DOI:
10.1039/C9RA10066K
(Paper)
RSC Adv., 2020,
10, 8074-8079
Synergistic anti-tumor efficacy by combination therapy of a self-assembled nanogel vaccine with an immune checkpoint anti-PD-1 antibody
Received
2nd December 2019
, Accepted 12th February 2020
First published on 25th February 2020
Abstract
Therapeutic strategies for cancer involving immune checkpoint inhibitors (ICIs) have been gaining widespread attention, but their efficacy remains limited. Thus, combination of ICI therapies with other therapeutic modalities may be required to improve their outcomes. In this study, we examined the improved efficacy of a CHP nanogel-based vaccine delivery system after combination with ICI therapy. For this, we evaluated the therapeutic efficacy of combining an anti-PD-1 antibody as an ICI with an OVA antigen-complexed CHP nanogel vaccine delivery system in a mouse E.G7-OVA tumor model. Mice were subcutaneously inoculated with E.G7-OVA tumor cells on one side of the back, and subcutaneously injected with OVA or the OVA/CHP nanogel vaccine on the other side of the back. Anti-PD-1 antibody was administered at defined intervals. Tumor volume, immune responses, and tumor-infiltrating cells were evaluated. Mice treated with OVA vaccine alone showed weak tumor suppression compared with untreated control mice. Mice receiving combined OVA/CHP nanogel vaccine and anti-PD-1 antibody therapy exhibited strong tumor growth suppression and markedly improved survival, suggesting that PD-1 signaling blockade by the anti-PD-1 antibody enhanced the anti-tumor efficacy of the OVA vaccine. Furthermore, tumor-infiltrating cells and immune responses were increased in the combined therapy group. No serious side effects were observed for any of the treatments. Taken together, the immune system activation induced by the CHP nanogel vaccine was synergistically enhanced by the anti-PD-1 antibody. The present findings suggest the potential for enhanced therapeutic efficacy by combining the CHP nanogel vaccine delivery system with ICI therapy for various cancer types.
1. Introduction
Immunotherapy has attracted attention as a new therapeutic strategy for cancer.1 Inhibitors of immune checkpoint molecules like PD-1/PD-L1 and CTLA-4 block immunosuppressive signaling pathways among T cells, cancer cells, and antigen-presenting cells, and consequently improve immunity.2,3 Although immune checkpoint inhibitor (ICI) therapy has shown impressive anti-tumor efficacy, the efficacy was dependent on individual patients or cancer types, because it was related to induction levels of cancer-specific cytotoxic T lymphocytes (CTLs) and expression levels of immune checkpoint molecules in tumors.4,5 Thus, despite the potential of ICI therapy for treatment of various cancers, its combination with other therapeutic modalities like immunotherapy, chemotherapy, or radiation therapy may be required for more effective outcomes.6–8
As one of the possible immunotherapies, vaccines specifically activate systemic immunity against antigens and have low side effects.9,10 In general, exogenous antigens induce Th2 immune system activation to exert cancer prophylactic effects. To activate the Th1 immune system and obtain cancer therapeutic effects, functional antigen delivery systems, such as delivery to lymph nodes, assistance of endosomal escape, and complexation with adjuvants, are needed.11–13 We previously developed a novel antigen delivery system involving a self-assembled nanogel.14,15 Specifically, cholesterol-bearing pullulan (CHP) self-assembly led to nanogel formation in water by hydrophobic interactions. The CHP nanogel acted as a protein delivery carrier, and showed immune-stealth ability by preventing interactions with non-antigen-presenting cells because of its highly hydrophilic surface.16 We further confirmed that the CHP nanogel induced Th1 immune system activation and exhibited anti-tumor efficacy as a cancer therapeutic vaccine,17,18 and CHP nanogel vaccines with HER-2 or NY-ESO-1 antigens have shown novel cancer therapeutic effect in clinical trials.19,20 The CHP nanogel effectively delivered antigens to lymph nodes and enabled activation of CTLs through cross-presentation of antigen-presenting cells including CD169+ macrophages. Thus, combination of the CHP nanogel vaccine with ICI therapy would be a promising strategy to enhance the anti-tumor efficacy for potential clinical applications. In the present study, we used an anti-PD-1 antibody as an ICI, and investigated its combination therapy with an OVA antigen-complexed CHP nanogel vaccine using an E.G7-OVA tumor model (Fig. 1).
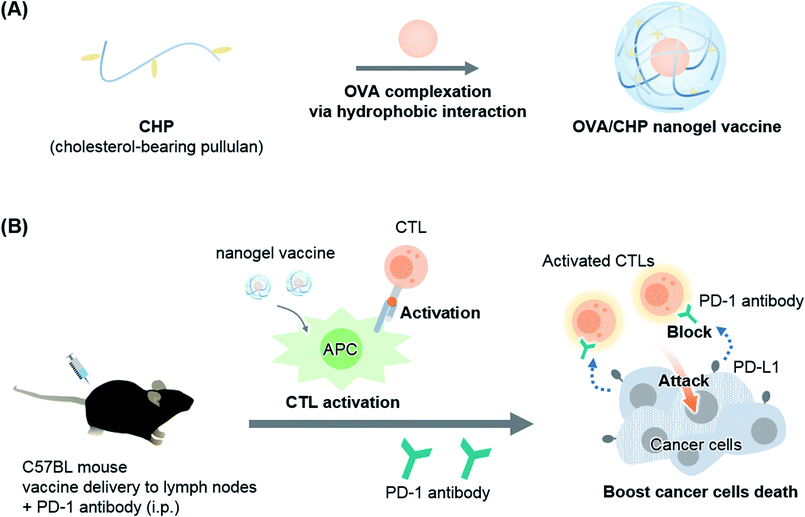 |
| Fig. 1 Combination therapy of OVA/CHP nanogel vaccine with anti-PD-1 antibody enhances the anti-tumor efficacy. A schematic illustration of the study is shown. (A) The CHP nanogel forms complexes with OVA protein via hydrophobic interactions between the cholesteryl group of CHP and hydrophobic amino acid residues of OVA. (B) The nanogel vaccine activates antigen-specific CTLs and the anti-PD-1 antibody enhances the anti-tumor efficacy by blockade of PD-1/PD-L1 signalling. | |
2. Materials and methods
2.1 Materials
CHP was purchased from NOF Corporation (Tokyo, Japan). Phosphate-buffered saline (PBS), ATCC-modified RPMI 1640 medium, fetal bovine serum (FBS), 2-mercaptoethanol, and antibiotic-antimycotic were purchased from Gibco (Carlsbad, CA, USA). G418 was purchased from Nacalai Tesque (Kyoto, Japan). EndoGrade OVA was purchased from Hyglos GmbH (Bernried, Germany). InVivoMAb anti-mouse PD-1 antibody (clone J43) was purchased from BioXCell (West Lebanon, NH, USA). Urea was purchased from Wako (Osaka, Japan). Slide-A-Lyzer™ Dialysis Cassettes (10 K MWCO, 3 mL) were purchased from Thermo Fisher Scientific (Waltham, MA, USA). IFN-γ Mouse Uncoated ELISA Kit was purchased from Invitrogen (Carlsbad, CA, USA). CpG with phosphorothioate modification was purchased from FASMAC (Kanagawa, Japan). PE-conjugated anti-mouse CD279 (PD-1) antibody (RMP1-30) and APC-conjugated anti-mouse CD8 antibody (53-6.7) were purchased from BioLegend (San Diego, CA, USA).
2.2 Cell culture and mice
E.G7-OVA cells were purchased from American Type Culture Collection (ATCC, Manassas, VA, USA), and cultured in ATCC-modified RPMI 1640 medium supplemented with 10% FBS, 0.4 mg mL−1 G418, 0.05 mM 2-mercaptoethanol, and 1% antibiotic-antimycotic. Female C57BL/6J mice were purchased from SLC Co. Japan (Shizuoka, Japan). All animal experiments were approved by the Ethics Committee for Animal Welfare of Kyoto University and were performed according to the institutional guidance of Kyoto University on animal experimentation.
2.3 Preparation of CHP nanogel vaccine
To obtain OVA/CHP complexes, CHP was dissolved in PBS containing 6 M urea, followed by addition of OVA (final concentrations: 5 mg mL−1 CHP nanogel and 0.2 mg mL−1 OVA in PBS containing 6 M urea). After complexation for 1 day at 37 °C, OVA/CHP complexes were dialyzed against PBS to remove urea. The OVA/CHP nanogel complex was passed through 0.22 μm filter for sterile. The complexes were kept at 4 °C until use. The diameters of CHP nanogel and OVA/CHP nanogel were measured by dynamic light scattering (DLS; Zetasizer Nano-ZS; Malvern, Worcestershire, UK).
2.4 Immunotherapy in tumor-bearing mice
For treatment with OVA/CHP nanogel vaccine, mice were inoculated with E.G7-OVA cells (1 × 106) in the backs of C57BL mice. For the combined treatment group, mice were treated with OVA or OVA/CHP vaccine (containing 20 μg OVA and 5 μg CpG) on days 6, 10, and 14 by subcutaneous administration, and anti-PD-1 antibody (200 μg) on days 8, 12, and 16 by intraperitoneal administration. For the anti-PD-1 antibody only group, mice were treated with anti-PD-1 antibody on days 6, 10, and 14 by intraperitoneal administration to start the treatment on the same day. As a control, one group was not take any treatment to monitor the natural growth of E.G7-OVA tumor. Tumor volumes were calculated by the formula: tumor volume = (major axis) × (minor axis) × (height) × π/6. Mice were euthanized for ethical reasons when the tumor volumes exceeded 1500 mm3. Each group contained 6–10 mice.
2.5 Immune activation
OVA or OVA/CHP nanogel vaccine (containing 20 μg OVA) plus CpG (5 μg) was subcutaneously injected into C57BL/6J mice to activate their immune system. Serum samples were collected on the following day for IFN-γ assays. CpG only was administered to control mice.
2.6 Evaluation of side effects
E.G7-OVA cells (1 × 106) were inoculated into C57BL/6J mice. Vaccines were subcutaneously injected on days 5 and 9, and anti-PD-1 antibody (200 μg) was intraperitoneally injected on days 7 and 11, followed by euthanasia on day 12. Serum was collected on day 12 for aspartate aminotransferase (AST) assays, which were performed by the customized services of Oriental Yeast Co. Ltd. (Nagahama, Japan). Spleens, lungs and tumors were harvested and weighed on day 12, and lungs were further weighed after drying. Tumors were minced in digestion buffer (RPMI 1640 medium containing 1 mg mL−1 collagenase type IV, 20 U mL−1 DNase IV, 10% FBS, and 1% antibiotics), and incubated at 37 °C for 1 h to obtain single-cell suspensions. The collected cells were immunostained with fluorescent dye-conjugated anti-CD8a and anti-PD-1 antibodies at 4 °C for 20 min. Tumor-infiltrating CD8+ cells and PD-1 expression levels in CD8+ cells were evaluated by flow cytometry (LSR Fortessa Cell Analyzer; BD Biosciences, San Jose, CA, USA). Data were analysed using FlowJo 7.6.5 software (Tree Star, Ashland, OR, USA).
3. Results and discussion
3.1 Anti-tumor efficacy against an E.G7-OVA tumor model
OVA/CHP nanogel complex was prepared by mixing denatured OVA and CHP nanogel. The diameters of CHP nanogel before and after OVA encapsulation were determined by DLS (Fig. 2). CHP nanogel and OVA/CHP nanogel complex showed 30 nm and 63 nm in diameter, respectively. The diameter of CHP nanogel became larger by OVA encapsulation, and kept suitable size for lymph node transfer (<100 nm).21 CHP nanogel enabled to form complex with OVA via hydrophobic interaction of cholesteryl group in CHP nanogel and hydrophobic domain in denatured OVA.
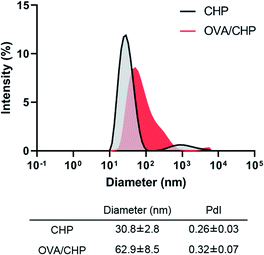 |
| Fig. 2 Characterization of OVA/CHP nanogel complex. Diameters of CHP nanogel before and after complexation with OVA were determined by DLS. | |
We investigated the anti-tumor efficacy of the combination therapy using an E.G7-OVA tumor model. C57BL/6J mice were inoculated E.G7-OVA tumor cells into the left side of the back, while vaccine was subcutaneously injected into the right side of the back to observe systemic immune activation. The anti-PD-1 antibody treatment group showed almost no tumor growth suppression efficacy and a similar tumor growth curve to the non-treatment group (Fig. 3A), indicating that vaccination with OVA antigen was necessary to induce sufficient CTLs into the tumor for treatment. The OVA group showed weak tumor growth suppression compared with non-vaccinated groups, but failed to exhibit tumor reduction. Meanwhile, the OVA/CHP nanogel vaccine group showed strong tumor reduction. As CHP nanogel enabled to deliver antigens into draining lymph nodes effectively,18 OVA/CHP nanogel vaccine would induce higher numbers of activated CTLs, which led efficient anti-tumor efficacy. The OVA with anti-PD-1 antibody group showed stronger anti-tumor efficacy than the OVA only group, suggesting that blockade of PD-1/PD-L1 signalling by the anti-PD-1 antibody enhanced the anti-tumor efficacy even in the OVA vaccine. Notably, the OVA/CHP nanogel vaccine with anti-PD-1 antibody combination therapy showed the strongest anti-tumor effect and the survival rate was dramatically improved (Fig. 3B). These results indicated that effective CTL activation by the CHP nanogel vaccine was essential for anti-tumor efficacy, and that the anti-PD-1 antibody combination therapy assisted the efficacy.
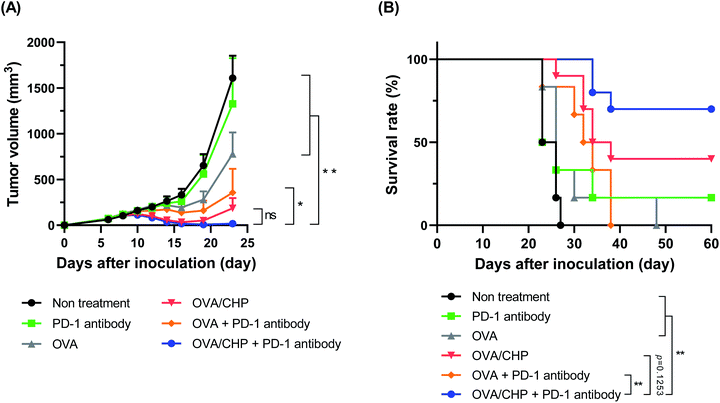 |
| Fig. 3 Anti-tumor efficacy of OVA/CHP nanogel vaccine with anti-PD-1 antibody. E.G7-OVA tumor-bearing mice (n = 6–8) were treated with vaccines and anti-PD-1 antibody. (A) E.G7-OVA tumor volumes in mice treated with anti-PD-1 antibody, OVA, OVA with anti-PD-1 antibody, OVA/CHP nanogel vaccine, or OVA/CHP nanogel vaccine with anti-PD-1 antibody. (B) Survival rates of the mice. Data are shown as means ± SEM. *p < 0.05, **p < 0.01, by one-way ANOVA (A) or log-rank (Mantel–Cox) test (B). | |
3.2 Effects on tumor environment by CHP nanogel vaccine and anti-PD-1 antibody treatment
To investigate activation of the tumor environment by the nanogel vaccine with anti-PD-1 antibody combination therapy, flow cytometric analysis was performed for E.G7-OVA tumors. Tumor-infiltrating CTLs (CD8+ cells) were increased when mice were vaccinated with OVA only or OVA/CHP nanogel vaccine (Fig. 4A). These findings indicated that the OVA/CHP nanogel vaccine activated more antigen-specific CTLs systemically and recruited these cells to tumor sites more effectively than the OVA vaccine. Moreover, infiltration of CTLs was slightly more effective with anti-PD-1 antibody administration.
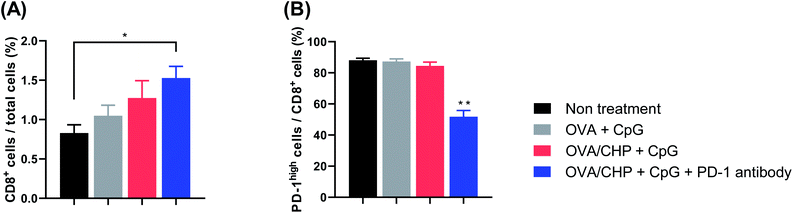 |
| Fig. 4 Effects on tumor-infiltrating T cells. E.G7-OVA tumor-bearing mice (n = 4) were treated with vaccines. (A and B) Ratios of tumor-infiltrating CTLs (CD8+ cells) (A) and PD-1 expression on CTLs (B) were evaluated by flow cytometry. Data are shown as means ± SEM. *p < 0.05, **p < 0.01, by one-way ANOVA. | |
High PD-1 expression in CTLs indicates T-cell exhaustion and loss of effector functions,22,23 and leads to weak tumor growth suppression and tumor regrowth. Almost all tumor-infiltrating CTLs showed high PD-1 expression after vaccination without anti-PD-1 antibody treatment, but the expression was down-regulated by vaccination plus anti-PD-1 antibody treatment (Fig. 4B). These results suggest that combination therapy of CHP nanogel vaccine and anti-PD-1 antibody recruited more CTLs into tumors and prevented T-cell exhaustion by inhibiting PD-1/PD-L1 signalling, thereby exhibiting marked anti-tumor efficacy.
3.3 Effects of CHP nanogel vaccine and anti-PD-1 antibody combination therapy on immune responses
We further evaluated the immune responses induced by nanogel vaccine with anti-PD-1 antibody combination therapy. CHP nanogel vaccine or OVA with CpG adjuvant were subcutaneously injected into mice, and serum IFN-γ levels were evaluated after 1 day (Fig. 5). IFN-γ was not detected in the CpG only group, because immune responses like production of inflammatory cytokines by CpG are very quick (within several hours) and the concentration would immediately decrease.24,25 Meanwhile, the serum IFN-γ level was increased after OVA or CHP nanogel vaccine treatment, suggesting that responses other than those induced by CpG had occurred. IFN-γ is an inflammatory cytokine that assists Th1 type immune system activation and leads to induction of CTL activation. Thus, the results indicated that treatment with the vaccines, especially the CHP nanogel vaccine, enabled activation of Th1 immunity.
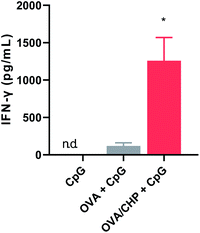 |
| Fig. 5 Immune system activation by OVA/CHP nanogel vaccine. At 24 h after subcutaneous injection of OVA or OVA/CHP nanogel vaccine into mice (n = 4), serum concentration of IFN-γ were measured by ELISA. Data are shown as means ± SEM. *p < 0.05, by one-way ANOVA. | |
Finally, the side effects of the treatments were evaluated. No splenomegaly or increased water content in the lung was observed after any of the treatments (Fig. 6A and B). The OVA/CHP nanogel vaccine and anti-PD-1 antibody combination therapy appeared to slightly increase the serum AST level, but the differences between the treatment groups were not significant (Fig. 6C). An increase in AST is a known side effect of nivolumab (anti-PD-1 antibody), and thus the AST level increment could be an effect of the anti-PD-1 antibody. Moreover, body weight did not decrease during the treatment schedule (Fig. 6D). Thus, there were no apparent serious side effects for the nanogel vaccine and the combination therapy with the anti-PD-1 antibody. While there were no differences in the tumor burdens on the mice, tumor weights were decreased by OVA/CHP nanogel vaccine administration (Fig. 6E), suggesting that antigen-specific Th1 immunity was effectively activated by the nanogel vaccine.
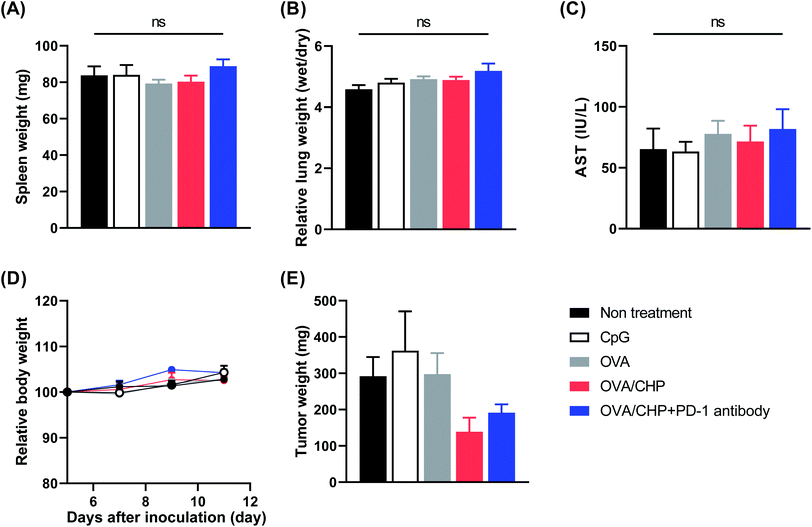 |
| Fig. 6 No side effects by OVA/CHP nanogel vaccine and the combination therapy with anti-PD-1 antibody. E.G7-OVA tumor-bearing mice (n = 4) were treated with vaccines and anti-PD-1 antibody, and measured for their spleen weight (A), lung water content (B), and serum AST level (C) as side effects. (D and E) Mice were monitored for their body weight during treatment (D) and tumor weight (E). Data are shown as means ± SEM. | |
4. Conclusions
We have utilized CHP nanogel as an antigen carrier for a cancer therapeutic vaccine. The CHP nanogel formed complexes with OVA protein by hydrophobic interactions, and enabled activation of the immune system. While anti-PD-1 antibody, OVA, or OVA with anti-PD-1 antibody treatments did not show anti-tumor efficacy, OVA/CHP nanogel vaccine combined with anti-PD-1 antibody showed tumor reduction. In particular, the combination therapy of OVA/CHP nanogel vaccine and anti-PD-1 antibody dramatically improved the survival rate compared with the other treatments without remarkable side effects.
The CHP nanogel formed stable complexes with protein antigens and activated the antigen-specific immune system, suggesting that the CHP nanogel vaccine system is applicable to various types of antigens. The anti-PD-1 antibody synergistically enhanced the immune activation induced by the CHP nanogel vaccine. In conclusion, the combination therapy of CHP nanogel vaccine with ICI therapy focuses on clinical applications and shows promising potential for cancer therapy.
Conflicts of interest
There are no conflicts to declare.
Acknowledgements
This work was supported by Grant-in-Aid for JSPS Fellows Grant number 16H063131 (K. A.) and 17J09905 (R. M.). The authors greatly appreciate Professor Hiroshi Shiku from Mie University, Japan and Dr Naozumi Harada from United Immunity, Japan for his helpful discussion.
References
- I. Mellman, G. Coukos and G. Dranoff, Nature, 2011, 480, 480–489 CrossRef CAS PubMed.
- P. Darvin, S. M. Toor, V. Sasidharan Nair and E. Elkord, Exp. Mol. Med., 2018, 50, 165 CrossRef PubMed.
- S. A. Patel and A. J. Minn, Immunity, 2018, 48, 417–433 CrossRef CAS PubMed.
- J. Galon and D. Bruni, Nat. Rev. Drug Discovery, 2019, 18, 197–218 CrossRef CAS PubMed.
- P. Bonaventura, T. Shekarian, V. Alcazer, J. Valladeau-Guilemond, S. Valsesia-Wittmann, S. Amigorena, C. Caux and S. Depil, Front. Immunol., 2019, 10, 168 CrossRef CAS PubMed.
- K. D. Moynihan, C. F. Opel, G. L. Szeto, A. Tzeng, E. F. Zhu, J. M. Engreitz, R. T. Williams, K. Rakhra, M. H. Zhang, A. M. Rothschilds, S. Kumari, R. L. Kelly, B. H. Kwan, W. Abraham, K. Hu, N. K. Mehta, M. J. Kauke, H. Suh, J. R. Cochran, D. A. Lauffenburger, K. D. Wittrup and D. J. Irvine, Nat. Med., 2016, 22, 1402–1410 CrossRef CAS PubMed.
- D. Schmid, C. G. Park, C. A. Hartl, N. Subedi, A. N. Cartwright, R. B. Puerto, Y. Zheng, J. Maiarana, G. J. Freeman, K. W. Wucherpfennig, D. J. Irvine and M. S. Goldberg, Nat. Commun., 2017, 8, 1747 CrossRef PubMed.
- W. Yue, L. Chen, L. Yu, B. Zhou, H. Yin, W. Ren, C. Liu, L. Guo, Y. Zhang, L. Sun, K. Zhang, H. Xu and Y. Chen, Nat. Commun., 2019, 10, 2025 CrossRef PubMed.
- S. H. Van Der Burg, R. Arens, F. Ossendorp, T. Van Hall and C. J. M. Melief, Nat. Rev. Cancer, 2016, 16, 219–233 CrossRef CAS PubMed.
- Y. Fan and J. Moon, Vaccines, 2015, 3, 662–685 CrossRef CAS PubMed.
- D. J. Irvine, M. A. Swartz and G. L. Szeto, Nat. Mater., 2013, 12, 978–990 CrossRef CAS PubMed.
- P. Sahdev, L. J. Ochyl and J. J. Moon, Pharm. Res., 2014, 31, 2563–2582 CrossRef CAS PubMed.
- Y. Mi, C. T. Hagan, B. G. Vincent and A. Z. Wang, Adv. Sci., 2019, 6, 1801847 CrossRef PubMed.
- K. Akiyoshi, S. Deguchi, H. Tajima, T. Nishikawa and J. Sunamoto, Macromolecules, 1997, 30, 857–861 CrossRef CAS.
- Y. Tahara and K. Akiyoshi, Adv. Drug Delivery Rev., 2015, 95, 65–76 CrossRef CAS PubMed.
- D. Muraoka, N. Seo, T. Hayashi, Y. Tahara, K. Fujii, I. Tawara, Y. Miyahara, K. Okamori, H. Yagita, S. Imoto, R. Yamaguchi, M. Komura, S. Miyano, M. Goto, S. I. Sawada, A. Asai, H. Ikeda, K. Akiyoshi, N. Harada and H. Shiku, J. Clin. Invest., 2019, 129, 1278–1294 CrossRef PubMed.
- D. Muraoka, N. Harada, T. Hayashi, Y. Tahara, F. Momose, S. I. Sawada, S. A. Mukai, K. Akiyoshi and H. Shiku, ACS Nano, 2014, 8, 9209–9218 CrossRef CAS PubMed.
- R. Miura, Y. Tahara, S. Sawada, Y. Sasaki and K. Akiyoshi, Sci. Rep., 2018, 8, 16464 CrossRef PubMed.
- S. Kitano, S. Kageyama, Y. Nagata, Y. Miyahara, A. Hiasa, H. Naota, S. Okumura, H. Imai, T. Shiraishi, M. Masuya, M. Nishikawa, J. Sunamoto, K. Akiyoshi, T. Kanematsu, A. M. Scott, R. Murphy, E. W. Hoffman, L. J. Old and H. Shiku, Clin. Cancer Res., 2006, 12, 7397–7405 CrossRef CAS PubMed.
- A. Uenaka, H. Wada, M. Isobe, T. Saika, K. Tsuji, E. Sato, S. Sato, Y. Noguchi, R. Kawabata, T. Yasuda, Y. Doki, H. Kumon, K. Iwatsuki, H. Shiku, M. Monden, A. A. Jungbluth, G. Ritter, R. Murphy, E. Hoffman, L. J. Old and E. Nakayama, Canc. Immun., 2007, 7, 9 Search PubMed.
- M. F. Bachmann and G. T. Jennings, Nat. Rev. Immunol., 2010, 10, 787–796 CrossRef CAS PubMed.
- E. J. Wherry, Nat. Immunol., 2011, 12, 492–499 CrossRef CAS PubMed.
- D. E. Speiser, D. T. Utzschneider, S. G. Oberle, C. Münz, P. Romero and D. Zehn, Nat. Rev. Immunol., 2014, 14, 768–774 CrossRef CAS PubMed.
- S. Hervas-Stubbs, A. Olivier, F. Boisgerault, N. Thieblemont and C. Leclerc, Blood, 2007, 109, 5318–5326 CrossRef CAS PubMed.
- X. Hou, X. Hao, M. Zheng, C. Xu, J. Wang, R. Zhou and Z. Tian, Cell. Mol. Immunol., 2017, 14, 675–684 CrossRef CAS PubMed.
|
This journal is © The Royal Society of Chemistry 2020 |