DOI:
10.1039/C9RA10813K
(Paper)
RSC Adv., 2020,
10, 6482-6490
Construction of a simple crosslinking system and its influence on the poling efficiency and oriental stability of organic electro-optic materials
Received
23rd December 2019
, Accepted 22nd January 2020
First published on 11th February 2020
Abstract
A new fluorinated polycarbonate was synthesized by solution polymerization of bisphenol AF, anthracen-9-ylmethyl-4,4-bis(4-hydroxyphenyl)pentanoate with triphosgene. This new polycarbonate was used as a host polymer. A traditional chromophore with a D–π–A structure was modified by bisphenol A acrylate affording chromophore A. The large modified group can not only effectively avoid the dipole interaction between chromophores, but also endow the chromophores with the properties of attaching and crosslinking with a host polymer through in situ Diels–Alder “click chemistry” reactions. Such properties can improve both the poling efficiency and the thermal stability of organic electro-optic (EO) polymers. The thermodynamic properties of the crosslinking system showed that the glass transition temperature rose with the increase of the chromophore content. The EO polymer showed a large EO coefficient of about 78.9 pm V−1 at a wavelength of 1310 nm and an excellent long-term stability of about 89% with respect to its initial value and it can be kept after 300 h of heating at 80 °C.
1. Introduction
With the rapid development of information technology and information demands in our lives and production, a large amount of high bandwidth optical communication devices, such as EO modulators, all-optical switches, optical phase shifters and tunable lasers have been designed and prepared in recent decades. As the material foundation of high bandwidth EO conversion devices, organic second order nonlinear optical (NLO) materials have also attracted great attention.1–5 Thousands of NLO materials have been reported. Some of them show large EO coefficients and some of them show good long-term stability. However, few of them have been used in commercial applications. The main reason is that the comprehensive performance of organic NLO materials is not good. Materials with both high EO coefficients and good long-term stability are very few. Normally, for practical commercial applications, the EO coefficients of organic NLO materials should reach 60 pm V−1@1310 nm,6,7 and the stability of EO activity should be kept at 80 °C for more than 200 hours.1,8–11
The host–guest doping method is the most commonly used method to prepare organic NLO materials. The guest NLO chromophores have the greatest movement activity in this system and large EO coefficients are mainly measured in this system. But the movement activity of the chromophore is a double-edged sword which can improve the poling efficiency and reduce the long-term stability of the organic NLO materials. Clicking the guest chromophores with host polymers with chemical bonds can effectively improve the long-term stability of organic NLO materials. However, due to the movement activity of the chromophore being reduced, the best orientation of the chromophore is difficult to achieve.12–14 “Click-chemistry”, based on Diels–Alder reactions, has emerged as a powerful strategy to construct NLO polymers.15–19 Synthetic advantages of this approach include excellent conversion efficiency, mild reaction conditions, and compatibility with the poling process.20 Thus Diels–Alder reactions have been selected as one of the most efficient “click chemistry” reactions to construct high-performance NLO polymers.
In this work, the new fluorinate polycarbonate P was synthesized from the monomer bisphenol AF and anthracen-9-ylmethyl-4,4-bis(4-hydroxyphenyl)pentanoate with triphosgene,21–23 which can afford the “D” part of the “Diels–Alder” reaction. The chromophore with the julolidinyl-based group as the electron donor and 2-dicyanomethylene-3-cyano-4-methyl-5-phenyl-5-trifluoromethyl-2,5-dihydrofuran (CF3-Ph-TCF) as the acceptor was also synthesized as the crosslinking agent. After being modified by acrylic ester, such a chromophore affords the “A” part of the “Diels–Alder” reaction. Polymers P1–P3 were obtained by doping chromophores of different concentrations into polycarbonate. The in situ Diels–Alder reaction between anthracene and acryloyl functional groups can form a crosslinked network for hindering the movement of chromophores. Polymers P1–P3 showed relatively high Tg, excellent thermal stability and large EO coefficients.
2. Experimental
2.1 Materials and instruments
All the chemicals were purchased from Aldrich or Beijing Lanyi Chemical co. ltd and were used as received unless otherwise specified. All the organic solvents were distilled before use. Chromatography on silica gel was carried out on Kieselgel (200–300 mesh). 1H NMR spectra were determined on an Advance Bruker 400 M (400 MHz) NMR spectrometer (tetramethylsilane was the internal reference). UV-Vis spectra were performed on a Cary 5000 photo spectrometer. Thermogravimetric analysis (TGA) and differential scanning calorimetry (DSC) were determined by a TA-instrument Q50 and Q10 with a heating rate of 10 °C min−1 under nitrogen respectively. The refractive index was measured by an SPA-4000 Waveguide prism coupling instrument under the wavelength of 1310 nm.
2.2 Synthesis of compound a
To a solution of 4,4-bis(4-hydroxyphenyl)pentanoic acid (2.86 g, 10.0 mmol) in N,N-dimethylformamide (DMF) (25 mL) was added KHCO3 (1.25 g, 12.5 mmol). After stirring for 30 min at 80 °C, 9-(chloromethyl)anthracene (2.72 g, 12.0 mmol) was added dropwise. The reaction mixture was stirred at 80 °C for another 5 h before being cooled to room temperature and then poured into distilled water (250 mL).24 Ethyl acetate (3 × 50 mL) was used to extract the product. The extracts were combined, washed with saturated NaCl solution, and dried over anhydrous magnesium sulfate. After removal of the solvents, the product was purified by column chromatography (silica gel, hexane/ethyl acetate: 1/1 v/v) and obtained as a syrup (4.35 g, yield: 84%). HRMS (ESI, m/z): calcd for C32H28O4, 476.20 [M + Na]+; found, 499.1853. 1H NMR (400 MHz, acetone) δ 8.62 (s, 1H), 8.40 (d, J = 8.9 Hz, 2H), 8.10 (d, J = 7.5 Hz, 4H), 7.65–7.58 (m, 2H), 7.56–7.47 (m, 2H), 6.98 (d, J = 8.2 Hz, 4H), 6.70 (d, J = 8.1 Hz, 4H), 6.14 (s, 2H), 2.41–2.33 (m, 2H), 2.13–2.08 (m, 2H), 1.47 (s, 3H). 13C NMR (101 MHz, (CD3)2CO) δ 174.14, 156.05, 140.87, 132.35, 131.82, 129.86, 129.79, 129.76, 128.95, 127.78, 127.40, 126.00, 125.00, 115.55, 59.08, 45.03, 37.57, 30.86, 28.01.
2.3 Synthesis of polycarbonate P
To a mixture of compound a (1.14 g, 2.5 mmol), pyridine (1 mL) and bisphenol AF (1.68 g, 5 mmol) in 30 mL 1,2-dichloroethane were added. After stirring for 15 min at rt, the mixture was cooled at 0 °C and a solution of triphosgene (0.78 g) in dry tetrahydrofuran (THF) (5 mL) was added dropwise. Subsequently, the mixture was stirred overnight at rt. The solution was slowly dropped into methanol (400 mL), filtered, washed with methanol, and dried in a vacuum oven for 24 h at 50 °C. The polycarbonate P was observed (3.52 g, yield: 90%). 1H NMR (400 MHz, CDCl3) δ 8.41 (s, 1H), 8.22 (d, J = 8.8 Hz, 2H), 7.93 (d, J = 8.1 Hz, 2H), 7.48 (t, J = 7.3 Hz, 2H), 7.38 (t, J = 8.8 Hz, 10H), 7.22 (dd, J = 22.2, 12.8 Hz, 8H), 7.14–7.00 (m, 8H), 6.04 (s, 2H), 2.39 (s, 2H), 2.05 (s, 2H), 1.48 (s, 3H).
2.4 Synthesis of compound 2
Compound 1 (0.44 g, 1.2 mmol) and compound 1a (0.667 g, 1.3 mmol) were dissolved in dichloromethane, and DCC and DPTS were added. After 5 h, the ethanol was removed by a rotary evaporator, the crude production was purified with flash chromatography. 0.97 g of a slightly yellow solid was obtained (yield 95%). HRMS (ESI, m/z): calcd for C52H79NO6Si2: 869.54 [M]− found, 870.5504. 1H NMR (400 MHz, CDCl3) δ 9.93 (s, 1H), 7.58 (s, 1H), 7.02 (d, J = 8.2 Hz, 4H), 6.72 (d, J = 8.1 Hz, 4H), 4.02 (t, J = 6.6 Hz, 2H), 3.95 (t, J = 6.7 Hz, 2H), 3.29 (t, J = 6.0 Hz, 2H), 3.25–3.20 (m, 2H), 2.41–2.34 (m, 2H), 2.12–2.05 (m, 2H), 1.92–1.83 (m, 2H), 1.71 (dd, J = 12.3, 6.6 Hz, 4H), 1.66–1.61 (m, 2H), 1.55 (s, 3H), 1.53–1.47 (m, 2H), 1.42 (s, 8H), 1.26 (s, 6H), 0.97 (s, 18H), 0.18 (s, 12H).13C NMR (101 MHz, CDCl3) δ 191.33, 177.69, 165.55, 157.01, 151.76, 145.17, 129.71, 129.16, 124.33, 122.89, 120.85, 82.13, 67.93, 51.08, 50.43, 48.16, 42.98, 40.25, 39.29, 36.05, 35.65, 33.93, 33.84, 33.55, 33.34, 32.14, 31.40, 29.52, 29.25, 21.73, −0.82.
2.5 Synthesis of compound 3
Compound 2 (0.87 g, 1 mmol) and CF3-Ph-TCF (0.34 g, 1.1 mmol) were dissolved in ethanol, and then heated under reflux for 1.5 h. The ethanol was removed by rotary evaporator, and the crude production was purified using flash chromatography. 0.97 g of a dark blue solid was obtained (yield 83%). HRMS (ESI, m/z): calcd for C68H85F3N4O6Si2: 1166.60, [M + 23]+ found, 1189.5834. 1H NMR (400 MHz, CDCl3) δ 7.80 (s, 1H), 7.46 (d, J = 7.7 Hz, 2H), 7.40 (d, J = 6.7 Hz, 3H), 7.27 (s, 1H), 6.95 (d, J = 8.4 Hz, 4H), 6.65 (d, J = 8.4 Hz, 5H), 3.94 (t, J = 6.7 Hz, 2H), 3.62–3.43 (m, 2H), 3.39 (t, J = 5.9 Hz, 2H), 3.30 (t, J = 5.3 Hz, 2H), 2.35–2.27 (m, 2H), 2.07–1.98 (m, 2H), 1.66–1.47 (m, 11H), 1.23 (dd, J = 35.3, 8.4 Hz, 16H), 0.89 (s, 18H), 0.11 (s, 12H).13C NMR (101 MHz, CDCl3) δ 176.39, 174.17, 162.16, 161.55, 153.55, 151.22, 146.19, 141.67, 131.16, 130.96, 129.54, 129.19, 128.28, 127.14, 123.07, 119.42, 117.22, 112.58, 112.41, 112.12, 107.46, 64.38, 48.46, 47.72, 44.67, 38.58, 36.79, 34.75, 32.46, 32.05, 30.44, 29.63, 29.37, 29.29, 29.07, 28.94, 27.92, 25.91, 25.77, 25.34, 18.24, −4.30.
2.6 Synthesis of compound 4
Compound 3 (0.60 g, 5.1 mmol) was dissolved in 30 mL methanol, and 10 mL hydrochloric acid (10%) was added and kept for 3 h. The methanol was removed by rotary evaporator, and the remaining part was washed by saturated brine and extracted by chloroform. The crude production was purified by flash chromatography. 0.457 g of a dark blue solid was obtained (yield 95%).
HRMS (ESI, m/z): calcd for C56H57F3N4O6, 938.42 [M]− found, 937.41156. 1H NMR (400 MHz, CDCl3) δ 7.88 (d, J = 14.9 Hz, 1H), 7.55–7.43 (m, 5H), 7.35 (s, 1H), 7.00 (d, J = 8.5 Hz, 4H), 6.74 (t, J = 7.1 Hz, 5H), 4.02 (t, J = 6.4 Hz, 2H), 3.61 (dq, J = 23.1, 7.9 Hz, 2H), 3.47 (t, J = 5.8 Hz, 2H), 3.38 (t, 2H), 2.43–2.35 (m, 2H), 2.21–2.09 (m, 4H), 1.76–1.56 (m, 8H), 1.53 (s, 3H), 1.34 (d, J = 8.7 Hz, 7H), 1.26 (d, J = 6.5 Hz, 7H). 13C NMR (101 MHz, CDCl3) δ 176.55, 174.67, 162.12, 161.44, 153.95, 151.10, 146.14, 140.78, 140.74, 131.15, 130.80, 129.52, 128.34, 127.05, 125.58, 123.44, 120.98, 117.67, 114.94, 112.68, 112.36, 112.01, 107.69, 95.67, 95.35, 77.93, 64.54, 48.55, 47.82, 44.50, 38.41, 36.74, 34.63, 32.42, 32.01, 30.93, 30.46, 29.55, 29.32, 29.26, 28.96, 28.87, 28.47, 27.83, 25.89, 25.30.
2.7 Synthesis of chromophore A
Compound 4 (0.20 g, 2.1 mmol) and a certain amount of Et3N were dissolved in 30 mL of dry acetone, and acryl chloride was added dropwise into the solution at 0 °C. After 2 h, acetone was removed by rotary evaporator, and the rest was purified by flash chromatography. 0.214 g of a dark blue solid was obtained (yield 96%). HRMS (ESI, m/z): calcd for C62H61F3N4O8: 1046.44 [M]− found: 1045.4368. 1H NMR (400 MHz, CDCl3) δ 7.86 (d, J = 12.5 Hz, 1H), 7.51 (dd, J = 24.5, 6.8 Hz, 5H), 7.34 (s, 1H), 7.22 (d, J = 8.4 Hz, 4H), 7.05 (d, J = 8.2 Hz, 4H), 6.74 (d, J = 15.0 Hz, 1H), 6.59 (d, J = 17.3 Hz, 2H), 6.31 (dd, J = 17.3, 10.4 Hz, 2H), 6.00 (d, J = 10.4 Hz, 2H), 4.03 (t, J = 6.6 Hz, 2H), 3.70–3.50 (m, 2H), 3.43 (dd, J = 24.3, 18.4 Hz, 4H), 2.50–2.42 (m, 2H), 2.18–2.10 (m, 2H), 1.75–1.58 (m, 11H), 1.43 (s, 2H), 1.35 (d, J = 10.7 Hz, 6H), 1.26 (d, J = 6.0 Hz, 6H), 0.86 (d, J = 7.3 Hz, 2H).13C NMR (101 MHz, CDCl3) δ 176.24, 173.74, 164.51, 162.01, 161.70, 150.90, 148.73, 146.17, 145.93, 132.51, 131.09, 130.86, 129.48, 128.97, 128.33, 128.00, 127.11, 122.94, 121.08, 117.07, 112.38, 112.23, 111.92, 107.52, 64.45, 54.13, 48.33, 47.61, 45.33, 38.56, 36.47, 34.75, 32.39, 31.99, 30.19, 29.54, 29.34, 29.26, 29.03, 28.90, 28.52, 27.80, 26.93, 25.84, 25.27, 22.66, 14.12, 11.43.
2.8 Preparation of EO polymer thin films P1–P3
Chromophore A and polycarbonate P were dissolved in dibromomethane with a concentration of about 10 wt%, and the solution was stirred for 12 h. When all the solids were dissolved, the solution was filtered by 0.22 μm PTFE-syringe filters and spin-coated onto the indium tin oxide (ITO) glass substrates with a rotating speed of about 500–600 rpm. The thickness of the yielding optical quality thin films was 1.5–2.5 μm. P1 was composed of chromophore A (15 mg) and polycarbonate P (100 mg). P2 was composed of chromophore A (25 mg) and polycarbonate P (100 mg). P3 was composed of chromophore A (35 mg) and polycarbonate P (100 mg).
2.9 Poling and crossing processes of the EO films
The EO films were heated at a temperature of 130 °C for 30 min, at the first stage. Most of the chromophores were cross-linked at this stage. The EO films were kept at (Tg + 5) °C for 10 min under an electric field voltage of 10.5 kV. Then, the temperature was reduced to 80 °C for the crosslinking process for 30 min. After the crosslinking process finished, the temperature was reduced to room temperature and the electric field was removed.
3. Results and discussion
3.1 Synthesis of chromophore A and polymer P
The synthetic routes for chromophore A are shown in Scheme 1. Compound 1, compound a and CF3-Ph-TCF have been reported before.26–28 Firstly, the julolidine electron donor reacted with diphenolic acid with the phenolic hydroxyl groups protected by tert-butyldimethylsilyl (TBS) groups. Then the CF3-Ph-TCF acceptor was introduced to the donor groups by a Knoevenagel condensation reaction affording the D–π–A conjugated structure. Then the protective group of TBS was removed by hydrochloric acid affording the active phenolic hydroxyl groups. Finally, through the esterification reaction between the phenolic hydroxyl groups of the chromophore and acryl chloride, chromophore A with Diels–Alder reaction active groups was synthesized. The total yield of chromophore A is about 72%. This synthesized route was very successful, due to the high yield and mild reactive conditions. The key step in this synthetic route is the last reaction. The weak change of such reaction conditions can destroy the D–π–A conjugated system and influence the yield of chromophore A. Comparing with the traditional side chain polycarbonate NLO materials,12,25 the strategy used in this manuscript avoids the damage of conjugated chromophores caused by high temperature during polycarbonate synthesis or the coupling process, so that the chromophore content in the polymer can be more accurately controlled.
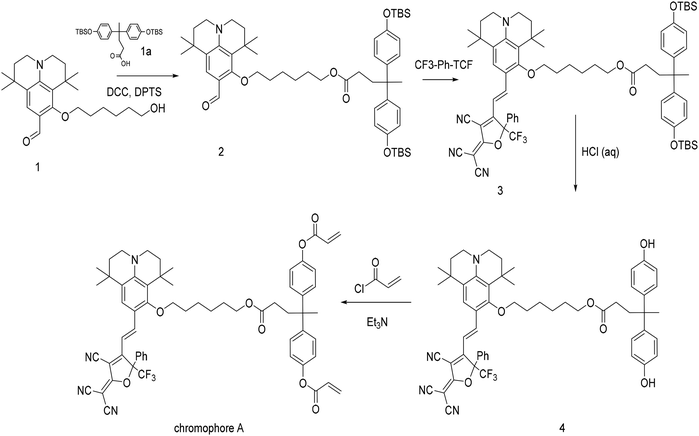 |
| Scheme 1 Synthetic routes for chromophore A. | |
The structure and synthetic routes of the polycarbonate P are shown in Scheme 2. The esterification reaction between 4,4-bis(4-hydroxyphenyl)pentanoic acid and 9-(chloromethyl)anthracene affords the monomer compound a. Because of the competition between the phenol hydroxyl and alcohol hydroxyl groups, the control of pH value and dropping rate of 9-(chloromethyl)anthracene have a great influence on the yield of compound a. Polycarbonate P was prepared by the esterification reaction between monomer compound a, bisphenol AF and triphosgene at low temperature.
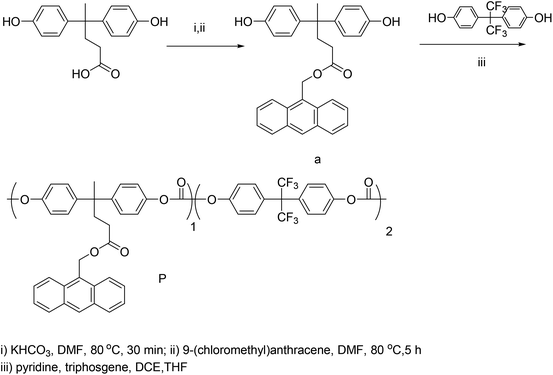 |
| Scheme 2 Synthetic routes for polycarbonate P. | |
The chemical structure of polymer P was characterized by 1H NMR spectrum. The feed ratio of compound a and bisphenol AF is about 1
:
2. From the calculation of H atoms from the 1H NMR spectrum (S6), the ratio of compound a and bisphenol AF in polycarbonate P is consistent with the feed ratio. The molecular weight and molecular weight distribution of polymer P were measured by gel permeation chromatography (GPC) using PS as the standards. The molecular weight and molecular weight distribution were about 132
103 and 1.68, respectively. Though the molecular weight is not very high, it is higher than other NLO polycarbonate materials with chromophores as side chains.
3.2 Photophysical properties of chromophore 3, 4 and A
The UV-Vis absorption spectra of chromophore A and its precursors compound 3 and compound 4 in six solvents are shown in Fig. 1 and the corresponding data is listed in Table 1. The maximum absorption wavelength (λmax) of compound 3, compound 4 and chromophore A is obtained in acetone, and the absorption of chromophore A is the minimum in dioxane, where they have a similar D–π–A structure and also show a similar absorption curve. The maximum absorption wavelengths (λmax) are 671 nm, 672 nm and 671 nm, respectively. The maximum red shifts were 27 nm, 26 nm and 25 nm, respectively. There was no significant change in the maximum absorption, which indicated that the modified groups at the end of the donor had no significant effect on the conjugated structure. The position of the maximum absorption peak of the chromophore is mainly determined by the π–π* orbit of the conjugated system. The second-order nonlinear optical properties of the dipole molecules have a great relationship with λmax. The chromophore with a larger λmax will have a lower charge transfer energy, so it also has a higher first-order hyperpolarizability and a higher second-order nonlinear optical response. The chromophore containing strong receptor CF3-PH-TCF will be more polarizable, more sensitive to the external electric field, and more conducive to the orderly orientation of molecules under the external electric field. At the same time, we found that the solubility of the three chromophores in various solvents is very good. At the same time, we can see that all the structures have slight shoulder peaks on the left side, indicating that the molecules are in the H-aggregation state, but all the shoulder peaks are extremely weak. Even in toluene, from the side, it is also shown that such chromophores can efficient shield their dipole–dipole interaction by their own modified structures.
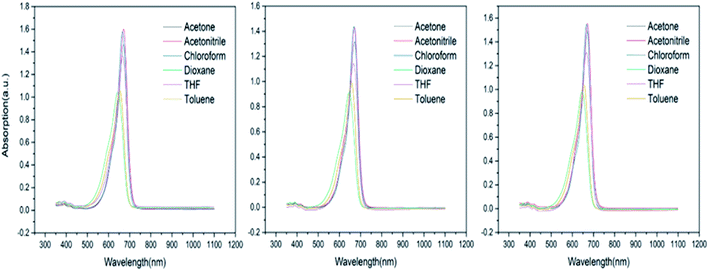 |
| Fig. 1 UV-visible spectroscopy of compound 3 (left), 4 (middle) and A (right). | |
Table 1 Maximum absorption peaks of the chromophore in different solvents
Chromophore |
Acetone (nm) |
Acetonitrile (nm) |
Chloroform (nm) |
Dioxane (nm) |
THF (nm) |
Toluene (nm) |
3 |
671 |
671 |
666 |
644 |
666 |
652 |
4 |
672 |
672 |
669 |
646 |
667 |
657 |
A |
671 |
671 |
646 |
646 |
667 |
654 |
3.3 Thermal stability of chromophore A and NLO polymers
The thermal stability of EO polymers was investigated by DSC. All the Tgs appear at nearly 150 °C before poling and crosslinking processes. Unlike the EO polycarbonates reported previously,12,25 the Tg values changed slightly with the variation of chromophores content. The EO polymer films P1–P3 have a high glass transition temperature Tg (>145 °C) and far more than P (Tg = 136 °C) which were high enough for the progress of the EO device operation, as shown in Fig. 2 and Table 2. P2 and P3 with more chromophore A have a higher Tg than P1 due to the rigid structure of the chromophore. After the poling and crosslinking process, no obvious Tg can be found for polymer 1, 2 and 3. The crosslinking process can be depicted as follows.
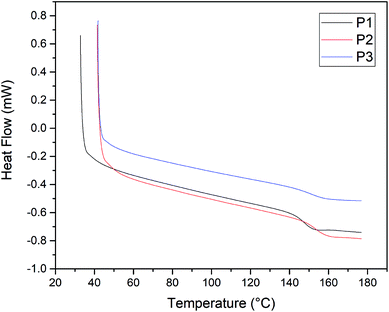 |
| Fig. 2 DSC curves of the EO polymer films P1–P3. | |
Table 2 Characterization and properties of EO polycarbonatesa
Polymer |
Tg (°C) |
Chromophore density (wt%) |
Number density (1020 molecules cm−3) |
Refractive index |
r33 (pm V−1) |
n3r33 (pm V−1) |
Film thickness (μm) |
Temporal stability (%) |
Tg measured at a heating rate of 10 °C min−1 under nitrogen atmosphere by DSC. r33 values were measured by simple reflection techniques at a wavelength of 1310 nm. Index of refraction measurement at 1310 nm for the unpoled film. |
P1 |
148 |
13 |
0.75 |
1.67 |
33.1 |
154.2 |
1.9 |
85 |
P2 |
153 |
20 |
1.15 |
1.68 |
55.5 |
263.2 |
2.0 |
86 |
P3 |
150 |
26 |
1.50 |
1.69 |
78.9 |
380.8 |
2.0 |
89 |
Compared to our previous work, it was only necessary to dope the chromophore with the polymer without adding additional crosslinkers. The chromophore can be attached to the polymer while the two polymer chains can be joined together to form a crosslinked network as a cross-linking agent. The EO film has a clear glass transition temperature compared to our previous work. This work is more repeatable and reliable. The heating cross-linking is integrated with the polarization process. The cross-linking process is shown in Scheme 3. It is an advantage of the cross-linking system, which can support high concentrations of chromophores while maintaining enough network structures. Such a result indicates that the crosslinking degree is very high. Such a high crosslinking degree can confirm the long-term stability of this EO polymer effectively. The unique UV spectrum of anthracene is used to study the reaction degree of the Diels–Alder reaction and the crosslinking degree of materials, which is shown in Fig. 3. Obviously, after the crosslinking process, the unique UV spectrum of anthracene disappeared completely. The same phenomenon was also observed for P1 and P2.
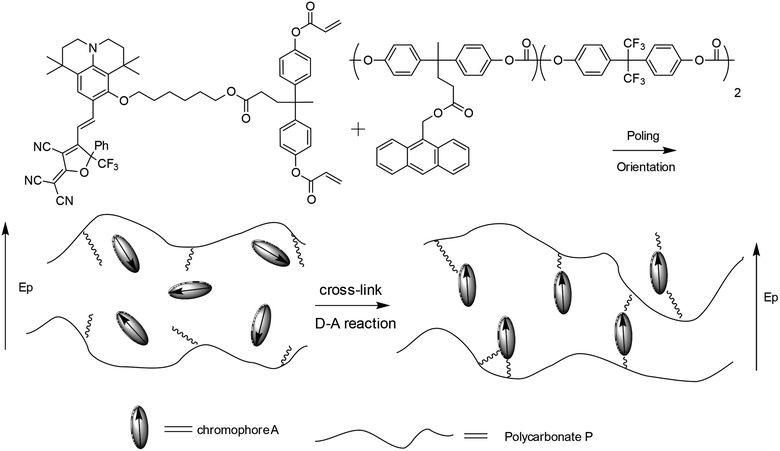 |
| Scheme 3 The crosslinking process of the EO polymers. | |
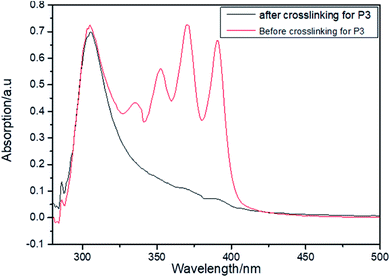 |
| Fig. 3 UV-Vis absorption spectra of polymer P3 before and after crosslinking. | |
Due to the introduction of 4,4'-(hexafluoroisopropylidene)diphenol (BPAF), the polycarbonate was soluble in common polar organic solvents such as DMF, dimethyl sulfoxide (DMSO), THF, halogenated solvents and cyclopentanone. The polycarbonate can also be prepared into homogeneous, smooth, and high-quality films. The preparation process of the EO films can be divided into several steps using the method proposed previously.19 After the synthesis of NLO chromophore A and polycarbonate P, the two things were dissolved in dibromomethane with an appropriate ratio, and the solution was filtered and spin-coated on ITO glass. The EO films were subjected to poling and cross linking under a direct current field and special temperatures during the poling process. As shown in Fig. 4, all of the EO polymers showed good surface features and no phase separation was found from the AFM images.
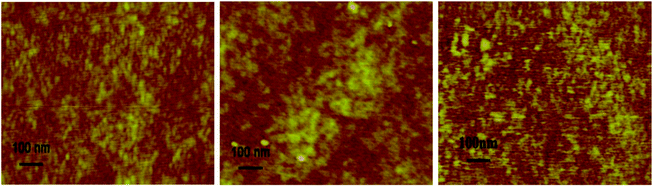 |
| Fig. 4 AFM images of the EO films prepared by polymer P1 (left), P2 (middle) and P3 (right). | |
3.4 Electro-optic performance and long-term stability of the NLO polymers
To evaluate the EO properties of the EO polymers, poled thin films were prepared. The r33 were measured by simple reflection method.29 The r33 value is calculated via the following equation:
Here, r33 is the EO coefficient of the poled polymer, λ is the optical wavelength, θ is the incident angle, Ic is the output beam intensity, Im is the amplitude of the modulation, Vm is the modulating voltage, and n is the refractive index of the polymer films.
The r33 values of polymer P1, P2 and P3 under the optimum poling conditions are shown in Table 2. Polymer P1 with a chromophore loading density of about 13 wt% obtained the desirable r33 value of 33.1 pm V−1. When the chromophore loading density increased to 20 wt%, the r33 values of polymer P2 reached 55.5 pm V−1. As the chromophore loading density increased again to 25 wt%, the r33 values of polymer P3 reached 78.9 pm V−1. The strong growth momentum of r33 values is still not weakened. This result is much better than the sidechain polycarbonates systems we reported previously.23 When the concentration was increased to 26 wt%, the r33 values only changed slightly and reached the saturated value in the previous sidechain polycarbonates systems. The maximum value of polymer P3 reached 78.9 pm V−1, and this result is much better than the doped guest–host systems (36 pm V−1 at 40 wt%) and sidechain polycarbonates systems (55 pm V−1 at 26 wt%). The enhanced EO coefficients indicated that the chromophores in polymer 3 can be effectively oriented, which is probably due to the fact that the large isolated groups of chromophores A have a good isolated effect and the inter-chromophore dipole–dipole interactions are effectively suppressed below the saturated loading density. Further, the good mobility of chromophore A under the action of an external electric field is another reason for the improvement of the EO coefficient. Comparing with the sidechain polycarbonate systems, the chromophore saturated loading density of polymer 3 is higher than the sidechain polycarbonates systems. It might reach the chromophore saturated loading density of the host–guest doped system. The large improvement of the EO coefficient of polymer P3 compared to the host–guest doped system indicated that the inevitable aggregation of dipolar chromophores in an antiparallel manner in the host–guest doped system was effectively avoided. n3r33 is an important metric for the design and preparation of organic EO devices such as modulators and optical switches. It is inversely proportional to the driving voltage (VπL) of such devices. Thus, organic NLO materials with a large refractive index and a high chromophore number density are favored. Comparing the traditional polycarbonate materials, the novel polycarbonate prepared in this paper showed a higher refractive index (1.67–1.69). Polymer P3 showed the highest n3r33 figure-of-merit around 380 pm V−1 (1310 nm).
The long-term stability of the poled polymer was investigated and the dipole reorientation of the poled polymer films was observed by measuring the EO coefficient (r33(t)/r33 (0)) as a function of time at 80 °C (above the device processing temperature) as shown in Fig. 5. The poled films of P1–P3 all displayed well long-term stability. After annealing at 80 °C for 300 h, P1–P3 retained 85%, 86%, and 89% of the initial r33 values, respectively. The fast decay of the EO coefficient at the beginning of thermal treatment may result from the recovery of the bond angle and the bond length of the oriented chromophore. Obviously, as the chromophore concentration increases, the cross-linking of the system also increases, making the EO film more stable. The improvement of such a performance can be attributed to the improvement of crosslinking degree with the increasing of chromophore loading density. These results indicated that the design strategy for NLO chromophores and EO polymers provides an effective method for the preparation of excellent EO materials.
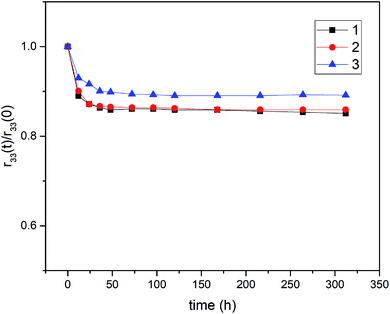 |
| Fig. 5 Long-term stability of the EO polymer films at 80 °C for 300 h. | |
4. Conclusions
In this work, a new type of fluorinated NLO co-polycarbonate was prepared. The BPAF group was used to improve its solubility. A post-functional methodology was used to control the chromophores' loading density exactly and easily. By the Diels–Alder reaction, a series of polycarbonates containing different contents of chromophore were obtained. These chromophores containing co-polycarbonates P1–P3 not only maintained the excellent thermal stability and optical properties of traditional polycarbonates, but also improved the solubility and film-forming capability, and high r33 values were achieved from these polycarbonates. Polycarbonate P3 (chromophore 26 wt%) presented a relatively high r33 (78.9 pm V−1) at 1310 nm. 89% of the r33 value was retained in polycarbonate P3 after being heated at 80 °C for 300 hours.
Conflicts of interest
There are no conflicts to declare.
Acknowledgements
We are grateful to the National Natural Science Foundation of China (No. 51503215) and the Young talent program of Chinese Academy of Agricultural Sciences for financial support.
References
- M. Stähelin, C. A. Walsh, D. M. Burland, R. D. Miller, R. J. Twieg and W. Volksen, Orientational decay in poled second-order nonlinear optical guest–host polymers: temperature dependence and effects of poling geometry, J. Appl. Phys., 1993, 73(12), 8471–8479 CrossRef.
- Y. Shi, C. Zhang, H. Zhang, J. H. Bechtel, L. R. Dalton, B. H. Robinson and W. H. Steier, Low (sub–1-volt) halfwave voltage polymeric electro-optic modulators achieved by controlling chromophore shape, Science, 2000, 288(5463), 119–122 CrossRef CAS PubMed.
- D. M. Burland, R. D. Miller and C. A. Walsh, Second-order nonlinearity in poled-polymer systems, Chem. Rev., 1994, 94(1), 31–75 CrossRef CAS.
- L. R. Dalton, W. H. Steier, B. H. Robinson, C. Zhang, A. Ren, S. Garner, A. T. Chen, T. Londergan, L. Irwin, B. Carlson, L. Fifield, G. Phelan, C. Kincaid, J. Amend and A. Jen, From molecules to opto-chips: organic electro-optic materials, J. Mater. Chem., 1999, 9(9), 1905–1920 RSC.
- J. D. Luo, X. H. Zhou and A. K. Y. Jen, Rational molecular design and supramolecular assembly of highly efficient organic electro-optic materials, J. Mater. Chem., 2009, 19(40), 7410–7424 RSC.
- Y. Enami, D. Mathine, C. T. DeRose, R. A. Norwood, J. Luo, A. K. Y. Jen and N. Peyghambarian, Hybrid cross-linkable polymer/sol–gel waveguide modulators with 0.65 V half wave voltage at 1550 nm, Appl. Phys. Lett., 2007, 91(9), 093505 CrossRef.
- R. Dinu, J. Dan, Y. Guomin, C. Baoquan, H. Diyun, C. Hui, A. Barklund, E. Miller, C. Wei and J. Vemagiri, Environmental Stress Testing of Electro–Optic Polymer Modulators, J. Lightwave Technol., 2009, 27(11), 1527–1532 CAS.
- R. J. Michalak, Y. H. Kuo, F. D. Nash, A. Szep, J. R. Caffey, P. M. Payson, F. Haas, B. F. McKeon, P. R. Cook, G. A. Brost, J. D. Luo, A. K. Y. Jen, L. R. Dalton and W. H. Steier, High-speed AJL8/APC polymer modulator, IEEE Photonics Technol. Lett., 2006, 18(9–12), 1207–1209 CAS.
- J. W. Kang, T. D. Kim, J. Luo, M. Haller and A. K. Y. Jen, Very large electro-optic coefficients from in situ generated side-chain nonlinear optical polymers, Appl. Phys. Lett., 2005, 87(7), 071109 CrossRef.
- S. J. Benight, D. H. Bale, B. C. Olbricht and L. R. Dalton, Organic electro-optics: understanding material structure/function relationships and device fabrication issues, J. Mater. Chem., 2009, 19(40), 7466–7475 RSC.
- H. Huang, J. Liu, Z. Zhen, L. Qiu, X. Liu, G. Lakshminarayana, S. Tkaczyk and I. V. Kityk, Synthesis of tricyanofuran chromophore with flexible rigid isolated group and its application as nonlinear optical materials, Mater. Lett., 2012, 75, 233–235 CrossRef CAS.
- G. Deng, S. Bo, T. Zhou, H. Huang, J. Wu, J. Liu, X. Liu, Z. Zhen and L. Qiu, Facile synthesis and electro-optic activities of new polycarbonates containing tricyanofuran-based nonlinear optical chromophores, J. Polym. Sci., Part A: Polym. Chem., 2013, 51(13), 2841–2849 CrossRef CAS.
- C. Peng, J. Wu, J. Liu, L. Qiu, X. Liu, S. Bo and Z. Zhen, Synthesis and optical properties of new fluorinated second-order nonlinear optical copolymers: an attempt toward the balance
between solubility and long-term alignment stability, Polym. Chem., 2013, 4(9), 2703 RSC.
- J. Liu, P. Si, X. Liu and Z. Zhen, Copper-catalyzed Huisgen cycloaddition reactions used to incorporate NLO chromophores into high Tg side-chain polymers for electro-optics, Opt. Mater., 2015, 47, 256–262 CrossRef CAS.
- Z. W. Shi, J. D. Luo, S. Huang, Y. J. Cheng, T. D. Kim, B. M. Polishak, X. H. Zhou, Y. Q. Tian, S. H. Jang, D. B. Knorr, R. M. Overney, T. R. Younkin and A. K. Y. Jen, Controlled Diels–Alder Reactions Used To Incorporate Highly Efficient Polyenic Chromophores into Maleimide-Containing Side-Chain Polymers for Electro-Optics, Macromolecules, 2009, 42(7), 2438–2445 CrossRef CAS.
- T.-D. Kim, J. Luo, Y. Tian, J.-W. Ka, N. M. Tucker, M. Haller, J.-W. Kang and A. K. Y. Jen, Diels-alder “click chemistry” for highly efficient electro-optic polymers, Macromolecules, 2006, 39, 1676–1680 CrossRef CAS.
- Z. Shi, S. Hau, J. Luo, T. D. Kim, N. M. Tucker, J. W. Ka, H. Sun, A. Pyajt, L. Dalton, A. Chen and A. K. Y. Jen, Highly Efficient Diels–Alder Crosslinkable Electro-Optic Dendrimers for Electric-Field Sensors, Adv. Funct. Mater., 2007, 17(14), 2557–2563 CrossRef CAS.
- T. D. Kim, J. Luo, J. W. Ka, S. Hau, Y. Tian, Z. Shi, N. M. Tucker, S. H. Jang, J. W. Kang and A. K. Y. Jen, Ultralarge and Thermally Stable Electro-optic Activities from Diels–Alder Crosslinkable Polymers Containing Binary Chromophore Systems, Adv. Mater., 2006, 18(22), 3038–3042 CrossRef CAS.
- W. Gao, G. Qin, J. Liu, A. A. Fedorchuk, K. Ozga and I. V. Kityk, Design and preparation of novel Diels–Alder crosslinking polymer and its application in NLO materials, J. Mater. Sci.: Mater. Electron., 2017, 28(12), 8480–8486 CrossRef CAS.
- P. Wang, H. Zhang, G. Zhang, Y. Huang, J. Liu and S. Bo, Greatly improved performance for NLO chromophore with 4,4′-bis(diethylamino)benzophenone as donor by introducing stronger acceptor, Mater. Lett., 2018, 226, 38–42 CrossRef CAS.
- M. Faccini, M. Balakrishnan, R. Torosantucci, A. Driessen, D. N. Reinhoudt and W. Verboom, Facile Attachment of Nonlinear Optical Chromophores to Polycarbonates, Macromolecules, 2008, 41(22), 8320–8323 CrossRef CAS.
- M. Faccini, M. Balakrishnan, M. B. J. Diemeer, R. Torosantucci, A. Driessen, D. N. Reinhoudt and W. Verboom, Photostable nonlinear optical polycarbonates, J. Mater. Chem., 2008, 18(43), 5293–5300 RSC.
- G. Deng, H. Huang, C. Peng, A. Zhang, M. Zhang, S. Bo, X. Liu, Z. Zhen and L. Qiu, Synthesis and electro-optic activities of new side-chain polycarbonates containing nonlinear optical chromophores and isolation groups, RSC Adv., 2014, 4(9), 4395–4402 RSC.
- B. Yaowen, S. Naiheng, G. Jianping, S. Xun, W. Xiaomei, Y. Guomin and W. Zhiyuan, A new approach to highly electrooptically active materials using cross-linkable, hyperbranched chromophore-containing oligomers as a macromolecular dopant, J. Am. Chem. Soc., 2005, 127, 2060–2061 CrossRef PubMed.
- G. Deng, H. Huang, P. Si, H. Xu, J. Liu, S. Bo, X. Liu, Z. Zhen and L. Qiu, Synthesis and electro-optic activities of novel polycarbonates bearing tricyanopyrroline-based nonlinear optical chromophores with excellent thermal stability of dipole alignment, Polymer, 2013, 54(23), 6349–6356 CrossRef CAS.
- J. Wu, S. Bo, J. Liu, T. Zhou, H. Xiao, L. Qiu, Z. Zhen and X. Liu, Synthesis of novel nonlinear optical chromophore to achieve ultrahigh electro-optic activity, Chem. Commun., 2012, 48(77), 9637–9639 RSC.
- A. Lederer, W. Burchard, T. Hartmann, J. S. Haataja, N. Houbenov, A. Janke, P. Friedel, R. Schweins and P. Lindner, Dendronized Hyperbranched Macromolecules: Soft Matter with a Novel Type of Segmental Distribution, Angew. Chem., Int. Ed. Engl., 2015, 54(43), 12578–12583 CrossRef CAS PubMed.
- M. He, T. M. Leslie and J. A. Sinicropi, α-Hydroxy Ketone Precursors Leading to a Novel Class of Electro-optic Acceptors, Chem. Mater., 2002, 14(5), 2393–2400 CrossRef CAS.
- C. C. Teng and H. T. Man, Simple reflection technique for measuring the electro-optic coefficient of poled polymers, Appl. Phys. Lett., 1990, 56(18), 1734–1736 CrossRef CAS.
|
This journal is © The Royal Society of Chemistry 2020 |