indicating that Trp residues are involved in the conjugation of EWP with CT and EGCG, and more of Trp was contributed to the covalent reaction of EWP with EGCG than that in the reaction with CT. Moreover, as seen, conspicuous red shift was observed in the maximum emission wavelength of EWP–EGCG conjugate, suggesting Trp residues in the EWP–EGCG conjugates existed in a more hydrophilic microenvironment.
4 Conclusion
In summary, EWP–CT and EWP–EGCG conjugates were successfully prepared by alkaline method. The results of antioxidant assays confirmed that CT and EGCG modification was an effective way to improve the antioxidant activities of EWP. In addition, CT or EGCG conjugation altered the isoelectric point value of EWP to lower pH. And the CT or EGCG modification on the EWP resulted in an increase in α-helix with a decrease in the β-sheet. Moreover, these modification on the EWP had significantly improved the emulsifying stability of EWP depending on the improvement of the antioxidant activities. Our results provided an efficient method for the synthesis of the antioxidant EWP via alkaline method.
Conflicts of interest
There are no conflicts to declare.
Acknowledgements
This work was supported by the National Key Research and Development Program of China (2018YFD0400303), Program of National Natural Science Foundation of China (31701530).
References
- L. Campbell, V. Raikos and S. R. Euston, Nahrung, 2003, 47, 369–376 CrossRef CAS PubMed.
- E. D. N. S. Abeyrathne, X. Huang and D. U. Ahn, Poultry Sci., 2018, 97, 1462–1468 CrossRef CAS PubMed.
- X. Duan, M. Li, J. Shao, H. Chen, X. Xu and Z. Jin, Food Hydrocolloids, 2018, 75, 223–228 CrossRef CAS.
- A. Salvi, P. Carrupt, J. Tillement and B. Testa, Biochem. Pharmacol., 2001, 61, 1237–1242 CrossRef CAS PubMed.
- T. H. Quan, B. Soottawat, S. L. Thanasak, K. B. Amjad and M. Sajid, Trends Food Sci. Technol., 2019, 91, 507–517 CrossRef CAS.
- N. E. Es-Safi, S. Ghidouche and P. H. Ducrot, Molecules, 2007, 12, 2228–2258 CrossRef CAS PubMed.
- M. A. Manso, M. Miguel, J. Even, R. Hernandez, A. Aleixandre and R. Lopez-Fandino, Food Chem., 2008, 109, 361–367 CrossRef CAS PubMed.
- L. Gu, Y. Su, M. Zhang, C. Chang, J. Li, D. J. Mcclements and Y. J. Yang, Food Res. Int., 2017, 96, 84–93 CrossRef CAS PubMed.
- M. P. Almajano, M. E. Delgado and M. H. Gordon, Food Chem., 2007, 101, 126–130 CrossRef CAS.
- Y. Fan, Y. Liu, L. Gao, Y. Zhang and J. Yi, Food Chem., 2018, 261, 283–291 CrossRef CAS PubMed.
- D. S. Lee, J. Y. Woo, C. B. Ahn and J. Y. Je, Food Chem., 2014, 148, 97–104 CrossRef CAS PubMed.
- L. Gu, C. Pan, Y. Su, R. Zhang, H. Xiao, D. J. McClements and Y. J. Yang, J. Agric. Food Chem., 2018, 66, 1649–1657 CrossRef CAS PubMed.
- H. M. Rawel, J. Kroll and S. Rohn, Food Chem., 2001, 72, 59–71 CrossRef CAS.
- T. Vatai, M. Skerget and Z. Knez, J. Food Eng., 2009, 90, 246–254 CrossRef CAS.
- P. Salvador, M. Toldra, E. Saguer, C. Carretero and D. Pares, Food Hydrocolloids, 2009, 23, 1654–1659 CrossRef CAS.
- M. S. Blois, Nature, 1958, 29, 1199–1200 CrossRef.
- A. Yildirim, A. Mavi and A. A. Kara, J. Agric. Food Chem., 2001, 49, 4083–4089 CrossRef CAS PubMed.
- A. Kato and S. Nakai, Biochim. Biophys. Acta, 1980, 624, 13–20 CrossRef CAS.
- F. Liu, C. Sun, W. Yang, F. Yuan and Y. Gao, RSC Adv., 2015, 5, 15641–15651 RSC.
- S. Dubeau, G. Samson and H. A. Tajmir-Riahi, Food Chem., 2010, 122, 539–545 CrossRef CAS.
- H. M. Rawel, S. Rohn, H. P. Kruse and J. Kroll, Food Chem., 2002, 78, 443–455 CrossRef CAS.
- Z. Wei, W. Yang, R. Fan, F. Yuan and Y. Gao, Food Hydrocolloids, 2015, 45, 337–350 CrossRef CAS.
- S. Rohn, H. M. Rawel and J. Kroll, J. Agric. Food Chem., 2004, 52, 4725–4729 CrossRef CAS PubMed.
- S. Oliver, O. Vittorio, G. Cirillo and C. Boyer, Polym. Chem., 2016, 7, 1529–1544 RSC.
- N. G. Kroll, H. M. Rawel and S. Rohn, Food Sci. Technol. Res., 2003, 9, 205–218 CrossRef.
- F. Liu, C. Ma, Y. Gao and D. J. McClements, Compr. Rev. Food Sci. Food Saf., 2017, 16, 76–95 CrossRef CAS.
- M. Ju, G. Zhu, G. Huang, X. Shen, Y. Zhang, L. Jiang and X. Sui, Food Hydrocolloids, 2020, 99, 105329 CrossRef.
- P. L. Bao, B. Wang, B. Zisu and B. Adhikari, Food Chem., 2019, 293, 463–471 CrossRef PubMed.
- N. Cotelle, J. L. Bernier, J. P. Catteau, J. Pommery, J. C. Wallet and E. M. Gaydou, Free Radical Biol. Med., 1996, 20, 35–43 CrossRef CAS.
- P. D. Duh, J. Am. Oil Chem. Soc., 1998, 75, 455–465 CrossRef CAS.
- C. G. Heijnen, G. R. Haenen, F. A. Van Acker, D. V. W. J. Van and A. Bast, Toxicol. In Vitro, 2001, 15, 3–6 CrossRef CAS PubMed.
- G. Cao, E. Sofic and R. L. Prior, Free Radical Biol. Med., 1997, 22, 749–760 CrossRef CAS PubMed.
- A. Rashidinejad, E. J. Birch, D. Sun-Waterhouse and D. W. Everett, Food Chem., 2014, 156, 176–183 CrossRef CAS PubMed.
- D. J. McClements, Annu. Rev. Food Sci. Technol., 2010, 1, 241–269 CrossRef CAS PubMed.
- Y. H. Wang, Z. L. Wan, X. Q. Yang, J. M. Wang, J. Guo and Y. Lin, Food Hydrocolloids, 2016, 54, 40–48 CrossRef CAS.
- S. D. Zhou, Y. F. Lin, X. Xu, L. Meng and M. S. Dong, Food Chem., 2020, 309, 125718 CrossRef PubMed.
- P. Suryaprakash, R. P. Kumar and V. Prakash, Macromol, 2000, 27, 219–228 CrossRef CAS.
- S. Chen, N. Zhang and C. H. Tang, Food Hydrocolloids, 2016, 61, 102–112 CrossRef CAS.
|
This journal is © The Royal Society of Chemistry 2020 |