DOI:
10.1039/C9RA10517D
(Paper)
RSC Adv., 2020,
10, 5202-5211
Tolerance mechanism of Trichoderma asperellum to Pb2+: response changes of related active ingredients under Pb2+ stress†
Received
14th December 2019
, Accepted 17th January 2020
First published on 31st January 2020
Abstract
Trichoderma asperellum ZZY has good tolerance to Pb2+, but the tolerance mechanism is not clear. The manuscript aimed to clarify the tolerance mechanism from the perspective of the response changes of related active ingredients. The synthesis of polysaccharides, proteins and thiol compounds in Trichoderma asperellum can be accelerated with Pb2+ stress. Under Pb2+ stress, Trichoderma asperellum can synthesize oxalic acid and secrete it extracellularly. In addition, high concentration of Pb2+ can inhibit the synthesis and extracellular secretion of formic acid and malic acid. The tolerance of Trichoderma asperellum to Pb2+ is the results of multiple reactions. The Pb2+ can promote the synthesis of polysaccharides, proteins, thiol compounds and oxalic acid. In the early stage of Pb2+ stress, Trichoderma asperellum can rapidly initiate an extracellular emergency mechanism, synthesize oxalic acid in mycelia and secrete it extracellularly to remove free Pb2+ and alleviate the toxicity of Pb2+ to cells. With the transport of Pb2+ into cells, it can promote the synthesis of polysaccharides, proteins, thiol compounds to adsorb and transform the Pb2+ and ease the damage to the cells. The manuscript provides theoretical support and scientific explanation for the application of Trichoderma asperellum.
1. Introduction
Heavy metal refers to the metal elements with a density exceeding 5 g mL−1. Heavy metal elements are initially fixed in the crust in the form of minerals. But with the exploitation of resources, various types and quantities of heavy metal elements are released into the environment to cause serious heavy metal pollution problems. The existence of heavy metals has caused serious global health challenges to human society. The environmental pollution remediation is an urgent problem. The bioremediation is a safe and effective way.1 Various biological materials were utilized to repair environmental pollutions of heavy metals, such as biochar, biochar-based nanocomposite, rhamnolipid (RL)-stabilized nanoscale zero-valent iron, nanoscale zero valent iron particles and so on.2–6 In addition, microorganisms are an important material in bioremediation. Microorganisms are a class of organisms not only with wide distribution, various species and faster reproduction speed but also with strong catabolic capacity and higher metabolic rate. The heavy metals can inhibit the growth and metabolism of organisms and even cause death when the concentration of heavy metals increases to a certain extent.7 The literature reports that some microorganisms have tolerance to heavy metal ions and also can absorb and transform heavy metals. Choudhary et al.8 reported that the Pseudomonas sp. has good tolerance and adsorption characteristics to Ni2+, Co2+, Cu2+ and Cd2+ with maximum adsorption capacities of 1048 nmol mg−1, 845 nmol mg−1, 828 nmol mg−1 and 700 nmol mg−1, respectively. Abd-Alla et al.9 utilized the Rhizobium leguminosarum bv. as an adsorbent to remove Cd2+ and Co2+ from aqueous solution. The maximum adsorption capacities of Cd2+ and Co2+ were 135.3 mg g−1 and 167.5 mg g−1, respectively.
Microorganisms can survive in high concentrations of heavy metals which suggesting that they can form an effective defense system to reduce the toxicity of heavy metals. These defense systems are based on the metabolites synthesized by the cells in the extracellular and intracellular and can chelated with heavy metal ions.10 The cell wall of microorganism contains various biological active substances, such as polysaccharides, proteins and so on. These biological active substances contains functional groups such as a carboxyl, phosphate, hydroxyl, thiol and amino which can combine with heavy metal ions around through electrostatic adsorption, complexation, chelation, ion exchange and covalent adsorption, or form extracellular precipitates with heavy metal ions to prevent them from entering the cell.11–13 The polysaccharides and proteins in EPS were primarily responsible for the resistance the toxicity.14–16 Microorganisms can also immobilize heavy metal ions intracellularly. The metal ions can be transported into the cell through the ion channel of the cell membrane. Intracellular metallothioneins, complexins, and peptides can bind to heavy metal ions and fix the heavy metals.17 Microorganisms can also produce related enzymes which can reduce heavy metal ions to decrease the toxic effects on the cells.18
Trichoderma asperellum is a fungus with strong vitality and simple requirements for nutritional conditions. It has extensive applications due to its faster reproduction speed and larger sporulation. Trichoderma asperellum can synthesize cell wall hydrolase, such as chitinase, glucanase and protease.19–21 Trichoderma asperellum can inhibit the growth of plant pathogens by degrading the cell wall of plant pathogens. Therefore, Trichoderma asperellum is widely used in the biological control of plants.22,23 In addition, Trichoderma asperellum has strong ability to degrade cellulose and it can also be applied to degrade waste with high cellulose content to reduce environmental pollution and develop new energy.24,25 In recent years, it has been found that Trichoderma asperellum has certain tolerance, adsorption and transformation effects on heavy metal ions. Trichoderma asperellum has good application prospect in repairing heavy metal pollution. Su et al.26 studied the tolerance and biotransformation of arsenic (As) by Trichoderma asperellum and found that the strain can transport arsenic to the bacterial cells to reduce and methylate the arsenic to non-toxic form. Chang et al.27 isolated Trichoderma asperellum PTN10 which has tolerance and detoxification ability to chromium. The extracellular enzyme system of PTN10 has strong reducing ability to Cr(VI), which can reduce the toxicity of Cr(VI) to strain. In our team, Zhu et al. screened and isolated a strain of Trichoderma asperellum from heavy metal contaminated soil. It found that this strain has good tolerance to Pb2+.28 This is the first report on the study of Pb2+ tolerance by Trichoderma asperellum. In previously work, a strain of Trichoderma asperellum ZZY was isolated from heavy metal contaminated soil. Trichoderma asperellum ZZY was applied in the National Spark Key Program of China (2015GA610001) and it proved that the Trichoderma asperellum ZZY can effectively reduce the contents of heavy metals in grains, fruits and vegetables through competitive adsorption on heavy metals with plants.
The Trichoderma asperellum ZZY has good tolerance to Pb2+, but the tolerance mechanism is not clear. So the manuscript aimed to clarify the tolerance mechanism from the perspective of the response changes of related active ingredients under Pb2+ stress. The effects of Pb2+ stress on biomass of mycelia were studied and the experiments of Pb2+ stress on Trichoderma asperellum were performed to determine the scientifically and accurately the concentrations of Pb2+ stress. The response changes of the polysaccharides, soluble proteins, thiol compounds and low molecular organic acids in Trichoderma asperellum under stress of Pb2+ were studied to clarify the tolerance mechanism. The concentration of Pb2+ was measured with atomic absorption spectrophotometry (AAS). The content of polysaccharides was detected by phenol-sulfuric acid and 3,5-dinitrosalicylic acid (DNS). The protein and the thiol compounds were measured with spectrocolorimetry. While the low molecular organic acids were detected with HPLC. The purpose of the manuscript was to provide scientific explanation and theoretical support for the application of Trichoderma asperellum.
2. Materials and methods
2.1 Materials and chemicals
Trichoderma asperellum ZZY, isolated from heavy metal contaminated soil, is a strain with resistant to Pb2+. The strain was preserved in China General Microbiological Culture Collection Center on January 14, 2016 and the deposit number is CGMCC no. 12071. The standards of oxalic acid, tartaric acid, formic acid, malic acid, acetic acid, citric acid and fumaric acid were bought from ANPEL Laboratory Technologies (Shanghai, China). The reagents used in HPLC were chromatographically grade. And all reagents used in manuscript were analytical grade.
2.2 Determination of Pb2+ concentration
The atomic absorption spectrophotometry (AAS) was applied to measure the concentrations of Pb2+. The solution with Pb2+ (1 mg mL−1) was used to prepare standard curve. The concentration of Pb2+ in measured solution was calculated with standard curve. The adsorption capacity and the removal ratio of Pb2+ were calculated with formula followed.
where α, C0, C1, m, V and AE represented the adsorption capacity of Pb (mg g−1), initial concentration of Pb2+ (mg L−1), concentration of Pb2+ after adsorption (mg L−1), the quality of adsorbent (g), volume of adsorption system (L) and removal ratio of Pb2+ (%), respectively.
2.3 Experiments of Pb2+ stress on Trichoderma asperellum
Microorganisms can grow normally with low concentration of heavy metal ions, while heavy metal ions with high concentration can cause damage to cells. To determine the scientifically and accurately the concentrations of Pb2+ stress, the effects of Pb2+ stress on biomass of mycelia were studied and the experiments of Pb2+ stress on Trichoderma asperellum were performed.
2.3.1 Effect of Pb2+ stress on biomass of Trichoderma asperellum mycelia. The strain, stored in 4 °C, needs to be activated for three generations. Then the spore suspension, concentration of 4 × 108 cells per mL, was prepared with activated strain and sterile water and applied to the liquid fermentation as seed liquid. The fermentation was performed with Potato Dextrose Medium and seed liquid of 2% at 26 °C and 160 rpm for 72 h. When the fermentation time was 24 h, the Pb(NO3)2 solution was added in the culture medium to make the concentrations of Pb2+ were 0, 50, 100, 200, 400, 800, 1000 and 2000 mg L−1, respectively. In the termination of the fermentation, the culture mediums with different concentrations of Pb2+ were treated with filtration to obtain the mycelia. The mycelia was washed, dried and weighed.
2.3.2 Experiments of Pb2+ stress on Trichoderma asperellum. The liquid fermentation was performed according to 2.3.1. When the fermentation time was 24 h, the Pb(NO3)2 solution was added in the culture medium to make the concentrations of Pb2+ were 10, 25, 50, 100, 200 and 400 mg L−1, respectively. When the fermentation times were 26, 28, 32, 36, 48, 72 and 96 h, the culture mediums with different concentrations of Pb2+ were treated with filtration to obtain the mycelia. The mycelia was washed, dried and weighed. The concentration of Pb2+ in culture mediums was detected and removal ratio Pb2+ was calculated with previously described.
2.4 Response changes of related active ingredients under Pb2+ stress
The tolerance and adsorption of microorganisms on heavy metal are depended on the related active ingredients synthesized by strain. The response changes of the polysaccharides, soluble proteins, thiol compounds and low molecular organic acids in Trichoderma asperellum under stress of Pb2+ were studied to clarify the tolerance mechanism.
2.4.1 Response changes of polysaccharides content in Trichoderma asperellum mycelia under Pb2+ stress. Bio-polysaccharide is a metabolite produced by plants or microorganisms during growth. The polysaccharide contains a functional group such as hydroxyl, carboxyl and amino group which can be effectively combined with metal ions.29,30 The response changes of the polysaccharides in mycelia were explored.The mycelia of Trichoderma asperellum were grinded and extracted with distilled water (1
:
20). The temperature and time were 80 °C and 2 h, respectively. The extraction was performed three times and all supernatant were combined and the volume was set to 250 mL. The total sugar and reducing sugar were measured with phenol-sulfuric acid and 3,5-dinitrosalicylic acid (DNS), respectively.31 The content of polysaccharides was the difference of total sugar and reducing sugar. The response changes of polysaccharides content in Trichoderma asperellum mycelia under Pb2+ stress were investigated.
2.4.2 Response changes of soluble proteins content in Trichoderma asperellum mycelia under Pb2+ stress. The protein is an important active substance in the cells. The heavy metals can attach to the metal-binding sites of proteins and stimulate the synthesis of related enzymes to regulate the synthesis and transport of related ingredients.32 The response changes of the proteins in mycelia were explored.The liquid fermentation was performed according to 2.3.1. The mycelia and culture medium were separated with filtration. The mycelia were washed five times with sufficient distilled water and then three times with pre-cooled phosphate buffer solution (50 mmol mL−1, pH 7.2). The mycelia were pre-cooled at 4 °C for 2–3 h and grinded to fine powder with liquid nitrogen. The mycelia were mixed with phosphate buffer solution (50 mmol mL−1, pH 7.2, 5 mL) and centrifuged for 30 min at 12
000 rpm and 4 °C. The extraction of mycelia was repeated three times to obtain the extract of Trichoderma asperellum mycelia. The response changes of soluble proteins content in Trichoderma asperellum mycelia under Pb2+ stress were investigated with Bradford (G-250) method.
2.4.3 Response changes of thiol compounds contents in Trichoderma asperellum mycelia and culture medium under Pb2+ stress. The thiol compounds are the active ingredients which contain –SH functional group in organism, such as reduced glutathione, phytochelatin, metallothionein, etc. These substances are important components in the detoxification pathway of heavy metal in different organisms.33–35 The content of thiol compounds in the fermentation broth and mycelia were determined to study the response changes of thiol compounds of Trichoderma asperellum under Pb2+ stress.The liquid fermentation was performed according to 2.3.1. The mycelia and culture medium were separated with filtration. The extracts of mycelia were prepared according to 2.4.2. The thiol compounds contents of extracts of mycelia and culture medium were detected accordance with literature. The response changes of thiol compounds contents in mycelia and culture medium under Pb2+ stress were investigated.
2.4.4 Response changes of low molecular organic acid contents in Trichoderma asperellum mycelia and culture medium under Pb2+ stress. Low molecular organic acids, also known as small organic acids, generally refer to organic acids with molecular weight of less than 250 Da. Low molecular organic acids can not only affect the migration and transformation of heavy metal ions in solid and liquid environments, but also affect the toxicity of heavy metals to organisms and the environment.36,37 Therefore, low molecular organic acids play vital roles in controlling the dissolution and biological toxicity of heavy metals. On the one hand, low molecular organic acids can be ionized to change the charge properties of the environment and affect the adsorption of heavy metals by the adsorbent. On the other hand, low molecular organic acids can also have complexation with heavy metal ions and immobilize them to affect the distribution of heavy metals in solid-phase and liquid–liquid environments.38 The content of low molecular organic acids in the fermentation broth and mycelia were determined to study the response changes of low molecular organic acids of Trichoderma asperellum under Pb2+ stress.The extracts of mycelia and culture medium were prepared according to 2.4.2. The oxalic acid, tartaric acid, formic acid, malic acid, acetic acid, citric acid and fumaric acid in extract of mycelia and culture medium were measured with HPLC (RID-20A, Shimadzu, Japan) equipped with Kromasil C18 (5 μm) (TOSOH, Japan) and UV-vis detector. It was eluted with KH2PO4–H3PO4 buffer (0.04 mol L−1, pH 2.5). The conditions of experiment were as follows: injection volume of 10 μL, flow rate of 0.5 mL min−1, detection wavelength of 210 nm and column temperature of 30 °C. The content of the organic acid was quantified using an external standard method. With the pre-test, the formic acid, acetic acid, malic acid, fumaric acid, citric acid, tartaric acid were mixed to detect and oxalic acid alone. The concentration of organic acid and the area of peak were used as horizontal coordinate and vertical coordinate to prepare standard curve.
2.5 Statistical analysis
The statistical analysis was done using SPSS 19.0 software. Data are presented as means ± SD at least three independent experiments.
3. Results and discussion
3.1 Effects of Pb2+ stress on Trichoderma asperellum mycelia biomass
The results related effects of Pb2+ stress on Trichoderma asperellum mycelia biomass were demonstrated in Fig. 1. When the Pb2+ were 0 mg L−1 and 50 mg L−1, the biomass was no significant difference while decreased significantly when 100 mg L−1. Then with the increase of Pb2+ concentration, the biomass began to decrease significantly. Compared with 0 mg L−1, the mycelial biomass decreased by 14.96%, 33.50% and 48.80%, respectively, when Pb2+ concentration of 200, 400 and 800 mg L−1. Therefore, for accurately exploring the tolerance and mechanism of Trichoderma asperellum to Pb2+, the stress experiments were performed in the Pb2+ concentration of 10, 25, 50, 100, 200 and 400 mg L−1.
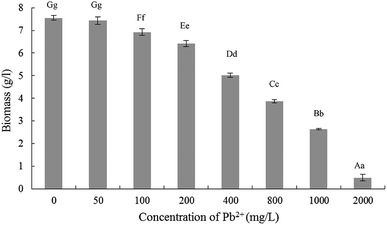 |
| Fig. 1 Effects of Pb2+ on the biomass of Trichoderma asperellum (The different uppercase letters and lowercase letters showed that the difference between the groups was extremely significant (P < 0.01) and significant (P < 0.05), respectively. The same lowercase letters indicated that the difference between the groups was not significant (P > 0.05).). | |
3.2 Experiments of Pb2+ stress on Trichoderma asperellum
The concentration of Pb2+ in fermentation broth and removal ratios of Pb2+ under different concentrations and culture time were shown in Fig. 2. And the significance analysis on removal ratio of Pb2+ was demonstrated in Table S1.† The Fig. 2a and b illustrated that, at initial concentrations of 10 mg L−1 and 25 mg L−1, the removal process of is relatively rapid and completed at 32 h with Pb2+ removal ratios of 98.79% and 99.81%, respectively. The Fig. 2c indicated that, at initial concentrations of 50 mg L−1, the removal process reached equilibrium at 48 h with Pb2+ removal ratio of 96.88%. The Fig. 2d indicated that, at Pb2+ initial concentration of 100 mg L−1, the removal process was relatively slow and reached equilibrium at 72 h with Pb2+ removal ratio of 92.70%. With the fermentation time increased to 96 h, Pb2+ removal ratio had no significantly changes. It can be seen from Fig. 2e and f that, at initial concentrations of 200 mg L−1 and 400 mg L−1, Pb2+ removal ratio both increaseds rapidly in 24–28 h. With the increase of fermentation time, the increase speed of Pb2+ removal ratio had a tendency to slow down in 28–36 h. This may be due to the saturation of the Pb2+ adsorption sites on the surface to inhibit the transportation of Pb2+ into cells in time. It may be caused by the high concentration of Pb2+ can inhibit the growth of Trichoderma asperellum and some response changes should be triggered to accommodate the growing conditions. When the Pb2+ initial concentrations were 200 mg L−1 and 400 mg L−1 and the fermentation time at 96 h, the removal ratios of Pb2+ were 89.66% and 61.49%, respectively. When the Pb2+ initial concentrations were 100, 200 and 400 mg L−1, the decrease speed of Pb2+ concentration in fermentation broth is proportional to the initial concentration of Pb2+.
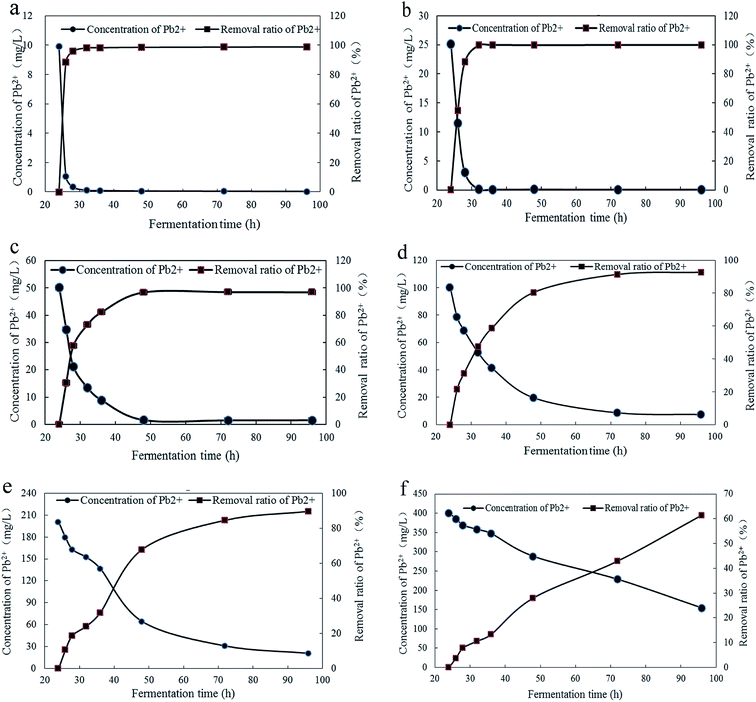 |
| Fig. 2 Changes of Pb2+ concentration and removal ratio of Pb2+ with fermentation time in different initial concentration of Pb2+. (a), (b), (c), (d), (e) and (f) represent the stress experiments were performed with Pb2+ initial concentrations of 10, 25, 50, 100, 200 and 400 mg L−1, respectively. | |
With comprehensive analysis, the Trichoderma asperellum can quickly complete the removal of Pb2+ at low concentrations of Pb2+. This could be because that the Pb2+ can be quickly combined with adsorption sites on the surface or the mycelia secrete active substances precipitated with Pb2+. While the higher the concentration of Pb2+ can urge the startup of the defense system on Pb2+ to alleviate the toxicity on mycelia. To clarify the tolerance mechanism, the response changes of polysaccharides and proteins in mycelia, thiol compounds and low molecular organic acids in mycelia and fermentation broth were further studied. From the above experimental results, Trichoderma asperellum can quickly complete the removal of Pb2+ when the initial concentrations were 10 mg L−1 and 25 mg L−1. Therefore the stress experiments were performed with the Pb2+ initial concentrations of 0, 50, 100, 200 and 400 mg L−1 to reduce the repeatability and increase the accuracy of experiment.
3.3 Response changes of polysaccharides content in Trichoderma asperellum mycelia under Pb2+ stress
The response changes of polysaccharides content in mycelia were illustrated in Fig. 3 and the significance analysis was shown in Table S2.† The contents of polysaccharides in all groups were increased gradually from 24 h to 48 h and no significant changes after 48 h. In the control group (Pb(0)) and experimental group (Pb(50), Pb(100), Pb(200), Pb(400)), when the fermentation time was 48 h, the polysaccharides content were 7.44%, 7.43%, 7.85%, 8.50%, 8.64%, respectively, which indicated that the Pb2+ can promote the synthesis of polysaccharides. The result was consistent with the literature. Li et al. analyzed the biochemical changes of polysaccharides under Pb(II) stress in Rhodotorula mucilaginosa.15 It concluded that the survived cells displayed a stress response and secreted more polysaccharides to resist Pb2+ toxicity at high Pb level. Kopycinska et al. explored the role of extracellular polysaccharide in the response of Rhizobium leguminosarum, free-living and during symbiosis to zinc stress. The zinc stress can stimulate extracellular polysaccharide synthesis and it effectively protected the cells to against the zinc stress.39 The polysaccharides can provide more adsorption sites for Pb2+ and reduce the toxicity of Pb2+ on cells.
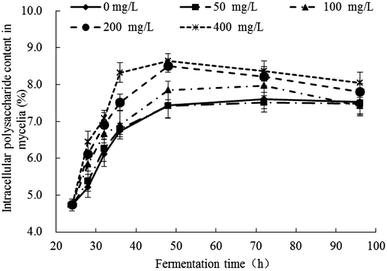 |
| Fig. 3 Response changes of polysaccharides content in mycelia of Trichoderma asperellum under Pb2+ stress. | |
3.4 Response changes of soluble proteins content in Trichoderma asperellum mycelia under Pb2+ stress
The response changes of soluble proteins content in mycelia were illustrated in Fig. 4 and the significance analysis was shown in Table S3.† In all groups, the soluble proteins contents in mycelia decreased sharply in the early stage after the addition of Pb2+ (24–32 h) and the downward trend was inversely proportional to the concentration of Pb2+. There were no difference in the degree of decrease between groups of Pb(0), Pb(50) and Pb(100). But the groups of Pb(200) and Pb(400) had obviously lower degree of decrease. In all groups, the trends remained stable after 32 h. The addition of Pb2+ can affect the normal growth of mycelia and the synthesis of proteins. The Pb2+ stress can stimulate the synthesis of related enzymes to strengthen the synthesis and transport of related ingredients to enhance the tolerance. The conclusion is consistent with literatures. Yuan et al. reported that the small heat shock proteins in Macrobrachium plays vital role against heavy metal toxicity.40 Mota et al. found that the proteins related to photosynthesis, CO2, fixation and carbohydrate metabolism, translation, and nitrogen and amino acid metabolism in Cyanothece sp. CCY 0110 had significant difference in the presence and absence of heavy metal ions.41
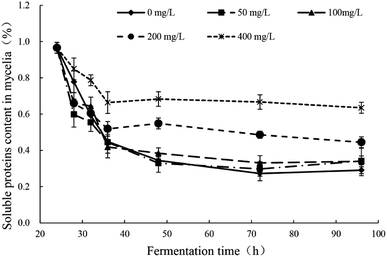 |
| Fig. 4 Response changes of soluble proteins content in mycelia of Trichoderma asperellum under Pb2+ stress. | |
3.5 Response changes of thiol compounds contents in fermentation broth and mycelia of Trichoderma asperellum under Pb2+ stress
The response changes of thiol compounds content (significance analyses) in the fermentation broth and mycelia were demonstrated in Fig. 5a (Table S4†) and Fig. 5b (Table S4†), respectively. In Fig. 5a, the thiol compound contents in all groups gradually increased at 24–32 h, reached maximum at 32 h and then gradually decreases with the increase of culture time. The rate and extent of reduction is inversely proportional to the initial concentration of Pb2+. In Fig. 5b, the thiol compounds contents in the mycelia increased first and then decreased with the culture time in all groups. The increase degree of the experimental group was higher than the control group. In the groups of Pb(100) and Pb(200), the increase trends of thiol compounds was obvious and, at 48 h, the thiol compounds contents reached the maximum (59.44 μmol g−1 and 65.00 μmol g−1, respectively) which were significantly higher than control group (45.44 μmol g−1). The thiol compounds content in the group of Pb(400) was similar with Pb(50) which revealed that the Pb2+ can inhibit the production of thiol compounds. With comprehensive analysis, it can be concluded that Pb2+ stress can promote the synthesis of thiol compounds, but high concentrations of Pb2+ have inhibition to the synthesis. The thiol compounds content in the mycelia reached the highest while in the fermentation broth was in a downward trend which revealed the Pb2+ inhibits extracellular secretion of thiol compounds. With comparison of Fig. 5a and b, in the late stage of Pb2+ stress, there are many thiol compounds can be synthesized by mycelia but less thiol compounds was secreted extracellularly. It is speculated that, with the transportation of Pb2+ into the intracellular, the mycelia can synthesize thiol compounds to complex or transform Pb2+ intracellular. But this mechanism can be affected by the concentration of Pb2+. This conclusion is consistent with the literatures. Xu et al. explored the response changes of thiol compounds in Agaricus bisporus under stress of heavy metal ions. It revealed that the thiol compounds contents was promoted under the stress of Cu2+ and Zn2+ which indicated that the stress of Cu2+ and Zn2+ can induce the synthesis of thiol compounds of Agaricus bisporus.42 Guimaraes-Soares et al. studied the response changes of thiol compounds in Fontanospora fusiramosa and Flagellospora curta under stress of heavy metal ions. The results indicated that the stress of Cd2+ can induce the synthesis of protein thiol compounds in mycelia.33
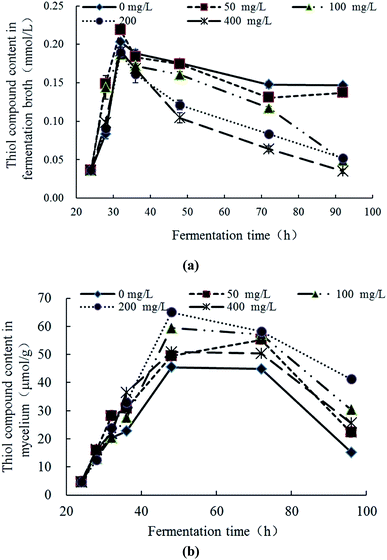 |
| Fig. 5 Response changes of thiol compounds in fermentation broth (a) and mycelia (b) Trichoderma asperellum under Pb2+ stress. | |
3.6 Response changes of low molecular organic acid contents in Trichoderma asperellum mycelia and fermentation broth under Pb2+ stress
The contents of low molecular organic acids were measured with HPLC. In Fig. 6a and b, the retention times of oxalic acid, tartaric acid, formic acid, malic acid, acetic acid, citric acid and fumaric acid were 2.348 min, 2.659 min, 2.868 min, 3.342 min, 4.349 min, 6.018 min and 6.554 min, respectively. The fermentation broth and cell extracts of mycelial were detected by HPLC. The results indicated that, under Pb2+ stress, the contents of oxalic acid, formic acid and malic acid in fermentation broth and mycelia had significant response changes. Therefore, the response changes of oxalic acid, formic acid and malic acid under Pb2+ stress were analyzed.
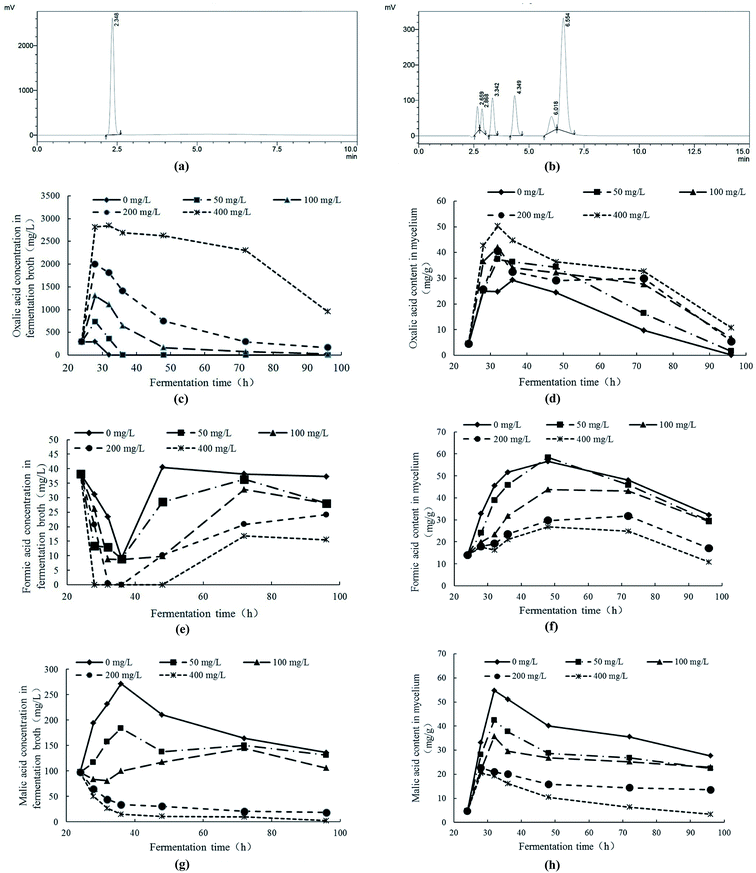 |
| Fig. 6 (a) HPLC chromatogram of oxalic acid; (b) HPLC chromatograms of tartaric acid, formic acid, malic acid, acetic acid, citric acid and fumaric acid (2.659 min: tartaric acid; 2.868 min: formic acid; 3.342 min: malic acid; 4.349 min: acetic acid; 6.018 min: citric acid; 6.554 min: fumaric acid); response changes of oxalic acid content in fermentation broth (c) and mycelia (d) of Trichoderma asperellum under Pb2+ stress; response changes of formic acid content in fermentation broth (e) and mycelia (f) of Trichoderma asperellum under Pb2+ stress; response changes of malic acid content in fermentation broth (g) and mycelia (h) of Trichoderma asperellum under Pb2+ stress. | |
3.6.1 Response changes of oxalic acid. The response changes of oxalic acid content (significance analyses) with culture times in fermentation broth and mycelia were illustrated in Fig. 6c (Table S6†) and Fig. 6d (Table S7†), respectively. In Fig. 6c, the oxalic acid contents in fermentation broth had significant difference in all groups. The oxalic acid content was 292.5 mg L−1 when the fermentation was 24 h. Then the Pb2+ was added into the fermentation broth. After 4 h, the oxalic acid content in group of Pb(0) had no obviously change, but the experimental groups increased significantly. The increase degree of oxalic acid content in fermentation broth was proportional to the initial concentration of Pb2+. With the increase of culture time, the oxalic acid contents slowly decreased to a stable level except for the experimental group of Pb(400). The Fig. 6c indicated that the oxalic acid contents in mycelia increases first and then decreases with the increase of fermentation time. With the addition of Pb2+, the oxalic acid in the mycelia increased sharply in all experimental groups. The increase degree of oxalic acid in mycelia was proportional to the initial concentration of Pb2+. After 36 h, the oxalic acid in the mycelia in all groups gradually decreased. The downward trend and the oxalic acid in the mycelia were inversely proportional and proportional, respectively, to the initial concentration of Pb2+. Comparing the contents of oxalic acid in group of Pb(0) and experimental groups (Pb(50) and Pb(100)), the content of oxalic acid in the fermentation broth is very low, while the synthesis of oxalic acid in the mycelia does not stop with the increase of fermentation time. It indicated that, with the transport of Pb2+ into mycelia, the decreased of Pb2+ concentration in the fermentation broth can induce the closure of cellular pathways related to the transportation of oxalic acid. With comprehensive analysis, it can be speculated that the addition of Pb2+ can induce the synthesis of oxalic acid, promote the efflux of oxalic acid in the mycelia and accelerate the secretion of oxalic acid. The oxalic acid can be reacted with Pb2+ and generated precipitate to remove the free Pb2+ and alleviate the toxicity of Pb2+ to mycelia.43 With the transportation of Pb2+ from fermentation broth to mycelia, the amount of oxalic acid secreted by mycelia is also gradually reduced. In conclusion, the oxalic acid played a crucial role in the tolerance of Trichoderma asperellum to Pb2+.
3.6.2 Response changes of formic acid. The response changes of formic acid content (significance analyses) with culture times in fermentation broth and mycelia were illustrated in Fig. 6e (Table S8†) and Fig. 6f (Table S9†), respectively. As Fig. 6e showed, the formic acid content in the fermentation broth decreased first and then increased with the increase of fermentation time in all groups. The reduction rate of formic acid in fermentation broth and the rate of recovery in late stage were proportional and inversely proportional to the initial concentration of Pb2+, respectively. In group of Pb(0), the formic acid was decreased from 38.31 mg L−1 to 9.13 mg L−1 and the formic acid returned to 40.48 mg L−1 and remained stable with the increase of fermentation time. In groups of Pb(200) and Pb(400), the formic acid in the fermentation broth decreased from the initial of 38.31 mg L−1 (24 h) to none (32 h) and there is a rebound trend in the late stage of fermentation. In groups of Pb(50) and Pb(100), the change trend was similar to control group, but the change rates were between the group of Pb(0) and the group of Pb(400). The Fig. 6f showed that the formic acid in mycelia was greatly affected under stress of Pb2+. In all groups, the formic acid increased first and then decreased. However, the increase degrees of formic acid were significantly different. The Pb2+ can significantly inhibit the increase of formic acid and the inhibition was proportional to the concentration of Pb2+. In summary, Pb2+ stress can inhibit the synthesis of formic acid and, in the early stage of Pb2+ stress, the sharply decrease of formic acid in the fermentation broth may be due to the extracellular secretion of oxalic acid cause inhibition on the formic acid secretion.
3.6.3 Response changes of malic acid. The response changes of malic acid content (significance analyses) with culture times in fermentation broth and mycelia were illustrated in Fig. 6g (Table S10†) and Fig. 6h (Table S11†), respectively. The Fig. 6g showed that the Pb2+ stress had significant effect on the malic acid in fermentation broth. In group of Pb(0), the malic acid increased in 24–36 h, reached the highest (271.58 mg L−1) at 36 h and gradually decreased after 36 h. In group of Pb(50), the response changes of the malic acid was similar to group of Pb(0) but the rate and extent of changes were weaker at 24–36 h and reached the highest (183.82 mg L−1) at 36 h. In group of Pb(100), the change trend is slowly decreased first, then starts to rise after 32 h. In groups of Pb(200) and Pb(400), the change trends of malic acid were similar to each other and, after the addition of Pb2+, the malic acid decreased with the increase of fermentation time. The Fig. 6h showed that, the change trends of malic acid in mycelia were increased first and then decreased in all groups. In group of Pb(0), Pb(50) and Pb(100), the malic acid in the mycelia reached the maximum at 36 h which were 54.79 mg g−1, 42.47 mg g−1 and 35.70 mg g−1, respectively, and then gradually decreased to a steady state with the increase of fermentation time. While in groups of Pb(200) and Pb(400), the malic acid reached the maximum at 28 h which were 22.72 mg g−1 and 20.45 mg g−1, respectively. The malic acid decreased to a steady trend with the increase of fermentation time in group of Pb(200) and gradually decreased in group of Pb(400). With comprehensive analysis of Fig. 6g and h, it can be concluded that the Pb2+ can inhibit the synthesis and secretion of malic acid in Trichoderma asperellum and the inhibition is proportional to the concentration of Pb2+.With the comprehensive analysis of response changes of oxalic acid, formic acid and malic acid in fermentation broth and mycelia of Trichoderma asperellum under Pb2+ stress, it can be obtained the following conclusions. Under Pb2+ stress, Trichoderma asperellum can synthesize large amounts of oxalic acid and secrete it extracellularly to eliminate the free Pb2+ in the system and alleviate the toxicity of Pb2+ to mycelia. In addition, the high concentration of Pb2+ can not only inhibit the synthesis and extracellular secretion of formic acid and malic acid. The oxalic acid is a low molecular organic acid and commonly found in living organisms. There are many ways to synthesize it and one of the synthetic pathways is the oxaloacetate fragmentation pathway.44 The oxaloacetate is an important substance involved in the tricarboxylic acid cycle (TCA), while oxaloacetate in TCA can be synthesized by malic acid with the catalysis of malate dehydrogenase.45 It is speculated that Pb2+ stress promotes the conversion of malic acid to oxaloacetate which has promotion on the production of oxalic acid. On the other hand, the oxalic acid can be degraded to formic acid and carbon dioxide. The Pb2+ stress can inhibit the synthesis and secretion of formic acid in Trichoderma asperellum which may be due to the inhibition of Pb2+ stress on the conversion of oxalic acid to formic acid.
In conclusion, under stress of Pb2+, the polysaccharides, soluble proteins, thiol compounds, oxalic, formic acid and malic acid all had varying degrees response changes to enhance the tolerance of Trichoderma asperellum to Pb2+. The synthesis of polysaccharides and proteins can be accelerated to enhance the tolerance. The synthesis of thiol compounds can be promoted with Pb2+ stress to complex or transform Pb2+ intracellular. Under Pb2+ stress, Trichoderma asperellum can synthesize large amounts of oxalic acid and secrete it extracellularly to eliminate the free Pb2+ in the system and alleviate the toxicity of Pb2+ to mycelia. In addition, the high concentration of Pb2+ can inhibit the synthesis and extracellular secretion of formic acid and malic acid. By comprehensively analysis, it revealed that the tolerance of Trichoderma asperellum to Pb2+ is the results of multiple reactions. The Pb2+ can promote the synthesis of polysaccharides, proteins, thiol compounds and oxalic acid. In the early stage of Pb2+ stress, Trichoderma asperellum can rapidly initiate an extracellular emergency mechanism, synthesize oxalic acid in mycelia and secrete it extracellularly to remove free Pb2+ and alleviate the toxicity of Pb2+ to cells. With the transport of Pb2+ into cells, it can promote the synthesis of polysaccharides, proteins, thiol compounds to adsorb and transform the Pb2+ and ease the damage to the cells.
4. Conclusion
The manuscript mainly studied the tolerance mechanism of Trichoderma asperellum to Pb2+ with exploring the response changes of related active ingredients under Pb2+ stress. The polysaccharides, soluble proteins, thiol compounds, oxalic, formic acid and malic acid all had varying degrees response changes to enhance the tolerance of Trichoderma asperellum to Pb2+. The tolerance of Trichoderma asperellum to Pb2+ is the results of multiple reactions. The manuscript provides theoretical support for the application of Trichoderma asperellum and has contributions to the remediation of heavy metal pollution in the environment and the environmental safety.
Conflicts of interest
None.
Acknowledgements
This work was financially supported by the National Natural Science Foundation of China (31871791), Technology Program of Tianjin, China, (18ZYPTJC00020), the key program of the Foundation of Tianjin Educational Committee (2018ZD06) and the National Spark Key Program of China (2015GA610001).
References
- S. Ye, G. Zeng, H. Wu, C. Zhang, J. Dai, J. Liang, J. Yu, X. Ren, H. Yi, M. Cheng and C. Zhang, Crit. Rev. Biotechnol., 2017, 37, 1062–1076 CrossRef CAS.
- S. Ye, G. Zeng, H. Wu, J. Liang, C. Zhang, J. Dai, W. Xiong, B. Song, S. Wu and J. Yu, Resour. Conserv. Recycl., 2019, 140, 278–285 CrossRef.
- S. Ye, M. Yan, X. Tan, J. Liang, G. Zeng, H. Wu, B. Song, C. Zhou, Y. Yang and H. Wang, Appl. Catal., B, 2019, 250, 78–88 CrossRef CAS.
- W. Xue, Z. Peng, D. Huang, G. Zeng, J. Wan, R. Xu, M. Cheng, C. Zhang, D. Jiang and Z. Hu, J. Hazard Mater., 2018, 359, 290–299 CrossRef CAS PubMed.
- W. Xue, D. Huang, G. Zeng, J. Wan, M. Cheng, C. Zhang, C. Hu and J. Li, Chemosphere, 2018, 210, 1145–1156 CrossRef CAS PubMed.
- X. Gong, D. Huang, Y. Liu, G. Zeng, R. Wang, J. Wan, C. Zhang, M. Cheng, X. Qin and W. Xue, Environ. Sci. Technol., 2017, 51, 11308–11316 CrossRef CAS PubMed.
- P. T. Gauthier, W. P. Norwood, E. E. Prepas and G. G. Pyle, Aquat. Toxicol., 2014, 154, 253–269 CrossRef CAS.
- S. Choudhary and P. Sar, Bioresour. Technol., 2009, 100, 2482–2492 CrossRef CAS.
- M. H. Abd-Alla, F. M. Morsy, A.-W. E. El-Enany and T. Ohyama, Int. Biodeterior. Biodegrad., 2012, 67, 48–55 CrossRef CAS.
- G. Zeng, D. Huang, G. Huang, T. Hu, X. Jiang, C. Feng, Y. Chen, L. Tang and H. Liu, Bioresour. Technol., 2007, 98, 320–326 CrossRef CAS PubMed.
- M. Rajkumar, N. Ae, M. N. Prasad and H. Freitas, Trends Biotechnol., 2010, 28, 142–149 CrossRef CAS PubMed.
- J. J. Harrison, H. Ceri and R. J. Turner, Nat. Rev. Microbiol., 2007, 5, 928–938 CrossRef CAS.
- M. R. Bruins, S. Kapil and F. W. Oehme, Ecotoxicol. Environ. Saf., 2000, 45, 198–207 CrossRef CAS PubMed.
- Z. Teng, W. Shao, K. Zhang, Y. Huo, J. Zhu and M. Li, Chem. Eng. J., 2019, 375, 122113 CrossRef CAS.
- J. J. Li, Z. Q. Jiang, S. S. Chen, T. Wang, L. Jiang, M. X. Wang, S. M. Wang and Z. Li, Ecotoxicol. Environ. Saf., 2019, 174, 484–490 CrossRef CAS PubMed.
- S. Kumari, S. Mahapatra and S. Das, Chem. Eng. J., 2017, 328, 556–566 CrossRef CAS.
- A. Rorat, F. Vandenbulcke, A. Galuszka, B. Klimek and B. Plytycz, Comp. Biochem. Physiol., Part C: Toxicol. Pharmacol., 2017, 203, 39–50 CAS.
- A. Bafana, K. Krishnamurthi, M. Patil and T. Chakrabarti, J. Hazard Mater., 2010, 177, 481–486 CrossRef CAS PubMed.
- R. da Silva Aires, A. S. Steindorff, M. H. S. Ramada, S. J. L. de Siqueira and C. J. Ulhoa, Carbohydr. Polym., 2012, 87, 1219–1223 CrossRef.
- D. Errandonea, R. Boehler and M. Ross, Phys. Rev. B: Condens. Matter Mater. Phys., 2015, 92, 026101 CrossRef.
- A. A. Silveira, J. P. Andrade, A. C. P. Guissoni, H. G. Silva, G. L. Souza and K. F. Fernandes, J. Biotechnol., 2017, 256, S110 CrossRef.
- S. Vinodkumar, T. Indumathi and S. Nakkeeran, Biol. Contr., 2017, 113, 58–64 CrossRef.
- J. C. da Silva, N. D. Suassuna and W. Bettiol, Crop Protect., 2017, 94, 28–32 CrossRef.
- Q. Wang, H. Lin, Q. Shen, X. Fan, N. Bai and Y. Zhao, PloS One, 2015, 10, e0119237 CrossRef PubMed.
- S. Raghuwanshi, D. Deswal, M. Karp and R. C. Kuhad, Fuel, 2014, 124, 183–189 CrossRef CAS.
- S. Su, X. Zeng, L. Bai, L. Li and R. Duan, Sci. Total Environ., 2011, 409, 5057–5062 CrossRef CAS.
- F. Chang, C. Tian, S. Liu and J. Ni, Chem. Eng. J., 2016, 298, 75–81 CrossRef CAS.
- Z. Zhu, Q. Song and F. Dong, J. Basic Microbiol., 2018, 58, 368–376 CrossRef CAS PubMed.
- B. Pourrut, S. Jean, J. Silvestre and E. Pinelli, Mutat. Res., 2011, 726, 123–128 CAS.
- H. M. Li, M. Wei, W. H. Min, Y. W. Gao, X. Q. Liu and J. S. Liu, Biocatal. Agric. Biotechnol., 2016, 6, 28–32 CrossRef.
- Y. Tang, Z. Y. Zhu, Y. Liu, H. Sun, Q. Y. Song and Y. Zhang, Food Funct., 2018, 9, 2300–2312 RSC.
- B. Khatiwada, M. T. Hasan, A. Sun, K. S. Kamath, M. Mirzaei, A. Sunna and H. Nevalainen, Algal Res., 2020, 45, 101764 CrossRef.
- L. Guimaraes-Soares, C. Pascoal and F. Cassio, Ecotoxicol. Environ. Saf., 2007, 66, 36–43 CrossRef CAS.
- A. Jarosz-Wilkolazka, M. Graz, B. Braha, S. Menge, D. Schlosser and G. J. Krauss, BioMetals, 2006, 19, 39–49 CrossRef CAS.
- S. K. Kawakami and E. P. Achterberg, Estuar. Coast, 2011, 35, 658–664 CrossRef.
- M. Mäkelä, S. Galkin, A. Hatakka and T. Lundell, Enzyme Microb. Technol., 2002, 30, 542–549 CrossRef.
- S. Galkin, T. Vares, M. Kalsi and A. Hatakka, Biotechnol. Tech., 1998, 12, 267–271 CrossRef CAS.
- J. K. Magnuson and L. L. Lasure, Adv. Fungal Biotechnol. Ind., Agric., Med., 2004, 307–340, DOI:10.1007/978-1-4419-8859-1_12.
- M. Kopycinska, P. Lipa, J. Ciesla, M. Koziel and M. Janczarek, Environ. Microbiol. Rep., 2018, 10, 355–368 CrossRef CAS PubMed.
- F. Yuan, Z. Yang, T. Tang, S. Xie and F. Liu, Fish Shellfish Immunol., 2019, 95, 635–643 CrossRef CAS.
- R. Mota, S. B. Pereira, M. Meazzini, R. Fernandes, A. Santos, C. A. Evans, R. De Philippis, P. C. Wright and P. Tamagnini, J. Proteomics, 2015, 120, 75–94 CrossRef CAS PubMed.
- H. Xu, P. Song, W. Gu and Z. Yang, Ecotoxicol. Environ. Saf., 2011, 74, 1685–1692 CrossRef CAS.
- N. J. Li, G. M. Zeng, D. L. Huang, S. Hu, C. L. Feng, M. H. Zhao, C. Lai, C. Huang, Z. Wei and G. X. Xie, Bioresour. Technol., 2011, 102, 8137–8142 CrossRef CAS.
- G. J. Ruijter, P. L. I. van de Vondervoort and J. Visser, Microbiology, 1999, 145(pt 9), 2569–2576 CrossRef CAS PubMed.
- D. A. Bender, Encyclopedia of Food Sciences & Nutrition, 2003, vol. 50, pp. 5851–5856 Search PubMed.
Footnote |
† Electronic supplementary information (ESI) available. See DOI: 10.1039/c9ra10517d |
|
This journal is © The Royal Society of Chemistry 2020 |