DOI:
10.1039/C9RA10480A
(Paper)
RSC Adv., 2020,
10, 6801-6806
Highly selective colorimetric determination of catechol based on the aggregation-induced oxidase–mimic activity decrease of δ-MnO2†
Received
13th December 2019
, Accepted 7th February 2020
First published on 13th February 2020
Abstract
Multiple enzyme-like activities of manganese oxides (MnO2) have been reported and applied in catalysis, biosensors, and cancer therapy. Here, we report that catechol can be determined colorimetrically based on the 3,3′,5,5′-tetramethylbenzidine (TMB) oxidase-like activity of δ-MnO2. The detection was based on pre-incubation of catechol containing water samples with δ-MnO2, and then the residual TMB oxidase-like activity of reacted δ-MnO2 was linearly dependent on the catechol concentration in the range of 0.5 to 10 μM. This determination method was stable at pH 3.73–6.00 and was not affected by ion strength up to 200 μM. Common co-solutes in water bodies (50 μM) have negligible effects and excellent selectivity of catechol among various phenolic compounds (15 μM) was facilitated. Both reduction and aggregation of δ-MnO2 were observed during the incubation process with catechol, and aggregation-induced TMB oxidase–mimic activity decrease was the main factor for this colorimetric determination.
1. Introduction
Phenolic compounds widely exist in natural water bodies and industrial wastewaters, and exhibit adverse effects on human health and the environment.1 Among these compounds, catechol has attracted concern because of its widespread occurrence in nature and its potential toxicity to human beings. It is used as an antifungal agent on seed potatoes or as an antioxidant in the rubber, dye, pharmaceutical and oil industries.2 It is also an important intermediate produced by bacteria during aerobic aromatic compound degradation. For human beings, 50 mg L−1 catechol is enough to cause significant changes in erythrocyte function.3 Herein, a variety of methods, such as UV-vis spectrophotometry, liquid chromatography, electrochemical analysis, and flow injection analysis, have been developed to facilitate catechol determination.4–8 However, the intrinsic drawbacks, such as complicated instruments, time-consuming pre-treatment, and low determination limits, restrict the practical application of these methods.
In the early decades of the 21st century, unexpected enzyme-like activities of nanomaterials were observed, and those nanomaterials were termed “nanozymes”.9 By employing the peroxidase, catalase, superoxide dismutase, and hydrogenase-like activities of different nanozymes, a variety of analytic platforms have been established through electrochemical and luminescence signal changes induced by substrate concentration.10–12 MnO2 is a relatively new type of nanozyme, that was reported to possess oxidase-like activity against 3,3′,5,5′-tetramethylbenzidine (TMB).13–16 The blue oxidation product of TMB (ox-TMB) has a large molar extinction coefficient of 39
000 M−1 cm−1 at 652 nm in UV-vis spectra, which makes this oxidation reaction suitable for colorimetric detection.17 In general, the TMB–MnO2 system can be applied to quantitatively determine any substrate that can inhibit the oxidase-like activity of MnO2. The inhibition effect is usually caused by either the redox reaction between the substrate and MnO2, or the strong absorption of substrate on the surface of MnO2.18 In the case of catechol, unfortunately, these two approaches cannot apply enough selectivity and sensitivity because the reaction rate and adsorption capacity of catechol with MnO2 are not superior to those of other phenolics. Interestingly, reduction-induced MnO2 aggregation by catechol, guaiacol and 4-methylguaiacol was observed, while 4-methylphenol and p-cresol cannot induce MnO2 aggregation.19 This phenomenon inspired us to determine whether this “reduction-induced aggregation” can be used for catechol determination with satisfactory selectivity and sensitivity.
In this paper, δ-MnO2 was synthesized, and its TMB oxidase-like activity was confirmed and optimized. Then, TMB oxidase-like activity was successfully used to determine catechol with good sensitivity and selectivity. This nanozyme-based system also exhibited a reasonable RSD value and recovery ratio in real water samples. Moreover, an aggregation-based determination mechanism of catechol was proposed.
2. Experimental
2.1 Materials and chemicals
TMB, dopamine (DOPA) and 2,2′-azino-bis(3-ethylbenzothiazoline-6-sulfonic acid) (ABTS) were purchased from Sigma-Aldrich (Steinheim, Germany), and methanol and dimethyl sulfoxide (DMSO) were purchased from Merck (Shanghai, China). All of the other chemicals were of analytical grade and purchased from Damao (Tianjin, China) or Aladdin (Shanghai, China). The metal salts used in this study were all in the chloride form, and the anions were all in the sodium form.
2.2 Synthesis and characterization
Birnessite-type MnO2 (δ-MnO2) was obtained through the redox reaction between KMnO4 and methanol, as our previous work mentioned.20 The products were collected and characterized. The morphology of δ-MnO2 was observed using transmission electron microscopy (TEM, Tecnai F30, FEI), and the chemical environment and element valences of the material surface were determined by X-ray photoelectron spectroscopy (ESCALAB™ 250Xi). The hydrodynamic radius of nanoparticles was determined by a nano-ZS zetasizer (Malvern, England). The Mn concentration in the solution was determined by atomic absorption spectroscopy (AAS, Techcomp AA6100). The catechol concentration was determined by high-performance liquid chromatography (HPLC, Agilent 1290). A gradient elution method was used for catechol determination. Mobile phase A was ultrapure water, and mobile phase B was acetonitrile. The gradient was as the follows (% B): 0 min (20%), 3 min (95%), 8 min (95%), and 8.1 min (20%). The re-equilibration time was 1.9 min. The flow rate was kept at 0.4 mL min−1.
2.3 TMB oxidase-like activity determination
In general, 5 mg δ-MnO2 power was added to 25 mL 20 mM HAc–NaAC buffer and sonicated for 5 min to obtain δ-MnO2 dispersions. Then, 50 μL of these dispersions was added to 1930 μL 20 mM HAC–NaAC buffer in a cuvette. Furthermore, 50 mg TMB was added to 5 mL DMSO to prepare a stock solution of TMB. 20 μL of this stock TMB solution was mixed with the pre-added δ-MnO2 dispersions and buffer, and reacted for 5 min. The absorbance at 652 nm (A652 nm) was monitored in either fixed wavelength or whole wavelength scanning mode (500 to 800 nm) by a UV-vis spectroscopy (723PC, SOPTOP Co. Ltd., China). To investigate the effect of pH, different buffers were used as follows: HAC–NaAC (pH 3.73 to 5.00, 20 mM), MES (pH 6.00, 20 mM), HEPES (pH 7.00, 20 mM) and Tris–HCl (pH 8.00 to 9.00, 20 mM). The ion strength referred to the concentration of HAc–NaAc buffer. The concentrations of common anions and cations were 50 μM. For the selectivity experiments, all of the phenolic compounds were used at a final concentration of 15 μM.
3. Results and discussion
3.1 TMB oxidase-like activity of δ-MnO2
The TMB oxidase-like activity of as-synthesized δ-MnO2 was first determined by UV-vis spectroscopy. As shown in Fig. 1a, A652 nm increased with the δ-MnO2 concentration, reaching approximately 1.4 with the addition of 5 mg L−1 δ-MnO2. A further increase in the δ-MnO2 concentration to 8 mg L−1 resulted in the value of A652 nm increasing to 2.5, which means that dilution of the sample was needed to obtain an accurate value because of the limitation of the Lamber–Bill law. Increase in the TMB concentration first increased A652 nm, with 5 mg L−1 TMB giving an A652 nm of 1.42. Further increase in the TMB concentration resulted in only a slight increase in A652 nm (Fig. 1b). The oxidation of TMB was fast, and A652 nm reached a stable value within 300 s (Fig. 1c). The TMB oxidase activity of δ-MnO2 is highly pH-dependent and maintained a relatively high value between pH 3.73 and 6.00, and then decreased sharply at pH 8.00 (Fig. 1d). This pH-dependent activity has been reported widely and is thought to be related to the oxidation power of MnO2 itself.21 Common coexisting cations and anions, together with a range of ion strengths (5 to 200 mM) showed insignificant effects on the TMB oxidase activity (Fig. 1e and f). Therefore, the following experiments were carried out under the following reaction conditions unless otherwise mentioned: [MnO2] = 5 mg L−1, [TMB] = 5 mg L−1, pH 3.73 (HAc–NaAc buffer), 20 mM NaCl, and a reaction time of 5 min.
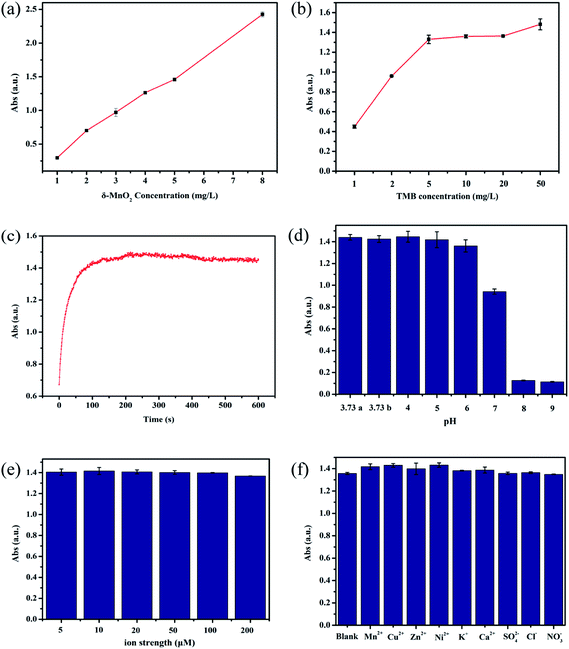 |
| Fig. 1 Effects of a variety of factors on the TMB oxidase-like activity of δ-MnO2. (a) Effect of δ-MnO2 concentration. (b) Effect of TMB concentration. (c) Effect of reaction time. (d) Effect of pH. 3.72a was assayed in 100 mM NaCl, while 3.72b was assayed in HAC–NaAC buffer. (e) Effect of ion strength. (f) Effect of common anions and cations. | |
3.2 Linearity and sensitivity to catechol determination
Then, different concentrations of catechol solution were mixed with δ-MnO2 dispersions, and TMB was added to this solution to determine the residual TMB oxidase activity immediately. It was found that adding catechol has a negligible effect on the TMB oxidation activity. However, if catechol was pre-incubated with δ-MnO2 for 2 h and then reacted with TMB for 5 min, a significant decrease in A652 nm was observed, and 15 μM catechol completely quenched the oxidase-like activity of δ-MnO2 (Fig. 2a). The color change from bright blue to colorless could be observed by the naked-eye when the catechol concentration increased from 0 to 15 μM (Fig. 2b). The ΔA652 nm was linearly related to the catechol concentration in the range of 0.5 to 10 μM, with a calculated limit of determination (LOD) of 218 nM (n = 11) and limit of quantification (LOQ) of 726 nM (Fig. 2c). Electrochemical and fluorescence-based determination methods usually have higher sensitivities than colorimetric-based methods, but the instruments are more expensive than that needed for UV-vis spectroscopy.22,23 However, the performance of TMB-δ-MnO2 for catechol determination is comparable to most of the reported catechol sensors, as summarized in Table 1. In addition to TMB, DOPA and ABTS were also employed to test the oxidase-like activity of δ-MnO2. DOPA is a specific oxidase substrate for CeNPs and cannot be oxidized by as-synthesized δ-MnO2.24 ABTS, however, can also be effectively oxidized by δ-MnO2 like TMB and forms a dark green product (Fig. 2d and e). The linear range was 0.5 to 10 μM, with a calculated LOD of 190 nM (n = 11) and LOQ of 577 nM (Fig. 2f).
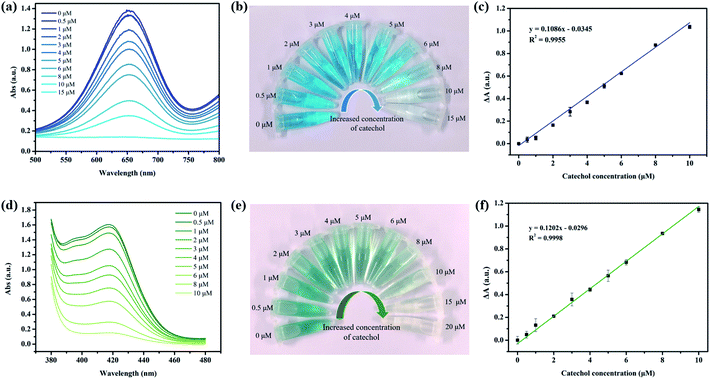 |
| Fig. 2 (a) UV-vis absorption spectra of the resulting solution upon sequential addition of δ-MnO2 pre-incubated with different concentrations of catechol and TMB. (b) The color change of δ-MnO2 incubated with different concentrations of catechol and then reacted with TMB. (c) Catechol calibration curve using TMB as the substrate. (d) UV-vis absorption spectra of δ-MnO2 pre-incubated with different concentrations of catechol and ABTS. (e) The color change of δ-MnO2 incubated with different concentrations of catechol and then reacted with ABTS. (f) Catechol calibration curve using ABTS as the substrate. | |
Table 1 Comparison of the catechol determination methods
Method |
System |
LOD (μM) |
Linear range (μM) |
Ref. |
Colorimetric |
ssDNA-AuNPs |
0.11 |
0.2–7 |
25 |
Electrochemical |
Fe3O4-GO-AuNPs |
0.8 |
2–145 |
5 |
Electrochemical |
Palygorskite-CPE |
0.57 |
5–100 |
7 |
Electrochemical |
Array BDD |
1.5 |
5–100 |
26 |
Fluorescence |
[C3(Amp)2][OH]2 |
0.4 |
1–1000 |
27 |
Colorimetric |
TMB-δ-MnO2 |
0.22 |
0.5–10 |
This work |
3.3 Selectivity and practical application
Considering that the colorimetric determination procedure involves a relatively long pre-incubation process between the catechol-containing solution and δ-MnO2, the effects of pH, cations and anions, incubation time, and ion strength on the final TMB oxidation activity (i.e., A652 nm) were also investigated. The A652 nm remained stable when the pH was between 3.73 and 6.00 in the presence of various ions, regardless of the ion strength (5–200 mM) (Fig. S1†). However, the incubation time of catechol and δ-MnO2 has a great effect on A652 nm (Fig. 3a). For 10 μM catechol, the A652 nm decreased nearly linearly with incubation time within 120 min, and then decreased more slowly with prolonged incubation. Therefore, 120 min was sufficient to obtain satisfactory sensitivity. To investigate selectivity, several phenolic compounds were incubated with δ-MnO2 separately in a similar way as catechol and tested for the corresponding TMB oxidation activity. δ-MnO2-TMB has at least a 3-fold higher response for catechol than the other phenolic compounds at pH 3.73 (Fig. 3b). The selectivity can be further improved by adjusting the solution pH to 6.00. Under this condition, the catechol response of δ-MnO2-TMB is at least 160-fold higher than that of the other compounds, demonstrating the high selectivity for catechol (Fig. 3c). The reductive compounds, including ascorbic acid, glutathione and H2O2, can also lead the decrease of A652 nm due to their redox reaction with δ-MnO2 (Fig. S2†). However, they are unlikely existed in wastewater in aerobic conditions. Therefore, they may not interfere the practical catechol determination. The reproducibility of δ-MnO2-TMB was also examined by randomly selecting two catechol concentrations to carry out multiple measurements (n = 8). The relative standard deviations (RSDs) for the determination of 5 μM and 10 μM catechol was 4.96% and 4.42%, respectively. Moreover, the application of δ-MnO2-TMB for catechol determination in real water samples was also attempted. The recovery ratios of catechol in tap water, reuse water and river water are shown in Table 2, with small RSD values (less than 10.00%) and good recovery (94.80% to 99.56%).
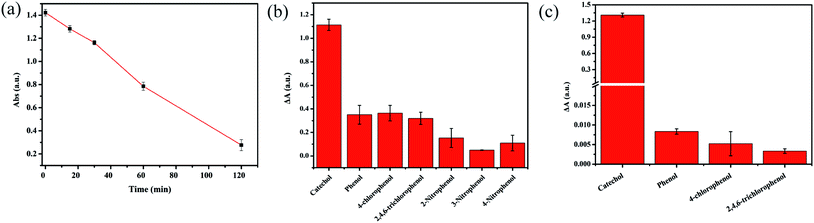 |
| Fig. 3 (a) The effect of incubation time of catechol and δ-MnO2 (b) detection selectivity of δ-MnO2-TMB to different phenolic compounds at pH 3.73 (c) detection selectivity of δ-MnO2-TMB to different phenolic compounds at pH 6.00. | |
Table 2 Determination of catechol in real water samples using δ-MnO2-TMB
Water sample |
Catechol added (μM) |
Catechol found (μM) |
RSD (%) |
Recovery (%) |
Tap water |
4.00 |
3.85 |
5.62 |
96.38 |
10.00 |
9.85 |
3.81 |
98.50 |
Reuse water |
4.00 |
3.79 |
9.96 |
94.80 |
10.00 |
9.96 |
2.80 |
99.56 |
River water |
4.00 |
3.94 |
5.61 |
95.22 |
10.00 |
9.89 |
1.75 |
98.92 |
3.4 Determination mechanism
After confirming the excellent sensing performance of δ-MnO2-TMB to catechol, the sensing mechanism of this system was investigated. The effect of δ-MnO2 on catechol, i.e., oxidant or catalyst, is still contentious.19,28 As shown in Fig. 4a, 42.3% of the catechol was quickly removed within the first 5 min, and then a slower increase in removal proportion was observed from 5 to 120 min. The final catechol removal rate was 70%. If ascorbic acid was used as the reaction quencher instead of filtration, the catechol absorbed on the surface of δ-MnO2 was released again into the solution. By comparing these two removal curves, it can be inferred that pre-absorption of catechol is necessary for its reaction, and absorption is much faster than the reaction. For MnO2, approximately 8.9% of Mn(II) in MnO2 was leached during the incubation process, which indicated that a redox reaction occurred between catechol and MnO2. In other words, δ-MnO2 is an oxidant for catechol, not a mimic of catechol oxidase.29 This result is also supported by the XPS spectra and TEM results (Fig. 4b and S3†). The average oxidation state (AOS) of Mn was calculated based on the Mn 3s spectra.30 After reacting with catechol for 2 h, the AOS of Mn decreased from 3.67 to 3.14, indicating the Mn(IV) and Mn(III) in MnO2 were reduced to Mn(II). In general, the inhibition of TMB oxidase-like activity of MnO2 by different compounds (GSH and ascorbic acid) is due to the decomposition of MnO2, which decrease the amount of MnO2 significantly.13,31 However, the small amount of Mn(II) leaching determined by AAS means the mass loss of δ-MnO2 was less than 10%. Therefore, the redox reaction between catechol and δ-MnO2 should not be the main factor for the inactivation of TMB oxidase-like activity. Furthermore, the size-dependent activity of nanozymes (Au NPs and Fe3O4 NPs) has been reported, but not mentioned in MnO2-based nanozymes.32 Fig. 4c shows the DLS results of δ-MnO2 with different concentrations of catechol. In the absence of catechol, the hydrodynamic radius of MnO2 was approximately 272.6 ± 20 nm at pH 3.73. Adding 2 μM catechol can induce the aggregation of δ-MnO2 and increase the hydrodynamic radius of δ-MnO2 to 488.5 ± 35.67 nm. Adding 10 μM catechol increased the diameter of δ-MnO2 beyond 10 μm, thus greatly decreasing the TMB oxidase-like activity. As the TMB-δ-MnO2 showed higher catechol selectivity to the other phenolic substrates at pH 6.00, the hydrodynamic radius of δ-MnO2 after incubation with 10 μM catechol and p-chlorophenol was compared. It can be observed that adding catechol increased the particle size to above 10 μm, while adding the same concentration of p-chlorophenol showed slight effect on the hydrodynamic radius of δ-MnO2. Therefore, catechol-induced δ-MnO2 specific aggregation should be the key factor in facilitating the sensitive and selective catechol determination. The aggregation mechanism of MnO2 caused by organics has been reported previously.19 The organics first reacted with MnO2 and dissolved MnO2 to release Mn(II); then, part of the Mn(II) was absorbed on the surface of MnO2 and formed a complex with the organics. Catechol is a strong complex of Mn(II) and Mn(III). Its oxidation products by δ-MnO2, i.e., semiquinone and hydroxylated semiquinone, are also strong complexes of Mn(II) and Mn(III), and can form an organic coating on the surface of MnO2.33 These organic coatings can act as bridge molecules to induce MnO2 aggregation.
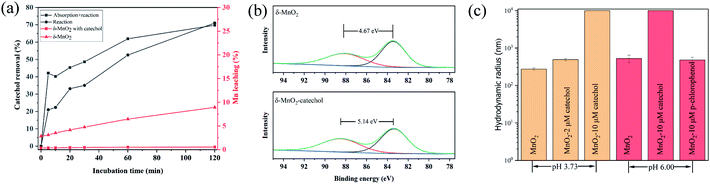 |
| Fig. 4 (a) Catechol removal and Mn(II) leaching profiles during the incubation process. The initial amount of MnO2 was 50 mg L−1, and the catechol was 150 μM (b) Mn 3s spectra of the pristine δ-MnO2 and catechol reacted δ-MnO2. (c) DLS results of pristine δ-MnO2, and δ-MnO2 treated with catechol and p-chlorophenol. | |
4. Conclusion
In conclusion, a TMB oxidase-like activity-based detection method for catechol was developed in this study. Catechol was first incubated with δ-MnO2 for 2 h to induce the δ-MnO2 aggregation, and then the aggregated δ-MnO2 reacted with TMB at a much lower rate than the well-dispersed δ-MnO2. This method has high sensitivity (with an LOD of 218 nM), and high selectivity (160-fold higher than those of other phenolic compounds) for catechol determination. It was also insensible to changes in ion strength and co-existing anions and cations. The only concern is the pH-dependent activity, which made a pre-adjustment of pH into 3.73 to 6.00 necessary for catechol determination in real water samples. Overall, the reduction-induced aggregation of a nanozyme was first used for the colorimetric determination of pollutants, which provides a new way to modulate and apply the activity of nanozymes.
Conflicts of interest
There are no conflicts to declare.
Acknowledgements
The authors were financially supported by the National Natural Science Foundation of China (Grant No. 41977197) and the Guangxi Major Project of Science and Technology (Grant No. AA17202032, AA18118013) from the Guangxi Science and Technology Department.
References
- W. Gernjak, T. Krutzler, A. Glaser, S. Malato, J. Caceres, R. Bauer and A. R. Fernández-Alba, Chemosphere, 2003, 50, 71–78 CrossRef CAS PubMed
. - N. Schweigert, A. J. Alexander and R. I. Eggen, Environ. Microbiol., 2001, 3, 81–91 CrossRef CAS PubMed
. - B. Bukowska and S. Kpwalska, Toxicol. Lett., 2004, 152, 73–84 CrossRef CAS PubMed
. - H. Du, J. Ye, J. Zhang, X. Huang and C. Yu, J. Electroanal. Chem., 2011, 650, 209–213 CrossRef CAS
. - S. Erogul, S. Z. Bas, M. Ozmen and S. Yildiz, Electrochim. Acta, 2015, 186, 302–313 CrossRef CAS
. - C. Bu, X. Liu, Y. Zhang, L. Li, X. Zhou and X. Lu, Colloids Surf., B, 2011, 88, 292–296 CrossRef CAS PubMed
. - Y. Kong, X. Chen, W. Wang and Z. Chen, Anal. Chim. Acta, 2011, 688, 203–207 CrossRef CAS PubMed
. - J. T. Han, K. J. Huang, J. Li, Y. M. Liu and M. Yu, Colloids Surf., B, 2012, 98, 58–62 CrossRef CAS
. - L. Gao, J. Zhuang, L. Nie, J. Zhang, Y. Zhang, N. Gu, T. Wang, J. Feng, D. Yang, S. Perrett and X. Yan, Nat. Nanotechnol., 2007, 2, 577 CrossRef CAS PubMed
. - H. Deng, S. He, X. Lin, L. Yang, Z. Lin, R. Chen, H. Peng and W. Chen, Chin. Chem. Lett., 2019, 30, 1659–1662 CrossRef CAS
. - M. Liu, Z. Li, Y. Li, J. Chen and Q. Yuan, Chin. Chem. Lett., 2019, 30, 1009–1012 CrossRef CAS
. - J. Zheng, D. Song, H. Chen, J. Xu, N. S. Alharbi, T. Hayat and M. Zhang, Chin. Chem. Lett., 2019 DOI:10.1016/j.cclet.2019.09.037
. - J. Liu, L. Meng, Z. Fei, P. J. Dyson, X. Jing and X. Liu, Biosens. Bioelectron., 2017, 90, 69–74 CrossRef CAS PubMed
. - X. Liu, Q. Wang, H. Zhao, L. Zhang, Y. Su and Y. Lv, Analyst, 2012, 137, 4552–4558 RSC
. - J. Ge, R. Cai, X. Chen, Q. Wu, L. Zhang, Y. Jiang, C. Cui, S. Wan and W. Tan, Talanta, 2019, 195, 40–45 CrossRef CAS PubMed
. - L. He, F. Wang, Y. Chen and Y. Liu, Luminescence, 2018, 33, 145–152 CrossRef CAS PubMed
. - A. Roy, R. Sahoo, C. Ray, S. Dutta and T. Pal, RSC Adv., 2016, 6, 32308–32318 RSC
. - L. Lin, D. Shi, Q. Li, G. Wang and X. Zhang, Anal. Methods, 2016, 8, 4119–4126 RSC
. - X. Huangfu, J. Jiang, J. Ma, Y. Wang, Y. Liu, X. Lu, X. Zhang and H. Cheng, Colloids Surf., A, 2015, 482, 485–490 CrossRef CAS
. - Y. Liu, H. Zhou, R. Cao, X. Liu, P. Zhang, J. Zhang and L. Liu, Appl. Catal., B, 2019, 245, 569–582 CrossRef CAS
. - C. K. Remucal and M. Ginder-Vogel, Environ. Sci.: Processes Impacts, 2014, 16, 1247–1266 RSC
. - M. Li, X. Huang and H. Yu, Mater. Sci. Eng., C, 2019, 101, 614–618 CrossRef CAS PubMed
. - R. Ai and Y. He, Sens. Actuators, B, 2020, 304, 127372 CrossRef
. - A. Asati, S. Santra, C. Kaittanis, S. Nath and J. M. Perez, Angew. Chem., Int. Ed. Engl., 2009, 48, 2308–2312 CrossRef CAS
. - L. P. Zhang, Y. P. Xing, L. H. Liu, X. H. Zhou and H. C. Shi, Sens. Actuators, B, 2016, 225, 593–599 CrossRef CAS
. - M. Lv, M. Wei, F. Rong, C. Terashima, A. Fujishima and Z. Z. Gu, Electroanalysis, 2010, 22, 199–203 CrossRef CAS
. - S. K. Patil, S. A. Patil, M. M. Vadiyar, D. V. Awale, A. S. Sartape, L. S. Walekar, G. B. Kolekar, U. V. Ghorpade, J. H. Kim and S. S. Kolekar, J. Mol. Liq., 2017, 244, 39–45 CrossRef CAS
. - A. Naidja and P. M. Huang, Surf. Sci., 2002, 506, 243–249 CrossRef
. - Y. Liu, H. Wu, Y. Chong, W. G. Wamer, Q. Xia, L. Cai, Z. Nie, P. Fu and J. Yin, ACS Appl. Mater. Interfaces, 2015, 7, 19709–19717 CrossRef CAS PubMed
. - J. Nie and H. Liu, J. Catal., 2014, 316, 57–66 CrossRef CAS
. - W. Huang, Y. Deng and Y. He, Biosens. Bioelectron., 2017, 91, 89–94 CrossRef CAS PubMed
. - M. Kalantari, T. Ghosh, Y. Liu, J. Zhang, J. Zou, C. Lei and C. Yu, ACS Appl. Mater. Interfaces, 2019, 11, 13264–13272 CrossRef CAS PubMed
. - A. Naidja, P. M. Huang and J. M. Bollag, Soil Sci. Soc. Am. J., 1998, 62, 188–195 CrossRef CAS
.
Footnote |
† Electronic supplementary information (ESI) available. See DOI: 10.1039/c9ra10480a |
|
This journal is © The Royal Society of Chemistry 2020 |
Click here to see how this site uses Cookies. View our privacy policy here.