DOI:
10.1039/C9RA10991A
(Paper)
RSC Adv., 2020,
10, 11918-11928
Optimization of extraction parameters of Pleurotus eryngii polysaccharides and evaluation of the hypolipidemic effect
Received
29th December 2019
, Accepted 13th March 2020
First published on 24th March 2020
Abstract
The hot water extraction of polysaccharides from the fruiting body of Pleurotus eryngii was studied. In this paper, according to single-factor experiments, a response surface methodology and the Box–Behnken design were applied to optimize the extraction parameters of Pleurotus eryngii polysaccharides (PEP). The optimal extraction conditions were as follows: a temperature of 79 °C, a time of 3.11 h, a ratio of water to fruiting bodies of 52.6 mL g−1. Under these parameters, the yield of PEP was 7.53%. When mice with hyperlipidemia were administered low, medium or high doses of PEP, their body weight was reduced compared with the model group, and the degree of weight loss was proportional to the dose. At the 16th week of PEP treatment, blood lipid biochemical parameters such as total cholesterol, triglycerides, low-density lipoprotein-cholesterol, aspartate aminotransferase, and alanine aminotransferase levels were all decreased. However, high-density lipoprotein-cholesterol levels increased after PEP treatment. Histopathological examination of the liver showed that low, medium and high doses of PEP had a certain liver protective effect. High-dose PEP treatment had the best effect in regard to lipid-lowering and liver protection. In addition, the metabolome of the mice was analyzed by LC-MS, and the results indicated that C16 sphinganine can be used as a potential biomarker, which displayed significant differences among the six groups. In conclusion, the possible metabolic mechanism of the PEP on lipid-lowering was closely related to sphingolipid metabolism.
1. Introduction
In recent years, the prevalence of obesity in the world has been steadily increasing.1 Accumulation of excessive or abnormal fat is called obesity,2 which can cause many health and psychological problems.3,4 As far as health is concerned, many problems are caused by obesity, such as muscular, skeletal and developmental delays,5,6 sleep disorders,7 central obesity, hypertension, dyslipidemia, hyperglycemia, coronary heart disease, stroke and some cancers,8 and dysfunction of the metabolic system by a series of mechanisms, including initiation of endothelial dysfunction, increasing free radical production, lipid peroxidation and production of inflammatory cytokines.9–11 Both psoriasis and psoriatic arthritis (PsA)12 are strongly correlated with obesity and metabolic syndrome (MetS). Metabolic syndrome (MetS) represents a group of clinical and laboratory abnormalities that augment the risk of developing atherosclerosis, cardiovascular disease, type 2 diabetes mellitus (T2DM) and all-causes of mortality. Obesity may also lead to lack of self-confidence, lack of self-esteem, and declining quality of life,13–15 which results in many social problems.16 For the sake of physical and mental health, many lipid-lowering drugs on the market have been widely used, such as statins, because they can not only reduce high cholesterol, low-density lipoprotein cholesterol, apolipoprotein B and triglyceride levels but can also raise high-density lipoprotein cholesterol levels. Thus, the effect of reducing fat in the circulatory system is achieved.
However, many problems still exist, and it has been reported that these synthetic drugs have serious side effects,17,18 such as liver and kidney damage, dependence, antagonism and the emergence of drug resistance, nausea, diarrhea, hair loss, abnormal glucose tolerance, memory loss, memory decline, confusion and so on. Such as, simvastatin is a fine-grained powder and is typical synthetic lipid-lowering drugs.19 Because of its limited dissolution rate, its absorption and pharmacological effects are unstable20 and its oral bioavailability is poor. Therefore, it is necessary to develop a natural lipid-lowering drug without side effects. It has been reported that polysaccharides that are biologically active substances can be extracted from an edible mushroom and may be effective in the prevention and treatment of hyperlipidemia, cardiovascular disease and metabolic syndrome caused by obesity.21,22 As biologically active substances, polysaccharides have been reported to have lipid-lowering effects.23 In addition, Pleurotus eryngii as a kind of edible and medicinal edible fungus has rich nutritional value,24 also, compared with other Chinese herbal medicines, their price is low, and the raw material is easy to obtain. Some reports have confirmed that PEP have been shown to inhibit lipid accumulation by experiments on RAW264.7 cells.25 However, some problems still exist in regard to Pleurotus eryngii polysaccharides (PEP) inhibits the accumulation of lipids mechanism that was not uncertain. This article is the first to analyze mouse urine by an LC-MS method and to study the possible lipid-lowering mechanisms of PEP in regard to metabolic pathways.
In this paper, the extraction process of PEP was first optimized to accumulate polysaccharides for subsequent experiments. Secondly, a high-fat animal model was established to explore the lipid-lowering and anti-obesity activities of PEP. It was an important research topic in the field of nutrition to find the natural functional substance that interfere with obesity from the edible fungi used in medicine and food. Finally, the LC-MS method was used to analyze the lipid-lowering mechanism of PEP from the metabolic level of mice, which has a significant contribution for further understanding the functional activity, in vivo transport and mechanism of PEP. At the same time, it provides a new idea for PEP, which as main functional ingredients in the development of nutritional and healthy products. In conclusion, PEP can be used as a promising natural active substance to assist in the treatment of hyperlipidemia.
2. Materials and methods
2.1 Materials and reagents
Pleurotus eryngii were purchased from the market in Xi'an City, Shanxi Province, China. Ordinary feed and high-fat feed were purchased from Beijing Aokang Biological Technology Co., Ltd., while 4% paraformaldehyde was purchased from Beijing Suo Laibao Technology Co., Ltd., ALT (alanine aminotransferase), AST (aspartate aminotransferase), TG (triglycerides), TC (total cholesterol), HDL-C (high-density lipoprotein-cholesterol), and LDL-C (low-density lipoprotein-cholesterol) kits were purchased from Shenzhen Minray Co., Ltd., MS-grade acetonitrile and formic acid were purchased from Thermo Fisher Scientific (USA). Ultrapure water was purified using a Milli-Q system (Millipore, Bedford, MA, USA).
2.2 Instruments and equipment
The equipment used in this study include a BS460 automatic biochemical analyzer from Shenzhen Minray Co., Ltd., a Eppendorf 5418 desktop high-speed freeze centrifuge was purchased from Germany, an electronic balance from Shanghai Balance Instrument Factory, a Type 101-2 constant temperature drying oven from the Shanghai Experimental Instrument Factory, a Re52-4 rotary evaporator from Shanghai Huxi Analytical Instrument Factory, an Hh-sh-4 electrothermal constant-temperature water bath pot from Beijing Chang'an Scientific Instrument Factory, a RM2135 paraffin slicer from Leica Company in Germany, an Olympus microphotography operating system from Japan, and an HPLC-Q-Orbitrap from the HRMS Thermoelectric company in Germany.
2.3 Single-factor design for crude polysaccharides
The fresh fruiting bodies of Pleurotus eryngii were cut into pieces and dried in a 40 °C oven, then they were pulverized and passed through a 40-mesh screen. Extraction temperature (X1: 40, 50, 60, 70, and 80 °C), extraction time (X2: 1, 2, 3, 4, and 5 h) and ratio of liquid to raw material (X3: 10, 20, 30, 40, and 50 mL g−1) were conditions tested for extraction. We applied a single-factor method to study the yield of Pleurotus eryngii polysaccharides, using 5 g dried Pleurotus eryngii powder for each experiment which were performed in triplicate. Pleurotus eryngii polysaccharide (PEP) was obtained using hot water extraction and ethanol precipitation. The specific hot water extraction method was that in the three parameters of temperature, time, and material–liquid ratio, one factor was changed while the other factors were kept constant in each experiment. Place the beaker in a water bath and heat for a certain temperature and time to extract PEP. The extracted solution was centrifuged at 8000 rpm for 10 min, and the supernatant was collected and incubated with 95% ethanol (the volumetric ratio of supernatant
:
ethanol = 1
:
4) for 24 h at 4 °C. After centrifugation, the supernatant was discarded and the precipitate was collected. Crude polysaccharides were obtained by removing the protein with Sevage reagent (volumetric ratio of N-butanol
:
chloroform = 1
:
4) after dialysis (8–14
000 kDa) and freeze-drying. The crude polysaccharide content was measured by the phenol-sulfuric acid method using glucose as a standard. The extraction yield was calculated with the following formula:26 |  | (1) |
2.4 Experimental design of the response surface methodology (RSM)
Based on the results of the single-factor experiments, a three-variable, three-level 17-run Box–Behnken (BBD) was designed to determine the optimal levels of extraction variables (Tables 1 and 2) by Design Expert software (Version 8.0.6). The experimental data were fitted to the following quadratic polynomial model:26–29 |  | (2) |
where Y is the response variable (the yield of Pleurotus eryngii polysaccharide), α0 is the constant coefficient, αi is the linear coefficient, αij is the interaction coefficients, αii is the squared coefficients, Xi and Xj are the coded independent variables, and Xi, Xj and Xi2 represent the interaction and quadratic terms.
Table 1 Independent variables and their levels used in the Box–Behnken design
Variables |
Level |
−1 |
0 |
1 |
Extraction temperature, X1 (°C) |
70 |
80 |
90 |
Extraction time, X2 (h) |
2 |
3 |
4 |
Ratio of liquid to raw materials X3 (mL g−1) |
40 |
50 |
60 |
Table 2 Box–Behnken design and the extraction rate of PEP
No. |
Temperature X1 (°C) |
Time X2 (h) |
Ratio of liquid to raw materials X3 (mL g−1) |
Yield Y (%) |
1 |
0 |
0 |
0 |
7.52 |
2 |
1 |
0 |
1 |
6.85 |
3 |
0 |
0 |
0 |
7.49 |
4 |
−1 |
0 |
1 |
7.01 |
5 |
1 |
−1 |
0 |
5.93 |
6 |
0 |
0 |
0 |
7.52 |
7 |
−1 |
1 |
0 |
6.31 |
8 |
0 |
0 |
0 |
7.52 |
9 |
0 |
1 |
1 |
6.53 |
10 |
0 |
−1 |
1 |
5.32 |
11 |
0 |
1 |
−1 |
4.91 |
12 |
0 |
0 |
0 |
7.52 |
13 |
1 |
1 |
0 |
6.32 |
14 |
0 |
−1 |
−1 |
4.51 |
15 |
−1 |
−1 |
0 |
3.51 |
16 |
1 |
0 |
−1 |
6.13 |
17 |
−1 |
0 |
−1 |
5.26 |
2.5 Anti-hypercholesterolemic effect of PEP
2.5.1 Animal experiments.
All animal treatments were conducted strictly in accordance with the National Institutes of Health Guidelines for the Care and Use of Laboratory Animals. Animal experiments were approved by the Administrative Committee of the Experimental Animal Care and Use at Xi'an Medical University.
A total of 60 male Kunming mice (20 ± 2 g), 4 weeks old, were purchased from the Experimental Animal Centre of Xi'an Jiaotong University (Xi'an, China). The mice were housed in cages (5 mice each) with controlled standard temperature (22 ± 1 °C), humidity (50–60%), and lighting (12 h light/dark cycle). Animals were allowed free access to water and standard rodent chow. After a week of adaptive feeding, the animals were randomly divided into 6 groups with 10 mice each. The normal control group (NC) was fed a normal diet from weeks 1–8. The hyperlipidemia model control group (MOD), positive group (PC) and the three PEP groups were fed a high-fat rodent diet with 45% of calories from fat: casein (200 g, 800 kcal), L-cysteine (3 g, 12 kcal), corn starch (72.8 g, 291 kcal), maltodextrin (100 g, 400 kcal), sucrose (172.8 g, 691 kcal), cellulose (200 g, 500 kcal), soybean oil (25 g, 225 kcal), lard (177.5 g, 1598 kcal), mineral mix S10026 (10 g, 0 kcal), dicalcium phosphate (13 g, 0 kcal), calcium carbonate (5.5 g, 0 kcal), potassium citrate (16.5 g, 0 kcal), vitamin mix V10001 (10, 40 kcal), choline bitartrate (2 g, 0 kcal) during the first eight weeks. In the next 9–16 weeks, the normal control group (NC) was treated with a normal diet and gavaged with saline 0.1 mL per 10 g per day, the hyperlipidemia model control group (MOD) was fed the high-fat diet and gavaged with saline 0.1 mL per 10 g per day, the positive control group (PC) was fed the high-fat diet and gavaged with simvastatin at 0.1 mL per g per day, and the three PEP groups were fed the high-fat diet and gavaged with different doses of PEP (50, 100 and 200 mg per kg per day) in 0.1 mL per 10 g per day. The diet and treatment plans for the six groups are listed in detail in Table 3.
Table 3 Diet and treatment plans for mouse groups n = 10 each
Group |
Week 1–8 |
Week 9–16 |
NC: normal control |
Normal diet |
Normal diet and saline |
MOD: model control |
High-lipid diet |
High-lipid diet and saline |
PC: simvastatin-treatment |
High-lipid diet |
High-lipid diet and simvastatin (10 mg per kg per day) |
LPEP: low dose of PEP (50 mg kg−1) |
High-lipid diet |
High-lipid diet and PEP (50 mg per kg per day) |
MPEP: medium dose of PEP (100 mg kg−1) |
High-lipid diet |
High-lipid diet and PEP (100 mg per kg per day) |
HPEP: high dose of PEP (200 mg kg−1) |
High-lipid diet |
High-lipid diet and PEP (200 mg per kg per day) |
2.5.2 Body weight.
The experiment began with recording the body weight of the mice, and then they were weighed once a week to measure the changes in their body weight. At the end of the experiment, the mice were weighed again and dissected to remove the liver, and one part of the liver was fixed in 4% paraformaldehyde while the other half was stored at −80 °C.
2.5.3 Sample preparation.
Under fasting conditions, blood samples were collected from the tail vein of the mice before and during the experiment every two weeks. Blood samples were centrifuged at 3000 rpm for 10 min at 4 °C to obtain the serum; then, the samples were divided into two equal parts into two new centrifuge tubes. One half was used for the detection of biochemical indicators, including TC, TG, AST, ALT, LDL-C, and HDL-C levels, and the other half were stored at −80 °C. The mice were placed in a metabolic cage, and their urine was collected and centrifuged at 3000 rpm for 10 min at 4 °C to remove the lower layer. The supernatant was stored at −80 °C until analysis.
2.5.4 Serum biochemical parameter determination and histological morphology examinations.
The serum levels of total TC, TG, LDL-C, HDL-C, AST, ALT were tested with an automatic biochemical analyzer BS-460 from Mindray. All procedures complied with the manufacturer's guidelines.
The histological morphological structure of the hepatic tissue was observed after hematoxylin and eosin (H&E) staining through an Olympus microphotography operating system, Japan.30
2.6 Metabolomics analysis
The urine samples were thawed on ice, and we removed all precipitated material by centrifugation at 12
000 rpm for 10 min at 4 °C. The supernatant 200 μL was transferred to a new tube and mixed with 1000 μL of an acetonitrile and water solution (ratio by volume acetonitrile
:
water = 4
:
1) and then vortexed for 60 s; then it was centrifuged at 12
000 rpm for 10 min at 4 °C, and the supernatant was passed through a 0.22 μm membrane then transferred to a vial for LC-MS analysis.
Urine samples were separated via an UHPLC C18 column (50 mm × 2.1 mm i.d., 1.9 μm) at 30 °C. The mobile phase consisted of acetonitrile containing 0.1% formic acid (A) and 0.1% aqueous formic acid (B). The gradient procedure was as follows: 0–2 min with 0% A, 2–10 min with 0–15% A, 10–14 min with 15–30% A, 14–17 min with 30–95% A, 17–19 min with 95% A, and 19–20 min with 95–0% A, followed by a re-equilibration step for 5 min. The injection volume was 5 μL, and the flow rate was 0.3 mL min−1. The key operating parameters of the mass spectrometry were set as follows: a spray voltage of 3.5 kV in positive ion mode, a sample cone voltage of 40 V, a source offset voltage of 80 V, a source temperature of 120 °C, a desolvation temperature of 450 °C, a nitrogen gas flow of 900 L h−1, 10 L h−1 for the cone gas flow, and a data acquisition rate of 0.3 s per scan. MS data were acquired in centroid mode from m/z 100 to 1500 in full scan. All data were recorded and processed by Thermo Scientific Xcalibur3.0 software (Thermo Fisher Scientific).
2.7 The statistical analysis
Unless otherwise indicated, all data are expressed as the means ± standard deviation (SD). Statistical differences were evaluated using ANOVA, and significant differences between the tested groups were determined using Duncan's post hoc test (SPSS 17.0). Metabolic raw data was processed using Compound Discover software from Thermo Fisher LC-MS, and the data was imported into SIMCA software to analysis.
3. Results and discussion
3.1 Single-factor experimental analysis
By single-factor experiments, we studied the efficiency of different extraction temperatures, extraction time and ratio of liquid to raw material on the yield of PEP, and the results are shown in Fig. 1A–C. Extraction temperature was a key factor that influenced the extraction efficiency. The yield of PEP in the range of 50–80 °C gradually increased and reached a maximum of 7.52% at 80 °C; also, when the temperature exceeded 80 °C, the yield of PEP slightly decreased (Fig. 1A). The reason for this result may be that as the temperature increased, the molecular motion accelerated, the solubility increased, and the corresponding yield also increased.31,32 When the temperature exceeded 80 °C, the raw materials was restricted, resulting in a decrease in polysaccharide yield. An extraction time of 0.5–3 h increased the yield of PEP tremendously, while for 3–5 h, the yield decreased with time. At 3 h, it reached a maximum level of 6.98% (Fig. 1B). The extraction yield then decreased with prolongation of the extraction time. The possible reason for a reduction in the yield was that the structure of the polysaccharides may have been damaged after spending a long time at a high temperature.31 Similarly, the ratio of liquid to raw material was another factor that significantly affected the extraction efficiency parameter. When the ratio of the liquid to raw material was 50
:
1, the yield of PEP reached a maximum amount of 6.89%. Ratios any higher than 50
:
1 resulted in a decreased polysaccharide yield (Fig. 1C).
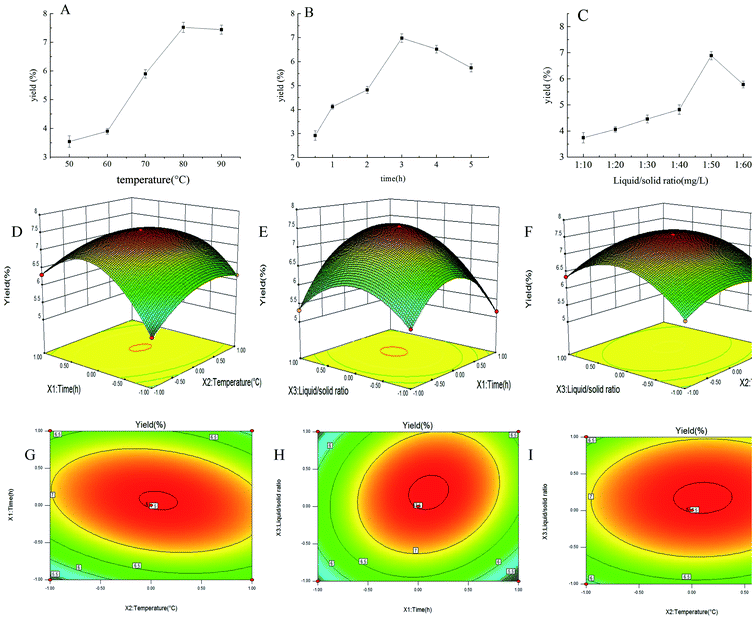 |
| Fig. 1 Effects of extraction parameters on the yield of PEP; response surface plots and contour plots. (A) Effect of extraction temperature (°C) on yield of PEP; (B) effect of extraction time (h) on yield of PEP; (C) effect of liquid/solid ratio (mL g−1) on yield of PEP; (D) response surface plots showing the interactive effects of extraction time (X1) and extraction temperature (X2); (E) response surface plots showing the interactive effects of extraction time (X1) and liquid/solid ratio (X3); (F) response surface plots showing the interactive effects of extraction temperature (X2) and liquid/solid ratio (X3); (G) contour plots showing the interactive effects of X1 and X2; (H) contour plots showing the interactive effects of X1 and X3; (I) contour plots showing the interactive effects of X2 and X3. | |
3.2 Optimization of the polysaccharides extraction conditions
To optimize the extraction parameters and analyze the interactive effects of the three factors on the extraction rate, a BBD under RSM was used, as shown in Table 2. Under different interactions, the PEP had different yields distributed in the range of 3.51–7.52%. The optimal three factors were an extraction time of 3.11 h, an extraction temperature of 79 °C, and a ratio of material to liquid of 1
:
52.6. The yield was then 7.53%. The PEP yield and the second-order polynomial equations for the three variables were analyzed by the Design-Expert software. The equation was as follows:
Y = 0.75 + 0.09X1 + 0.17X2 + 0.28X3 − 0.32X1X2 + 0.53X1X3 + 0.32X2X3 − 0.48X12 − 1.11X22 − 0.83X32 |
An analysis of variance analysis found the model group was extremely significant (P < 0.0001) and the lack of fit was not obvious (p > 0.05), R2 = 0.9993, Radj2 = 0.9984, as shown in Table 4; thus, the model could make a scientific analysis of most of the changes in response values. In addition, the low CV% = 0.54 value indicates that the experimental values were accurate and reliable (Xu & Wei, 2008 (ref. 28)). Therefore, the model had good experimental prediction ability within the range of experimental variables. From the overall factors, the primary items X1, X2, X3; the secondary items X12, X22, X32; and the interactive items X1X2, X1X3, X2X3 were considered to be extremely significant (p < 0.0001). The order of influence of the different variables was X1 < X2 < X3. That is to say, reaction time < reaction temperature < ratio of liquid to raw material.
Table 4 Results of the ANOVA of the response surface quadratic model
Source |
Sum of squares |
df |
Mean square |
F-value |
Prob > F |
Model |
11.81 |
9 |
1.31 |
1126.53 |
<0.0001 |
X
1
|
0.065 |
1 |
0.065 |
55.62 |
0.0001 |
X
2
|
0.23 |
1 |
0.23 |
195.55 |
<0.0001 |
X
3
|
0.63 |
1 |
0.63 |
543.19 |
<0.0001 |
X
1
X
2
|
0.40 |
1 |
0.40 |
346.12 |
<0.0001 |
X
1
X
3
|
0.011 |
1 |
0.011 |
9.46 |
0.0179 |
X
2
X
3
|
0.41 |
1 |
0.41 |
351.59 |
<0.0001 |
X
1
2
|
0.98 |
1 |
0.98 |
837.92 |
<0.0001 |
X
2
2
|
5.23 |
1 |
5.23 |
4485.19 |
<0.0001 |
X
3
2
|
2.93 |
1 |
2.93 |
2513.87 |
<0.0001 |
Residual |
8.155 × 10−3 |
7 |
1.165 × 10−3 |
|
|
Lack of fit |
6.275 × 10−3 |
3 |
2.092 × 10−3 |
4.45 |
0.0916 |
Pure error |
1.880 × 10−3 |
4 |
4.700 × 10−4 |
|
|
Cor total |
11.82 |
16 |
|
|
|
|
R
2 = 0.9971 |
|
R
adj
2 = 0.9934 |
CV% = 0.45 |
|
The 3D response surface and 2D contour plot of the effects of different single factors and interactions on PEP yield are shown in Fig. 1D–I. When the ratio of material to liquid was fixed at the 0 level, the effects of temperature (X1) and time (X2) and interaction on PEP production showed a curved surface, and the temperature (X1) was kept at 0 level; also, with an extension of time (X2), the yield increased at first and then decreased, as shown in Fig. 1D. Similarly, as shown in Fig. 1E and F, when the time and temperature were fixed at the 0 level, the influence of the corresponding two variables on the PEP yield were also curved surfaces. The shapes shown in the Fig. 1G–I contour maps were an ellipse, indicating that the interactions of the corresponding variables were significant.33
In summary, the optimized parameters were an extraction temperature of 79 °C, an extraction time of 3.11 h and a ratio of material to liquid of 1
:
52.6; also, the yield of PEP was 7.53%, which was close to the previously reported results,34 indicating that the optimization conditions were reliable. The polysaccharide content was determined to be 67.84%, which implied that the following studies were meaningful.
3.3 Vary of body weight
The changes in body weight of the different groups during the entire 16 week period are shown in Fig. 2 below. The high-fat model was established in 1–8 weeks. At the 8th week, there was a significant difference in body weight between the high-fat diet group and the normal control group, indicating that the model was successfully established. From 9–16 weeks, simvastatin and different doses of PEP were administered, as shown in Table 3. Compared to the normal control group and the high-fat model group, the body weight of the model group significantly increased, but treatment with PEP could inhibit the body weight gain and fat accumulation. The degree of inhibition was dose-dependent on the concentration of PEP. The high-dose PEP group had a significantly (p < 0.05) decreased body weight gain and accumulation of fat compared with the model control group.
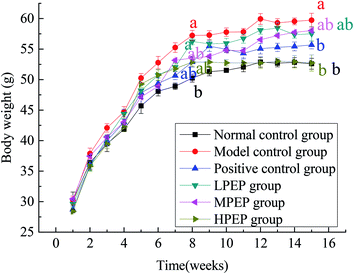 |
| Fig. 2 Changes in body weight in different groups of mice over 16 weeks. (a and b): the same letter indicates there were not significant difference between the groups, and different letters represent significant differences (p < 0.05). | |
3.4 Liver histological morphology examinations
HE staining results of liver from the different groups of mice were observed by an optical microscope, as shown in Fig. 3. Specifically, Fig. 3A–F are the liver morphology observed under a microscope of the normal control group, the model control group, the positive control group, the LPEP group, the MPEP group, and the HPEP group, respectively. From Fig. 3A, we can see that the liver cells morphology are arranged in a regular manner, and very small fat particles appear, showing no obvious liver lesions, after a 16 week normal diet. However, compared with the normal control group, in the high-fat model, a large number of large particles of fat vacuoles can be observed in the liver tissues as shown in Fig. 3B. The liver tissue morphology of the simvastatin treated positive control group was close to that of the normal group as shown in Fig. 3B. Different doses of PEP significantly reduced the number and volume of fat vacuoles in the liver cells compared with the model group. The high-dose PEP group had the best effect on fat granule reduction, even better than that of the long-term normal diet NC group. As shown in Fig. 3F, almost no fat particles are apparent after high doses of PEP. Both the LPEP group and the MPEP group also showed a reduced number and size of liver fat vacuoles, and the effect on the MPEP group was slightly better than that of the LPEP group. The fat particles and size, as shown in Fig. 3D, are fewer and smaller than those in Fig. 3C. The optical microscopy results of the liver tissue sections indicated that PEP can mitigate the peroxidation damage to the liver tissues and promote the recovery of the damaged liver tissues from the high-fat-diet-induced injury.
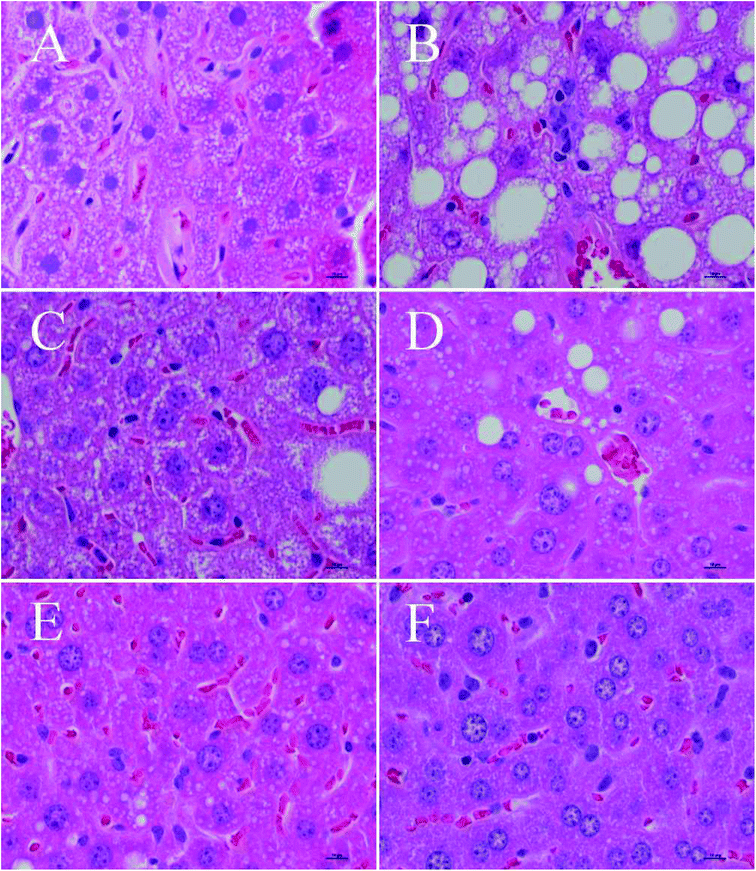 |
| Fig. 3 Morphological observation of liver tissue in different groups of mice (hematoxylin–eosin staining, 400×). (A) Normal control (NC) group, (B) model control (MC) group, (C) positive control (PC) group, (D) low-dose Pleurotus eryngii polysaccharide group (LPEP) group, (E) medium-dose Pleurotus eryngii polysaccharide (MPEP) group, (F): high-dose Pleurotus eryngii polysaccharide (HPEP) group. | |
3.5 Serum biochemical parameters
Hyperlipidemia is a disease characterized by an abnormal increase of total cholesterol (TC), triglycerides (TG), low-density lipoprotein-cholesterol (LDL-C), and alanine aminotransferase (ALT), aspartate aminotransferase (AST), and high-density lipoprotein-cholesterol (HDL-C) is decreased in the blood. Every 3 weeks, the biochemical parameters such as TC, TG, LDL-C, HDL-C, ALT and AST were measured in each group. While establishing a high-fat model, during the first 8 weeks, the normal control was given a normal diet and the other groups were given a high-fat diet. From Table 5, we can see that in the first week, TC, TG, LDL-C, HDL-C, ALT and AST were not significantly different (p > 0.05) between the normal diet group and the high-fat-diet groups. TC and LDL-C levels in the serum were significantly different (p < 0.05) between the normal control group (NC) and the model control group (Mod); however, for the normal control group (NC) compared with the PC group, the low-dose PEP group (LPEP), the moderate-dose PEP group (MPEP) and the high-dose PEP group (HPEP) were not significantly different (p > 0.05) in the fourth week. At week 7, TC, TG, LDL-C, ALT and AST levels in the high-fat diet groups (Mod, PC, LPEP, MPEP, HPEP groups) were significantly increased (p < 0.05), and the HDL-C level significantly decreased (p < 0.05) compared with the normal diet control group, which indicates that the high-fat diet groups had diet-induced changes in their serum lipid parameters that led to hypercholesterolemia. Therefore, the animal model of hyperlipidemia was successfully established. At the same time, the serum levels of TC, TG, LDL-C, ALT and AST in the mice in high-fat model group were increased by 64.7%, 32.2%, 48.4%, 81.4% and 78.5%, respectively, compared with the normal diet control group at the end of the diet induction.
Table 5 Effect of different doses of PEP on serum biochemical parameters induced by a high-fat diet in micea
|
Groups |
Time |
1 week |
4 week |
7 week |
10 week |
13 week |
16 week |
Data are expressed as the mean ± SD, the same letter represents no significant difference between the groups, different letters represent significant differences (p < 0.05).
|
TC (mmol L−1) |
NC |
1.31 ± 0.21a |
1.83 ± 0.26a |
1.84 ± 0.27a |
1.98 ± 0.19a |
2.03 ± 0.24a |
2.63 ± 0.36a |
MOD |
1.79 ± 0.23a |
2.96 ± 0.31b |
3.03 ± 0.30b |
2.89 ± 0.23b |
3.70 ± 0.31b |
4.07 ± 0.37b |
PC |
1.63 ± 0.31a |
2.84 ± 0.28ab |
3.03 ± 0.33b |
2.38 ± 0.31ab |
2.86 ± 0.24ac |
2.84 ± 0.25ac |
LPEP |
1.28 ± 0.43a |
2.76 ± 0.30ab |
3.01 ± 0.21b |
2.84 ± 0.33ab |
3.46 ± 0.43b |
3.29 ± 0.30ab |
MPEP |
1.39 ± 0.25a |
2.69 ± 0.25ab |
2.92 ± 0.31b |
2.74 ± 0.28ab |
3.26 ± 0.34b |
2.85 ± 0.24ac |
HPEP |
1.35 ± 0.34a |
2.77 ± 0.24ab |
3.10 ± 0.29b |
2.30 ± 0.24ab |
3.17 ± 0.31b |
2.73 ± 0.28ac |
TG (mmol L−1) |
NC |
0.35 ± 0.07a |
0.65 ± 0.13a |
0.68 ± 0.21a |
0.67 ± 0.09a |
0.81 ± 0.21a |
0.74 ± 0.03a |
MOD |
0.38 ± 0.12a |
0.62 ± 0.18a |
0.82 ± 0.16b |
0.85 ± 0.05b |
1.23 ± 0.11b |
1.38 ± 0.05b |
PC |
0.46 ± 0.10a |
0.6 ± 0.20a |
0.76 ± 0.13b |
0.62 ± 0.03ab |
0.53 ± 0.09c |
0.71 ± 0.10ac |
LPEP |
0.40 ± 0.09a |
0.54 ± 0.14a |
0.73 ± 0.18b |
0.68 ± 0.10ab |
0.66 ± 0.07c |
0.57 ± 0.09ac |
MPEP |
0.44 ± 0.17a |
0.49 ± 0.16a |
0.80 ± 0.11b |
0.67 ± 0.08ab |
0.58 ± 0.04c |
0.52 ± 0.11ac |
HPEP |
0.44 ± 0.13a |
0.53 ± 0.09a |
0.79 ± 0.10b |
0.63 ± 0.12ab |
0.56 ± 0.06c |
0.48 ± 0.08ac |
LDL-C (mmol L−1) |
NC |
0.156 ± 0.06a |
0.238 ± 0.10a |
0.287 ± 0.12a |
0.279 ± 0.08a |
0.271 ± 0.07a |
0.265 ± 0.03a |
MOD |
0.164 ± 0.09a |
0.286 ± 0.08ab |
0.426 ± 0.10b |
0.382 ± 0.09b |
0.413 ± 0.03b |
0.412 ± 0.03b |
PC |
0.168 ± 0.08a |
0.204 ± 0.09ab |
0.355 ± 0.09b |
0.326 ± 0.07ab |
0.336 ± 0.03ab |
0.318 ± 0.05ab |
LPEP |
0.172 ± 0.05a |
0.244 ± 0.12ab |
0.362 ± 0.05b |
0.328 ± 0.03ab |
0.333 ± 0.04ab |
0.301 ± 0.06ab |
MPEP |
0.162 ± 0.09a |
0.208 ± 0.08ab |
0.361 ± 0.06b |
0.316 ± 0.04ab |
0.323 ± 0.02ab |
0.291 ± 0.04a |
HPEP |
0.168 ± 0.09a |
0.216 ± 0.11ab |
0.358 ± 0.08b |
0.327 ± 0.02ab |
0.33 ± 0.05ab |
0.273 ± 0.02a |
HDL-C (mmol L−1) |
NC |
0.86 ± 0.09a |
2.38 ± 0.22a |
2.69 ± 0.18a |
1.15 ± 0.08a |
1.49 ± 0.32a |
2.028 ± 0.34a |
MOD |
1.18 ± 0.16a |
2.03 ± 0.10a |
2.07 ± 0.24ab |
1.95 ± 0.12ab |
1.601 ± 0.36ab |
1.601 ± 0.21b |
PC |
1.05 ± 0.15a |
2.50 ± 0.38a |
2.14 ± 0.13ab |
1.80 ± 0.26ab |
2.31 ± 0.25b |
2.31 ± 0.18ab |
LPEP |
0.88 ± 0.21a |
2.41 ± 0.18a |
2 ± 0.23ab |
2.13 ± 0.23b |
3.04 ± 0.43bc |
3.04 ± 0.30c |
MPEP |
0.96 ± 0.23a |
1.09 ± 0.05b |
1.48 ± 0.18bC |
2.18 ± 0.29b |
2.556 ± 0.33b |
2.56 ± 0.26ac |
HPEP |
0.9 ± 0.12a |
1.20 ± 0.17b |
1.62 ± 0.17bC |
1.61 ± 0.13ab |
3.05 ± 0.28bc |
3.05 ± 0.36ac |
ALT (U L−1) |
NC |
30.41 ± 1.52a |
39.44 ± 6.56a |
42.91 ± 2.62a |
43.53 ± 1.80a |
49.35 ± 5.21a |
57.56 ± 2.25a |
MOD |
33.24 ± 2.36a |
56.08 ± 3.81b |
77.84 ± 3.43b |
70.84 ± 2.01b |
86.67 ± 3.35b |
91.60 ± 4.23b |
PC |
29.68 ± 3.24a |
53.84 ± 5.63b |
63.72 ± 1.86b |
40.84 ± 4.00a |
45.36 ± 1.56a |
68.09 ± 3.51a |
LPEP |
28.99 ± 1.64a |
40.08 ± 6.12a |
77.40 ± 2.42b |
46.16 ± 3.12a |
50.32 ± 4.28a |
57.47 ± 3.01a |
MPEP |
32.12 ± 1.85a |
56.26 ± 3.25b |
62.28 ± 3.01b |
51.44 ± 3.42a |
47.96 ± 2.33a |
47.60 ± 2.21a |
HPEP |
31.21 ± 2.64a |
59.96 ± 3.15b |
69.68 ± 1.98b |
50.56 ± 2.46a |
43.11 ± 3.04a |
45.91 ± 1.87a |
AST (U L−1) |
NC |
46.32 ± 2.03a |
64.49 ± 2.12a |
73.31 ± 1.33a |
79.35 ± 2.04a |
89.35 ± 2.37a |
92.80 ± 2.32a |
MOD |
43.55 ± 2.18a |
68.52 ± 3.54a |
130.88 ± 1.64b |
154.44 ± 3.42b |
168.09 ± 6.12b |
196.40 ± 3.29b |
PC |
42.81 ± 2.64a |
76.60 ± 1.23a |
128.16 ± 2.30b |
84.04 ± 2.33a |
81.28 ± 3.36a |
104.71 ± 4.02a |
LPEP |
48.01 ± 1.39a |
88.52 ± 6.12a |
133.96 ± 2.41b |
114.28 ± 5.41ab |
96.44 ± 4.39a |
118.00 ± 3.18a |
MPEP |
47.62 ± 1.82a |
52.36 ± 4.24a |
138.36 ± 3.25b |
85.68 ± 3.84a |
79.04 ± 2.44a |
107.88 ± 1.64a |
HPEP |
45.23 ± 3.24a |
66.76 ± 3.25a |
129.40 ± 5.43b |
102.24 ± 2.87ab |
82.44 ± 1.86a |
97.41 ± 1.87a |
During weeks 9–16, simvastatin and different doses of PEP were administered to the corresponding groups of hyperlipidemic mice. The hypolipidemic effect of PEP was determined by evaluating serum TC, TG, LDL-C, HDL-C, ALT and AST every 3 weeks after the oral administration of 50, 100 or 200 mg kg−1 PEP. At the tenth week, the TC, TG and LDL-C levels between the normal control group and high-fat model group had significant differences (p < 0.05), but the simvastatin and different doses of PEP treatment groups compared with the high-fat model group did not show any significant differences (p > 0.05). With the increase of PEP administered time, up to the 16th week, the levels of TC, TG, and LDL-C of the PC group and the PEP administered groups were different from the values of the model group and close to the values corresponding to the NC group. The TC and LDL-C values of the HPEP treatment group were closest to those of the NC group. The values of TC, TG, and LDL-C in the treatment groups with different doses of PEP were reduced and dose-dependent (Table 5). At 13 and 16 weeks, the HDL-C levels in the PEP-treated group were significantly higher than in the model group and were dose-dependent. During the PEP treatment of the hyperlipidemic mice, the ALT content decreased significantly (p < 0.05) compared with the model group. The AST content at weeks 13 and 16 showed that it was significantly reduced in the PEP treatment group (P < 0.05) in comparison with the model group.
These results indicate that PEP had the ability to inhibit the activity of HMG-CoA and reduce cholesterol synthesis.35 One reason may be that PEP, through upregulating the ileum FXR and hepatic CYP7A1 genes expression and downregulating the expression of I-BABP and hepatic FXR, inhibited the entero-hepatic cycle of the bile acids and increased the metabolism and decomposition of cholesterol.36 Finally, we can speculate that there was a decrease of the fat synthesis rate and an increase of the fat hydrolysis rate by way of transcriptional upregulation of PPARα, PPARγ, Lpl and Lpic, thus speeding up the metabolism and decomposition of triglycerides.37
3.6 LC/MS analysis of metabolic profiling and biomarkers identification
PLS-DA, a supervised pattern recognition method, was used to further characterize the difference in the urinary metabolic between the groups. All the data were acquired from the NC, MOD, LPEP, MPEP and HPEP groups. Complete separation between the NC and Mod groups were observed in the score scatter 3D plot from the PLS-DA shown in Fig. 4, which indicated that there was a significant metabolic profiling difference between the two groups and that the high-fat-diet-induced hyperlipidemia model was successfully established.38Fig. 4 shows that the positive control group and the PEP treatment group were far from the model group, and the higher the dose in the PEP group, the further away it was from the model group. The results indicated that PEP had the effect of reducing hyperlipidemia.
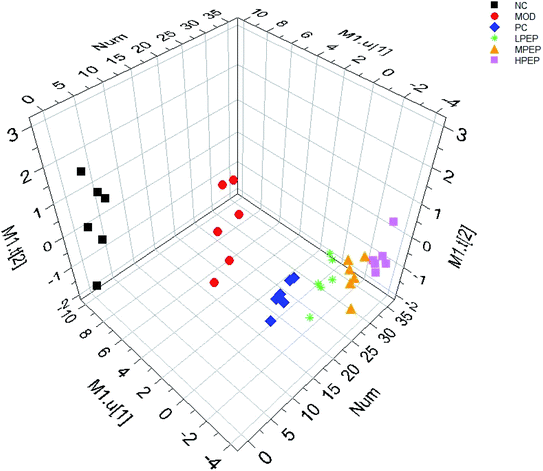 |
| Fig. 4 3D-PLS-DA score plots of NC, Mod, PC, LPEP, MPEP and HPEP groups in positive mode. | |
To reveal the potential biomarkers contributing the most to the difference among the groups, first, in the Human Metabolome Database (HMDB), we calculated the possible molecular formulas of interests by high-accuracy quasi-molecular ion within a mass error of 5 ppm. Then, the potential biomarkers were manually verified based on a mass search. The metabolites with a VIP value above 1.5 and a P value below 0.05 were considered as potential biomarkers that discriminated among the groups. The potential biomarkers are summarized in Table 6 with their corresponding retention time, accurate molecular mass, related trends and related pathway.
Table 6 Potential biomarkers identified and variation trends of hyperlipidemia mice in positive modea
Mass |
Formula |
Name |
VIP value |
Trend |
Related pathway |
MOD/NC |
PC/MOD |
LPEP/MOD |
MPEP/MOD |
HPEP/MOD |
MOD/NC: model versus control, #P < 0.05, ##P < 0.01. LPEP/MOD: LPEP versus model; MPEP/MOD: MPEP versus model, HPEP/MOD: HPEP versus model *P < 0.05, **P < 0.01.
|
273.27 |
C16H35NO2 |
C16Sphinganine |
1.68 |
↑# |
↓ |
↓ |
↓* |
↓* |
Sphingolipid metabolism |
330.06 |
C10H12N5O6P |
cAMP |
1.45 |
↑# |
↓ |
↓ |
↓ |
↓ |
Purine metabolism |
196.03 |
C5H4N4O3 |
Uric acid |
1.37 |
↓# |
↑ |
↑ |
↑ |
↑ |
Purine metabolism |
166.05 |
C8H7NO3 |
Formylanthranilic acid |
1.31 |
↑# |
↓ |
↓ |
↓ |
↓ |
Tryptophan metabolism |
154.09 |
C8H11NO2 |
Dopamine |
1.32 |
↑# |
↓ |
↓ |
↓ |
↓ |
Tyrosine metabolism |
117.02 |
C4H6O4 |
Succinate |
1.27 |
↓# |
↑ |
↑ |
↑ |
↑ |
TCA cycle |
184.06 |
C8H9NO4 |
4-Pyridoxate |
1.21 |
↑# |
↓ |
↓ |
↓ |
↓ |
Vitamin B6 metabolism |
176.10 |
C6H13N3O3 |
L-Citrulline |
1.09 |
↓# |
↑ |
↑ |
↑ |
↑ |
Arginine and proline metabolism |
243.06 |
C9H12N2O6 |
Uridine |
1.03 |
↑# |
↓ |
↓ |
↓ |
↓ |
Pyrimidine metabolism |
C16 sphinganine is a metabolite related to ceramide, which had alterations in the urine among the six groups. Comparing the NC group with the MOD group, C16 sphinganine was detected to be significantly increased, which indicated that sphingolipid metabolism turned out to be abnormal in response to hyperlipidemia.39 C16 sphinganine was decreased after simvastatin and PEP treatment and decreased significantly in the HPEP and MPEP groups, which implied that PEP treatment could show favorable inhibition of hyperlipidemia; thus, the reason for the inhibition of hyperlipidemia might be associated with the biomarker C16 sphinganine found in this research. It has been previously reported that the abnormal accumulation of esterification of cholesterol leads to obviously increased levels of C16 sphinganine in the urine of mice.40 Therefore, C16 sphinganine can be reliably used as a metabolic biomarker associated with lipid lowering. In addition, the key enzyme involved in sphingolipid metabolism of sphingomyelinases (SMases) is associated with ceramide production and bioactive lipid cascades.41 It has been reported that C16 sphingosine accumulation is associated with upregulation of SMase. Detailed mechanisms of the inhibition of hyperlipidemia by PEP needs further study.
Sphingolipids play important roles in cell growth, cell differentiation, cell apoptosis and vital signal transduction pathways.42–44 Sphingolipid metabolism disorders contribute to the development of NAFLD (non-alcoholic fatty liver disease) in multiple ways involving overweight, inflammation, insulin resistance, and oxidative stress. The mRNA expression of neutral and acid SMases and sphingosine levels is increased in the adipose tissue of obese mice, confirming the involvement of ceramide in the pathophysiology of obesity. PEP may increase the rate of fat decomposition, inhibit the rate of fat synthesis, and regulate the obesity induced by a high-fat diet through a combination of metabolic pathways such as fatty acid metabolism, amino acid metabolism, sphingolipid metabolism, and the TCA cycle in collaboration to affect the hyperlipidemia mice.
4. Conclusions
In this study, single factors and corresponding surface methods were used to optimize the processing parameters of polysaccharides from PEP. When the highest yield of polysaccharide was 7.52%, the required extraction temperature was 79 °C, the extraction time was 3.11 h, and the ratio of material to liquid was 1
:
52.6. The serum levels of TC, TG, LDL-C, AST and ALT in the mice treated with PEP were significantly reduced; at the same time, HDL-C levels were significantly increased at 16 weeks. The results suggested that the biochemical parameters of hypercholesterolemic mice can be normalized via the gavage of PEP for 8 weeks. A high-fat-diet-induced hyperlipidemia mice model was established successfully, and the anti-hyperlipidemic effect of PEP was evaluated by a metabolomics approach. The urine of each group of mice was analyzed by LC-MS method, and we found that C16 sphinganine can be used as a potential biomarker, which changed under the PEP treatment. This paper is the first to explore the therapeutic effect and metabolic mechanism of PEP on hyperlipidemia mice through biochemistry, histopathology and urinary metabolomics. These results showed that PEP could effectively treat hyperlipidemia mice and have a certain protective effect on the liver. The mechanism of PEP lipid-lowering may be through an effect on sphingolipid metabolism. Therefore, PEP can be used as a promising natural active substance for the adjuvant treatment of hyperlipidemia.
Conflicts of interest
There was no conflict of interest among authors: all authors agreed that this manuscript be submitted to RSC Advances.
Acknowledgements
This work was supported by the National Natural Science Foundation of China (No. 31401633, 31800328), the Science and Technology Department of Shaanxi Province (No. 2018JQ3017, 2018ZDXM-NY-054, 2017NY-146) and the Microbial Drug Engineering Laboratory of Xi'an City, Shaanxi Province, China.
References
- R. B. A. Kolsi, A. B. Gara and R. Chaaben,
et al., Anti-obesity and lipid lowering effects of Cymodocea nodosa sulphated polysaccharide on high cholesterol-fed-rats, Arch. Physiol. Biochem., 2015, 121(5), 1–8 Search PubMed.
- R. M. Baños, E. Oliver, J. Navarro, M. D. Vara, A. Cebolla and E. Lurbe,
et al., Efficacy of a cognitive and behavioral treatment for childhood obesity supported by the ETIOBE web platform, Psychol. Health Med., 2019, 1–11 Search PubMed.
- C. Tang, K. Zhang, Q. Zhao and J. Zhang, Effects of dietary genistein on plasma and liver lipids, hepatic gene expression, and plasma metabolic profiles of hamsters with diet-induced hyperlipidemia, J. Agric. Food Chem., 2015, 63, 7929–7936 CrossRef CAS PubMed.
- D. W. Haslam and W. P. James, Obesity, 2005, 366(9492), 1197–1209 Search PubMed.
- X. Wang, D. Zhao and Y. Cui,
et al., Proinflammatory macrophages impair skeletal muscle differentiation in obesity through secretion of tumor necrosis factor-α via sustained activation of p38 mitogen-activated protein kinase, J. Cell. Physiol., 2018 DOI:10.1002/jcp.27012.
- B. Gance-Cleveland, H. Aldrich and S. Schmiege,
et al., Virtual obesity collaborative with and without decision-support technology, Int. J. Qual. Health Care, 2016, 29 DOI:10.1093/intqhc/mzw029.
- I. Narang and J. L. Mathew, Childhood Obesity and Obstructive Sleep Apnea, J. Nutr. Metab., 2012, 1–8 Search PubMed.
- D. W. Haslam and W. P. James, Obesity, 2005, 366(9492), 1197–1209 Search PubMed.
- S. Min, L. Hayley, J. M. Andrew and Q. S. Xiao, Blueberry as a source of bioactive compounds for the treatment of obesity, type 2 diabetes and chronic inflammation, J. Funct. Foods, 2017, 30, 16–29 CrossRef.
- L. Chen, R. Chen, H. Wang and F. Liang, Mechanisms linking inflammation to insulin resistance, Int. J. Endocrinol., 2015, 508409 Search PubMed.
- E. P. Neale, M. J. Batterham and L. C. Tapsell, Consumption of a healthy dietary pattern results in significant reductions in C-reactive protein levels in adults: a meta-analysis, Nutr. Res., 2016, 36(5), 391–401 CrossRef CAS PubMed.
- E. Klingberg, A. Bilberg and S. Björkman,
et al., Weight loss improves disease activity in patients with psoriatic arthritis and obesity: an interventional study, Arthritis Res. Ther., 2019, 21 DOI:10.1186/s13075-019-1810-5.
- V. Snowdon-Carr, Psychological Approaches in the Treatment of Obesity: Pathogenesis, Diagnosis, and Treatment, Obesity, 2019 DOI:10.1007/978-3-319-46933-1_30.
- N. Yazdani, V. Sayed and M. Hosseini,
et al., Body image & psychological well-being in morbid obesity relationship between body image and psychological well-being in patients with morbid obesity, Int. J. Community Nurs. Midwifery, 2018, 6(2), 175–184 Search PubMed.
- L. E. Hayward, L. R. Vartanian and R. T. Pinkus, Weight Stigma Predicts Poorer Psychological Well-Being Through Internalized Weight Bias and Maladaptive Coping Responses, Obesity, 2018 DOI:10.1002/oby.22126.
- E. Y. Lee, Epidemic obesity in children and adolescents: risk factors and prevention, Front. Med., 2018, 12(6), 658–666 CrossRef PubMed.
- L. Chris, A. D. Wilson and V. Elisa, Atorvastatin Activates Skeletal RyR1 Channels: Towards Reducing Statin Side-Effects, Biophys. J., 2018, 114(3), 470 Search PubMed.
- A. Ju, C. S. Hanson and E. Banks,
et al., Patient beliefs and attitudes to taking statins: systematic review of qualitative studies, Br. J. Gen. Pract., 2018 DOI:10.3399/bjgp18X696365.
- N. A. Kasim, M. Whitehouse and C. Ramachandran,
et al., Molecular properties of WHO essential drugs and provisional biopharmaceutical classification, Mol. Pharm., 2004, 1, 85–96 CrossRef CAS PubMed.
- A. A. Ambike, K. R. Mahadik and A. R. Paradkar, Spray-dried amorphous solid dispersions of simvastatin, a low Tg drug: in vitro and in vivo evaluations, Pharm. Res., 2005, 22, 990–998 CrossRef CAS PubMed.
- L. Chen, Y. Zhang and O. Sha,
et al., Hypolipidaemic and hypoglycaemic activities of polysaccharide from Pleurotus eryngii in Kunming mice, Int. J. Biol. Macromol., 2016 DOI:10.1016/j.ijbiomac.2016.09.094.
- A. C. Burke, B. G. Sutherland and D. E. Telford,
et al., Intervention with citrus flavonoids reverses obesity, and improves metabolic syndrome and atherosclerosis in obese Ldlr/mice, J. Lipid Res., 2018, 59(9) DOI:10.1194/jlr.m087387.
- H. Wei, S. Yue, S. Z. Zhang and L. Lu, Lipid-Lowering Effect of the Pleurotus eryngii (King Oyster Mushroom) Polysaccharide from Solid-State Fermentation on Both Macrophage-Derived Foam Cells and Zebrafish Models, Polymers, 2018, 492 DOI:10.3390/polym10050492.
- B. Z. Zhang, K. T. Inngjerdingen, Y. F. Zou, F. Rise, T. E. Michaelsen, P. S. Yan and B. S. Paulsen, Characterisation and immunomodulating activities of exo-polysaccharides from submerged cultivation of Hypsizygus marmoreus, Food Chem., 2014, 163(3), 120–128 CrossRef CAS PubMed.
- J. J. Chen, Y. Y. Yong, M. C. Xing, Y. F. Gu, Z. Zhang, S. Z. Zhang and L. Lu, Characterization of polysaccharides with marked inhibitory effect on lipid accumulation in Pleurotus eryngii, Carbohydr. Polym., 2013, 97, 604–613 CrossRef CAS PubMed.
- Y. Liu, Y. Zhou and M. Liu,
et al., Extraction optimization, characterization, antioxidant and immunomodulatory activities of a novel polysaccharide from the wild mushroom Paxillus involutus, Int. J. Biol. Macromol., 2018, 112, 326–332 CrossRef CAS PubMed.
- J. L. Ren, Optimization of extraction process of Glycyrrhiza glabra polysaccharides by response surface methodology, Carbohydr. Polym., 2008, 74(4), 858–861 CrossRef.
- J. H. Xu and C. Wei, Optimization of extraction process of crude polysaccharides from wild edible BaChu mushroom by response surface methodology, Carbohydr. Polym., 2008, 72(1), 67–74 CrossRef.
- Y. Zhu, Q. Li, G. H. Mao, Y. Zou, W. W. Feng, D. H. Zheng, W. Wang, L. L. Zhou, T. X. Zhang, J. Yang, L. Q. Yang and X. Y. Wu, Optimization of enzyme-assisted extraction and characterization of polysaccharides from Hericium erinaceus, Carbohydr. Polym., 2014, 101, 606–613 CrossRef CAS PubMed.
-
P. Dey, Haematoxylin and Eosin Stain of the Tissue Section, Basic and Advanced Laboratory Techniques in Histopathology and Cytology, 2018, ch. 8, pp. 69–79, DOI:10.1007/978-981-10-8252-8.
- L. Yang, Y. L. Cao, J. G. Jiang, Q. S. Lin, J. Chen and L. Zhu, Response surface optimization of ultrasound-assisted flavonoids extraction from the flower of Citrus aurantium L. var.Amara Engl, J. Sep. Sci., 2010, 33(9), 1349–1355 CrossRef CAS PubMed.
- M. Wettasinghe and F. Shahidi, Evening primrose meal: a source of natural antioxidants and scavenger of hydrogen peroxide and oxygen-derived free radicals, J. Agric. Food Chem., 1999, 47(5), 1801–1812 CrossRef CAS PubMed.
- P. Yu and X. Chao, Statistics-based optimization of the extraction process of kelp polysaccharide and its activities, Carbohydr. Polym., 2013, 91(1), 356–362 CrossRef CAS PubMed.
- J. Chen, Y. Yong and M. Xing,
et al., Characterization of polysaccharides with marked inhibitory effect on lipid accumulation in Pleurotus eryngii, Carbohydr. Polym., 2013, 97(2), 604–613 CrossRef CAS PubMed.
- T. Qiu, X. Ma and M. Ye,
et al., Purification, structure, lipid lowering and liver protecting effects of polysaccharide from Lachnum YM281, Carbohydr. Polym., 2013, 98(1), 922–930 CrossRef CAS PubMed.
- V. García-Mediavilla, C. Villares, J. M. Culebras, J. E. Bayón and J. González-Gallego, Effects of dietary β-cyclodextrin in hypercholesterolaemic rats, Pharmacol. Toxicol., 2003, 92, 94–99 CrossRef PubMed.
- N. Takahashi, T. Kawada, T. Goto, T. Yamamoto, A. Taimatsu and N. Matsui,
et al., Dual action of isoprenols from herbal medicines on both PPARγ And PPARα in 3T3-L1 adipocytes and HepG2 hepatocytes, FEBS Lett., 2002, 514, 315–322 CrossRef CAS PubMed.
- H. Ji, Y. Liu and F. He,
et al., LC-MS based urinary metabolomics study of the intervention effect of aloe-emodin on hyperlipidemia rats, J. Pharm. Biomed. Anal., 2018, 156, 104 CrossRef CAS PubMed.
- Q. Wu, H. Zhang, X. Dong, X. F. Chen, Z. Y. Zhu, Z. Y. Hong and Y. F. Chai, UPLC-Q-TOF/MS based metabolomic profiling of serum and urine of hyperlipidemic rats induced by high fat diet, J. Pharm. Anal., 2014, 4(6), 360–367 CrossRef CAS PubMed.
- X. Wang, H. Lv and A. Zhang,
et al., Metabolite profiling and pathway analysis of acute hepatitis rats by UPLC-ESI MS combined with pattern recognition methods, Liver Int., 2014, 34, 759–770 CrossRef CAS PubMed.
- C. Pavoine and F. Pecker, Sphingomyelinases: their regulation and roles in cardiovascular pathophysiology, Cardiovasc. Res., 2009, 82, 175–183 CrossRef CAS PubMed.
- R. P. Rao, C. Yuan and J. C. Allegood,
et al., Ceramide transfer protein function is essential for normal oxidative stress response and lifespan, Proc. Natl. Acad. Sci. U. S. A., 2007, 104, 11364–11369 CrossRef CAS PubMed.
- N. P. Rotstein, G. E. Miranda and C. E. Abrahan,
et al., Regulating survival and development in the retina: key roles for simple sphingolipids, J. Lipid Res., 2010, 51, 1247–1262 CrossRef CAS PubMed.
- M. Regnier, P. Arnaud and H. Guillou, Nicolas Loiseau Sphingolipid metabolism in non-alcoholic fatty liver diseases, Biochimie, 2019, 159, 9–22 CrossRef CAS PubMed.
|
This journal is © The Royal Society of Chemistry 2020 |