DOI:
10.1039/C9RA09380J
(Paper)
RSC Adv., 2020,
10, 3438-3449
An L-cystine/L-cysteine impregnated nanofiltration membrane with the superior performance of an anchoring heavy metal in wastewater†
Received
11th November 2019
, Accepted 5th January 2020
First published on 21st January 2020
Abstract
Considerable efforts are being made to develop new materials and technologies for the efficient and fast removal of toxic ions in drinking water. In this work, we developed a sulfur-complexed strategy to enhance the removal capability of heavy metal ions using the polyamide nanofiltration membrane by the covalent anchoring of L-cystine and L-cysteine. The sulfur-functionalized polyamide nanofiltration membrane exhibits superior complexation of heavy metal ions and can efficiently remove them from high-concentration wastewater. As a result, the sulfur-functionalized nanofiltration membrane not only showed excellent desalination performance but also achieved a record removal rate of heavy metal ions (99.99%), which can effectively reduce Hg(II) concentration from 10 ppm to an extremely low level of 0.18 ppb, well below the acceptable limits in drinking water (2 ppb). Moreover, the sulfur-functionalized nanofiltration membrane showed an exciting long-term stability and can be easily regenerated without significant loss of Hg(II) removal efficiency even after six cycles. Such outstanding performances were attributed to the synthetic effect of Hg–S coordinative interaction, electrostatic repulsion, and the sieving action of nanopores. These results highlight the tremendous potential of thiol/disulfide-functionalized NF active layer as an appealing platform for removing heavy metal ions from polluted water with high performance in environmental remediation.
1. Introduction
In recent years, water contamination and scarcity have evolved to be a global challenge owing to the rapid growth in population and industrialization.1–3 One of the major contaminants in drinking and industrial wastewater are heavy metals ions; they are highly toxic and difficult to decompose or metabolize in organisms.4,5 These heavy-metal ions continuously accumulate to exceed the permissible limits, which vastly threatens public health.6 In particular, Hg(II) is considered to be the most dangerous heavy metal ion widespread in the environment and it affects the brain, kidney, and lung tissues, severely impairing neurological development and even causing death.7,8 Therefore, there is a critical need to remove heavy metal ions from polluted water to maintain human and environmental well-being.9–11
Among the methods of heavy metal remediation including chemical precipitation,12 membrane separation, ion exchange,13 and adsorption,14–16 membrane separation is the most popularly used due to the advantages of smaller footprint, low-cost, elimination of chemical residuals, relative ease of scaling up, and environmental friendliness.17–19 As one of the effective means of membrane process, the nanofiltration (NF) technique, which combines the separation mechanisms of size exclusion and Donnan exclusion, has being widely used in drinking water and wastewater treatments because of the higher energy efficiency and simplicity of operation. Currently, the state of the art NF membrane for desalination is composed of a thin film composite (TFC) structure by growing polyamide (PA) nanofilm active layer on a support material,20 and the permselectivity performance is highly dependent on the molecular and structural characteristics of the PA layer. Since the last few years, researchers have devoted numerous efforts on the modification of TFC nanofiltration separation layer for enhancing the heavy metal removal efficiency. For instance, Chung et al.21 realized higher than 98% rejections of different heavy metals including Pb(II), Cu(II), Ni(II), Cd(II), Zn(II), Cr(VI), and As(V) by employing the chelating polymer modified P84 NF hollow fiber membrane as a separation layer. The poly(amidoamine) dendrimer (PAMAM) was grafted on TFC NF hollow fiber membranes to enhance the heavy metal removal efficiency and achieve an ultrahigh rejection of 99%.22 In addition, graphene-based materials such as graphene oxide (GO) was also used to improve the heavy metal removal properties of the TFC NF separation layer because of the high porosity, well-defined nanoscale pore size, and suitable mechanical stability.23,24 Although water permeability is greatly enhanced after the decoration of GO-based frameworks, the undesirable rejection of small ionic species cannot meet the requirement of practical application.25–27 Currently, the difficulty of constructing the NF membrane mainly lies in enhancing the binding affinity for the target toxic ions to simultaneously achieve the outstanding performance of removal efficiency, selectivity, and reusability. At the same time, the specific and selective elimination of Hg(II) ions from wastewater using separation membranes has scarcely been developed. In this regard, exploring a novel NF membrane material with high efficiency and selectivity for heavy metal removal is mandatory and has been pursued for long.
As a family of soft Lewis bases, sulfur-containing species own a highly polarizable donor center, enabling it to strongly coordinate with low-lying orbitals of soft Lewis acids such as heavy metal ions according to Pearson's theory of Hard/Soft Acids and Bases (HSAB).28–35 In this context, numerous organic sulfur compounds including thiols, thioethers, disulfides, and mercaptans have been adopted to prepare new materials with high removal efficiency of heavy metal ions such as Hg(II), Ag(I), Pb(II), and Cd(II).36,37 For example, some thiol/thio-functionalized covalent organic frameworks (COFs), metal–organic frameworks (MOFs), carbon nanotubes, mesoporous silicas, zeolites as well as porous carbons have been developed38–46 and exhibit high efficiency, selectivity, and easy recyclable adsorption capability towards toxic heavy metal ions. Nevertheless, several drawbacks of these adsorbents are needed to be improved, such as time consumption, production of large amounts of toxic sludge and liquid waste as well as high operational and capital costs.22 These weaknesses and barriers have sparked our interest in the exploration of new types of materials functionalized with sulfur ligands for water purification. Given all of this, the TFC NF membrane incorporated with sulfur-containing species may be a potential candidate for mitigating environmental problems caused by toxic heavy metals. However, no sulfur-containing species functionalized NF membranes have been reported for effective heavy metal removal from aqueous environments.
Thus, the objective of the present work is to prepare TFC NF active layers functionalized with disulfide (–SS–) and thiol (–SH) groups that are expected to exhibit specific binding ability towards the targeted heavy metals. The surface-bound sulfur ligands enable strong metal-sulfur coordinative interactions, thereby affording efficient and selective removal of toxic heavy metals. Characterizations were conducted to understand the evolution of surface chemistry, morphology, and physicochemical properties of the membranes before and after modification. Heavy metal ions, including Hg(II), Pb(II), Ni(II), Cu(II), Ag(II), and Cd(II), were also used to test the performance of the pristine and modified membranes. The resultant sulfur-impregnated NF active layers combined exceptional selectivity, good long-term stability, easy regeneration, and unprecedentedly high removal rate of Hg(II) ions (99.99%), making it a highly promising material for decontamination of drinking water. To the best of our knowledge, this is the first attempt towards the use of sulfur-impregnated membrane materials for heavy metal removal studies. This study may open up a new perspective to design advanced TFC NF membrane materials for heavy metal ion removal.
2. Experimental
2.1 Materials
1,3,5-Benzenetricarboxylic chloride (TMC, 98%) and anhydrous piperazine (PIP, 99%) were purchased from Alfa. L-cystine (99%), L-cysteine (99%), AgNO3 (99%), Pb(NO3)2 (99%), and NiCl2·6H2O (99%) were obtained from Adamas-beta. Hg(NO3)2 (99%) was received from Xiya Reagent. n-hexane, Cd(NO3)2·4H2O, CuCl2·2H2O, NaOH, HCl (36.0–38.0%), Na2SO4, MgSO4, NaCl, and CaCl2 were bought from Kelong Chemical Reagent Factory (Chengdu, China). All reagents were directly used without further purification. Flat-sheet non-woven-reinforced polysulfone (PSf) ultrafiltration membrane with a molecular weight cut-off of 60
000 as the support was provided by Beijing Haiqingyuan Tech. Co. Ltd and deionized water (3 μS cm−1) was obtained from an ultrapure water treatment system.
2.2 Preparation of the membrane
The sulfur-impregnated NF active layers were prepared via a typical two-step interfacial polymerization (IP) reaction (as illustrated in Fig. 1). Specifically, PIP aqueous solution (0.35 wt%) was firstly poured onto the top surface of porous PSf support. After being immersed for 2 min, the excess PIP solution was decanted and the PSf support was air-dried for further reaction. Then, 0.3 wt% TMC hexane solution was rapidly poured on the soaked support for interfacial polymerization. After 1 min, the residual n-hexane was removed to terminate the reaction and the freshly prepared PA layer is defined as PIP-TMC. Immediately after this step, an aqueous phase containing L-cystine (1) or L-cysteine (2) was separately poured onto the fresh PIP-TMC surface to react with the residual acyl chloride functional groups. After allowing to react for 2 min, the excess solution was drained off the surface, followed by drying in air for 3 min, then in an oven at 50 °C for 15 min; a sulfur-impregnated PA active layer was obtained. Finally, the fabricated membrane was rinsed with deionized water and stored in NaHSO3 (1 wt%, pH = 12) aqueous solution for performance characterization. The obtained membranes are denoted as 1 or 2-X, where X corresponds to the addition of monomers. Also, 1-0.1 and 2-0.1 are named as NF-SS and NF-SH, respectively. The TFC NF membranes that refer to 3-1 (L-cystine grafted) and 3-2 (L-cysteine grafted) were prepared by the one-step IP technique for comparison with those made by the two-step route. The one-step procedure started with immersion of the PSf support into a mixed aqueous phase containing PIP (0.35 wt%) and L-cystine or L-cysteine (0.1 g) for 2 min and subsequent removal of the excess liquid. Then, the membrane was immersed into a TMC (0.3 wt%) solution in hexane for 1 min and subjected to the same treatment as mentioned above.
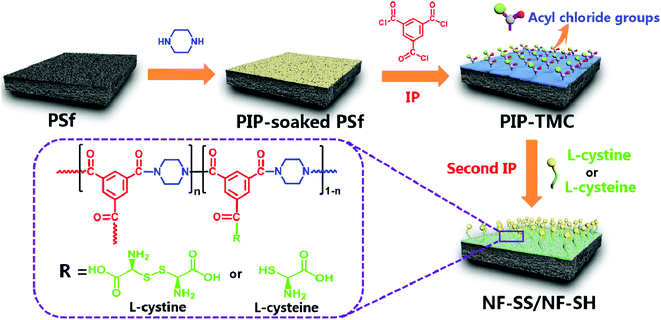 |
| Fig. 1 Schematic representation of the fabrication of sulfur-impregnated NF active layers. | |
2.3 Membrane characterization
All the membranes were washed with deionized water and dried in a vacuum oven before material characterization. The chemical component of NF selective layers was determined by using a Fourier transform infrared/attenuated total reflectance spectrometer (FT-IR/ATR, Nicolet 380) with an attenuated total reflectance (ATR) unit and an X-ray photoelectron spectrometer (XPS, Kratos, England). The surface and cross-sectional morphologies of the NF layers were investigated by field-emission scanning electron microscopy (FE-SEM, NOVA NANO SEM 450, FEI, USA), atomic force microscopy (AFM, Asylum Research MPD-3d, USA), and transmission electron microscopy (TEM, FEI Tecnai G2 F20, USA). The samples for FE-SEM cross-sectional images were obtained by fracturing the membrane in liquid nitrogen. The surface root-mean-square roughness (RMS) was estimated through the three-dimensional AFM images (5 μm × 5 μm) operated in the tapping mode. To obtain the cross-sections TEM images, the woven fabric substrate (polyester) was carefully removed from the membranes and the NF active layer was embedded with the epoxy resin, then cut at room temperature to obtain the cross-section. Water contact angles (CAs) were measured with a goniometer (DSA30 drop shape analysis system, KRUSS) under sessile drop mode at 25 °C; at least 7 different locations on each sample were tested. Nitrogen-sorption isotherms were obtained from a Quantachrome ASiQwin Instrument. Before the adsorption measurements, the samples were degassed in vacuum at 80 °C for 24 h. The zeta potential of the membrane surface was determined with an electrokinetic analyzer (SurPASS Anton Paar, GmbH, Austria); 1 mM KCl solution served as the background electrolyte and a pH range of 3–10 was adopted.
2.4 Membrane performance test
Water flux and rejection performance of the NF membranes were examined by using a home-made cross-flow filtration instrument with an effective cell area of 60 cm2, and the operating pressure and temperature were set as 6.0 bar and 25 °C, respectively. 2000 ppm of Na2SO4, MgSO4, NaCl, and CaCl2 solutions were used as feed solutions to evaluate the desalination performance. The electrical conductivity of the permeate and feed solutions were measured with a DDS-307 conductivity meter (Shanghai INESA & Scientific Instrument Co. Ltd, China); a calibration curve was used to relate the solution conductivity to the salt concentration. 10 ppm (Hg(NO3)2, Pb(NO3)2, NiCl2·6H2O, CuCl2·2H2O, AgNO3, and Cd(NO3)2·4H2O) solutions were filtered through the separation layers to estimate the heavy metal ion removal property, and the concentrations of the feed and the permeate were obtained by using atomic absorption spectrophotometry (AAS, VARIAN, SpectrAA 220FS, SpectrAA 220Z) and inductively coupled plasma-mass spectrometry (ICP-MS, VG PQ ExCell, TJA, USA). Experimental data were collected after 1 h-filtration to ensure steady-state flow conditions. The water flux of the NF membranes (J, L m−2 h−1) was calculated with eqn (1) and the rejection or removal rate (R, %) was obtained with eqn (2): |
 | (1) |
|
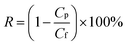 | (2) |
where V (L) is the volume of the collected permeate, Δt (h) is the time elapsed for collecting a required permeate volume, A (m2) is the superficial area of the membrane, and Cf and Cp represent the solute concentration of the feed solution and the permeate solution, respectively.
3. Results and discussion
3.1 Membrane surface chemical composition
The fabrication process of the sulfur-impregnated NF active layers is schematically illustrated in Fig. 1. The TFC NF active layer was prepared by creating a polyamide selective layer on the porous PSf support via a two-step IP reaction. The surface chemical components of the TFC NF active layer were identified by FT-IR/ATR and XPS techniques. Fig. S1† presents the FT-IR/ATR spectra of the NF membranes. It was found that there appeared a new peak at about 1620 cm−1 on the polyamide membrane surface. This peak is ascribed to the stretching vibration of C
O in the amide groups, which reveals that interfacial reaction had successfully occurred and a polyamide active layer was formed on the surface of the PSf support. What should be pointed out here is that we had difficulty in determining the differences between the FT-ATR spectra of PIP-TMC and the sulfur-impregnated active layers. This is probably because the S–H (2500 cm−1), C–S (700–600 cm−1), and S–S (500 cm−1) stretching bands are weak and their peak overlaps with the absorption of other already existing stretching vibrations. The predominant peaks at 285.0 eV, 400.0 eV, and 532.0 eV are facilely assigned to C 1s, N 1s, and O 1s, respectively (Fig. 2a).47 As evidenced in Fig. 2 and S2,† two eminent peaks of S 2s (231.6 eV) and S 2p (163.0 eV) emerged in the active layers of NF-SS and NF-SH, revealing the existence of the sulfur species.48 Furthermore, the high-resolution XPS spectra could provide more detailed information on the chemical composition of the NF active layers and thus, the peaks of C 1s, N 1s, and S 2p were deconvoluted into several characteristic peaks under consideration of the elements and the groups directly bonded to the atoms. The C 1s peaks at binding energies of 284.2, 285.4 eV, and 287.6 are assigned to C
C/C–C/C–H, C–N, and N–C
O in PIP-TMC, respectively (Fig. 2b),49,50 while they are assigned to C
C/C–C/C–H/C–S, C–N, and N–C
O/O–C
O in NF-SS and NF-SH, respectively (Fig. 2d and g). Similarly, the peaks at 399.4 eV and 399.9 eV (Fig. 2c, e, and h) correspond to N–C
O and C–N in the N 1s spectra.51 The characteristic peaks of S 2p centered at 163.0 eV were detected, as Fig. 2f and i demonstrate, in which the two peaks were respectively assigned to S–C/S–S and S–C/S–H.52,53 These results signified that the reactant monomers L-cystine and L-cysteine containing –SS– and –SH functional groups have been successfully grafted on the pristine PIP-TMC membrane surface.
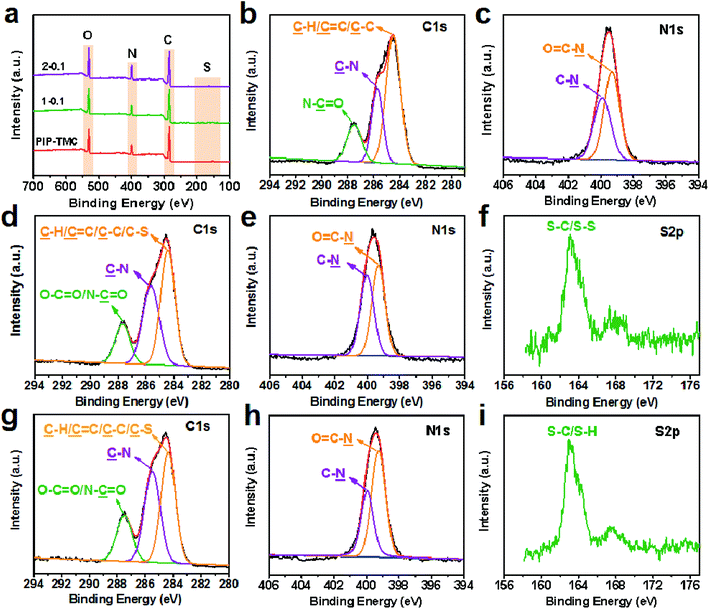 |
| Fig. 2 (a) XPS survey spectra of the NF active layers; high-resolution XPS analysis of (b) C 1s and (c) N 1s of the PIP-TMC active layer; high-resolution XPS analysis of (d) C 1s, (e) N 1s, and (f) S 2p core-level spectra of the NF-SS active layer; high-resolution XPS analysis of (g) C 1s, (h) N 1s, and (i) S 2p core-level spectra of the NF-SH active layer. | |
3.2 Membrane surface morphology and porosity
The surface morphology of the membranes was characterized by FE-SEM analysis. It could be observed that the nascent active layer shows a nodular surface accompanied by plenty of crumples (Fig. 3a).51 In comparison, a fairly uniform and obvious grainy surface was generated after the two-step interfacial polymerization, which might be attributed to the smooth surface of the NF-SS and NF-SH active layers. To gain insight into the changes in surface morphology, the surface roughness was examined by employing the AFM technique. A smoother surface could be obviously seen and the RMS values significantly decreased after the impregnation of sulfur species probably because of trampling of L-cystine and L-cysteine chains in the valley structures of the pristine PIP-TMC membrane (Fig. 3d–f), which agree well with the SEM results.54 At the same time, as Fig. S3† shows, the topography images of the membranes are also highly consistent with the corresponding SEM results. Therefore, the attachment of L-cystine and L-cysteine monomers onto the raw PA layer would not affect the depositions of foulants on the membrane surface caused by surface roughness,55 which plays a positive role in the antifouling performance of the resultant membrane. Moreover, the cross-sectional SEM and TEM images were also observed. It could be seen that the thickness of the bare NF active layer is about 200 nm, whereas that of the NF-SS and NF-SH layers significantly diminished to 119 nm and 164 nm, respectively (Fig. 4a–c). The reduction in the nanofilm thickness would greatly reduce the resistance to water permeation and thereby facilitate mass transport across the TFC NF barrier layer used for water/wastewater treatment. To gain insight into the porosity properties of the active layers, nitrogen gas sorption experiments were carried out. As evidenced by Fig. 5c, the pore size distribution (PSD) curves calculated by using the density functional theory (DFT) method gave a pore size of about 2.0 nm for the PIP-TMC, NF-SS, and NF-SH separation layer, which is characteristic of the NF membrane. The sorption curve at low relative pressures (P/P0 < 0.01) exhibited steep nitrogen uptakes (Fig. S5†), which is indicative of its microporous feature.56 Such a microporous structure would greatly influence the subsequent separation performance for inorganic salts and heavy metal ions.
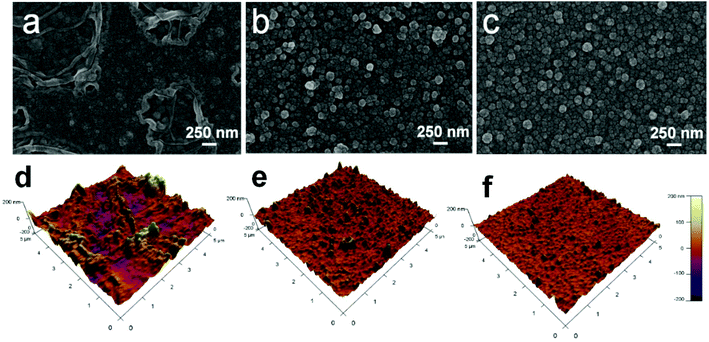 |
| Fig. 3 The surficial-sectional SEM and AFM images of (a and d) PIP-TMC, and (b and e) NF-SS, and (c and f) the NF-SH active layers. | |
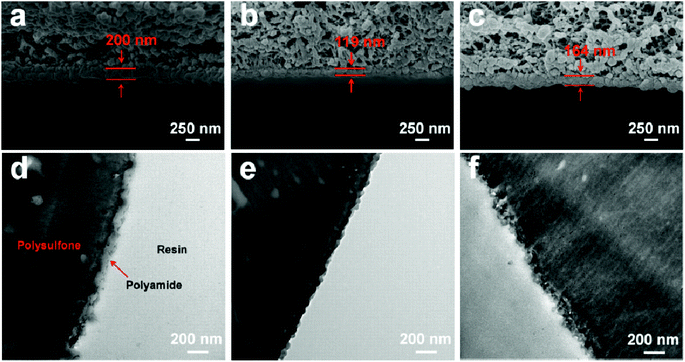 |
| Fig. 4 The cross-sectional SEM and TEM images of (a and d) PIP-TMC, and (b and e) NF-SS, and (c and f) NF-SH active layers. | |
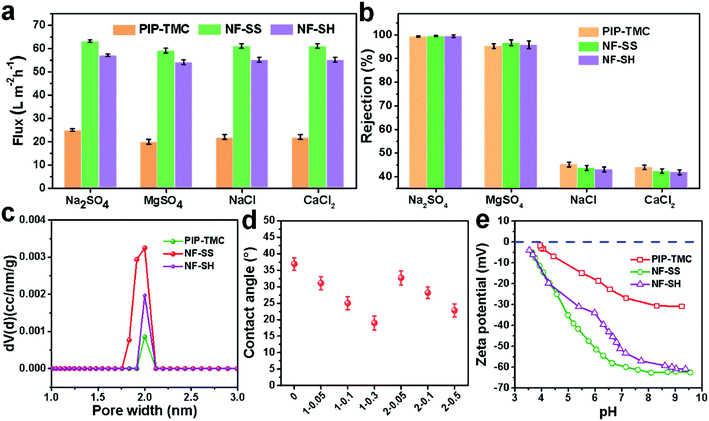 |
| Fig. 5 (a) Water fluxes and (b) rejections of PIP-TMC, NF-SS, and NF-SH active layers for different salt aqueous solution of 2000 ppm at 25 °C and 6 bar; (c) pore size distribution profiles of PIP-TMC, NF-SS, and NF-SH; (d) water contact angles of different NF samples; (e) zeta potentials of PIP-TMC, NF-SS, and NF-SH active layers with increasing pH values. | |
3.3 Permselectivity of inorganic salts
In order to evaluate the desalination performance of PIP-TMC, NF-SS, and NF-SH separation layer, the water flux and salt rejection of different membranes were measured with a home-made cross-flow filtration instrument. As Fig. 5a and b show, the desalination performance of NF-SS and NF-SH for all the investigated inorganic salts are significantly improved compared to the traditional PIP-TMC active layer, and achieve a 2.5-fold enhancement in water permeance and an ultrahigh salt rejection. Moreover, the NF-SS and NF-SH active layers display even higher rejections against multivalent anions (99.6%, 99.5% vs. 99.3% to Na2SO4, 96.7%, 95.5 vs.95.3% to MgSO4, 43.7%, 43.1% vs. 45.2% to NaCl, 42.4%,41.9% vs. 44.0% to CaCl2) (Fig. 5b). These results demonstrate that the grafting of L-cystine and L-cysteine on the PIP-TMC active layer accounts for the enhancement in desalination performance. We reasoned that the high desalting performance of NF-SS and NF-SH is attributed to the more hydrophilic and negative-charged surface. On one hand, the hydrophilic property was examined by comparing the water CAs of the surface before and after impregnation. It could be found that the CA of the NF-SS and NF-SH membranes declined to 25.0° and 28.2°, respectively (Fig. 5d), illustrating that the surface is more hydrophilic surface, thereby favoring the reduction in membrane fouling by hydrophobic foulants.57 On the other hand, based on the Donnan exclusion theory,58,59 strong electrostatic repulsion exists between multivalent anions (SO42−) and the membrane surface, which decisively influences the multivalent anions passing through the barrier layer of the membranes.60 It is believed that the surface charge character of the membrane material is closely correlated with the zeta potentials; we therefore measured the zeta potential data in the pH range of 3.0–10.0. Obviously, the NF-SS and NF-SH surfaces tethered L-cystine and L-cysteine monomers bearing an abundance of carboxyl groups have more negative charges than PIP-TMC (Fig. 5e), which thereby enhance the electrostatic repulsive effect between the membrane surface and multivalent anions (SO42−), thus endowing the separation with layer higher retention for multivalent anion salts. Taken together, the involvement of L-cystine and L-cysteine resulted in more hydrophilic and negatively charged active layer surfaces, which provide satisfactorily high water permeance while maintaining high selective capability for different inorganic salts.
3.4 Removal performances for heavy metal ions
The flux and removal rate for heavy metal ions of the as-prepared active layers were determined by using 10 ppm heavy metal ion solutions as seed solutions. As Fig. 6a and b illustrate, NF-SS and NF-SH can effectively eliminate toxic ions in water such as Hg(II), Pb(II), Ni(II), Cu(II), Ag(I), and Cd(II) and exhibit excellent water flux. To be specific, NF-SS shows a high removal rate of ∼99.98% for Hg(II) and can reduce the concentration of heavily mercury contaminated water down to 1.8 ppb, thus acquiring allowable elemental limits (2 ppb for Hg(II)) in drinking water, which is far beyond that provided by the bare PIP-TMC NF membrane (88.47%). At the same time, NF-SH achieved an unprecedentedly high Hg(II) removal rate of 99.99% and obtained an acceptable mercury level of 0.18 ppb, according to the international standards of U. S. Environmental Protection Agency (EPA) and World Health Organization (WHO).61 It is also worth mentioning that the sulfur-impregnated NF active layers display the highest removal rate of Hg(II) when compared to other selected heavy metal ions including Pb(II), Ni(II), Cu(II), Ag(I), and Cd(II), thus revealing an outstanding selectivity for different heavy metal ions. Furthermore, we compared Hg(II) ion removal performances of NF-SS and NF-SH with recently reported benchmark porous materials and other state-of-the-art thiol/thio-functionalized materials (ESI, ref. 1–12†).38–40,45,46 As demonstrated in Fig. 6d, it is can be observed that the Hg(II) removal performance of NF-SH is superior to those of most benchmark materials, such as porous carbon,46 mesoporous silica (ESI, ref. 6†),45 and MOF materials (ESI, ref. 1 and 2†).40 Although COF-S-SH38 and PAF-1-SH (ESI, ref. 8†) can reduce Hg(II) concentrations to 0.1 and 0.4 ppb, respectively, the ultralong equilibrium time (3–6 h) increases the operational and capital costs, thus precluding any implementation. Comparatively speaking, our newly designed NF active layers used for the purification process within a few minutes can reduce Hg(II) to acceptable concentrations. We surmise that such extraordinary performances are due to the covalent binding of L-cystine and L-cysteine with the –SS– and –SH functional groups in the bare TFC polyamide framework, which demonstrate a great promise for selective and efficient clean-up of heavy metal ions from drinking water.
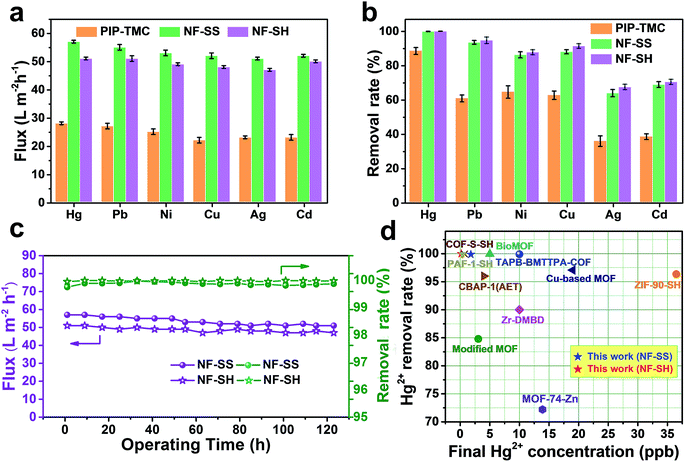 |
| Fig. 6 (a) Water flux and (b) removal rate of PIP-TMC, NF-SS, and NF-SH active layers for different heavy metal ions solutions of 10 ppm at 25 °C and 6 bar; (c) durability of NF-SS and NF-SH in a long-time NF operation process for the removal of Hg(II) ion; (d) comparison of Hg(II) removal performance of recently reported state-of-the-art TFC PA separation membranes and other benchmark thiol/thio-functionalized porous materials (see Table S1† for details). | |
From the perspective of ecological protection and economic sustainability, long-term cyclic stability of wastewater treatment materials is vital for its realistic application; hence, the demetallization and reuse of NF-SS and NF-SH were investigated. Remarkably, the NF-SS and NF-SH active layers still maintain high removal rate for Hg(II) ions over 120 h of continuous filtration, and no appreciable decline in the permeating flux was observed (Fig. 6c). Further, the Hg(II)-laden NF-SS and NF-SH membranes were easily regenerated by rinsing with an aqueous HCl solution (6.0 M), which resulted in almost 98% and 100% demetallation, respectively; the regenerated NF-SS and NF-SH were then subjected to the next round of Hg(II) decontamination processes. It is notable that NF-SS and NF-SH still retain about 91% and 96% of the original removal rate even after six regeneration and reuse cycles (Fig. 7b),51 suggesting the sulfur-impregnated NF membrane can work as a promising candidate as a long-term and effective heavy metal-scavenging material. The selectivity of the NF membrane in high concentration and multi-component wastewater is another critical challenge for practical applications. When the NF membrane is used for water treatment, the different ions will compete for binding sites and the inorganics may block the pores of the NF active layer, thereby compromising the removal efficiency.40 In light of this, selectivity tests were carried out by using a mixture solution of Hg(II), Pb(II), Cu(II), Ca(II), Mg(II), and Na(I), each at a concentration of 10 ppm, as the seed solution. The results show that NF-SS and NF-SH are capable of removing more than 90% of Hg(II), Pb(II), and Cu(II), and reduce them to drinkable concentrations but they are not active for other metal ions such as Ca(II), Mg(II), and Na(I), thus demonstrating the promising application of sulfur-impregnated NF active layers for the purification of high concentration multicomponent wastewater.
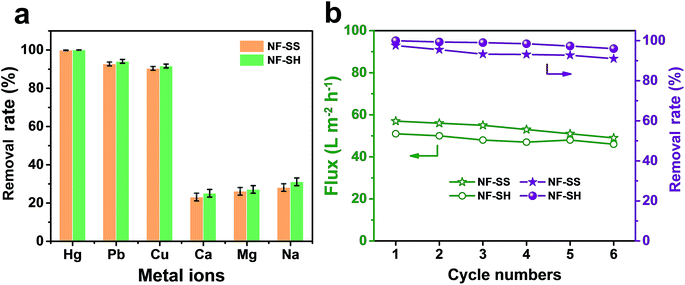 |
| Fig. 7 (a) The removal rate of NF-SS and NF-SH active layers for Hg(II) ion in the presence of other competing metal ions; (b) cycle performance of NF-SS and NF-SH active layers for Hg(II) ion removal. | |
Encouraged by the exceptional performances, we further explored the heavy metal ion removal mechanism of sulfur-impregnated NF active layers, as depicted in Fig. 8. First, –SS– and –SH functional groups are covalently tethered on the surface of the bare polyamide NF membrane. The highly accessible and densely populated sulfur species within the polymeric frameworks serve as available soft Lewis bases to bond the soft Lewis acids of heavy metal ions.28 Second, the hydrated heavy metal ions such as Hg(II) would approach the surface of the membrane quickly, immediately followed by the occurrence of metal-S chelating behavior, which is a spontaneous process propelled by strong and specific surface complexation interactions. As electron acceptors, the empty orbital of heavy metal ions such as Hg(II) could receive electrons from the electron donor of sulfur species to form stable coordination bonds.35,42 Specifically, each Hg was bound exclusively by two S, that is, Hg(II) ions are complexed in an S–Hg–S bidentate manner, as reported elsewhere.38,62–65 With respect to the other relatively soft metal ions, such as Pb(II), Cd(II), Ni(II), Cu(II), and Ag(I), due to their lower affinity towards sulfur species than Hg(II), the rejection performances are thereby inferior to that for Hg(II). This is because thiol and disulfide are soft bases groups and hence, they are more likely to attract and interact strongly with soft acids heavy metal ions; among them, Hg(II) is in fact classified as a softer metal than Pb(II) and Cd(II) because of its larger size and greater electron-cloud deformability.42,63,66–68 As such, the selective separation of Hg(II) from the mixed metal solutions could be well explained. It should also be noted that Hg(II) interaction with –SH groups in NF-SH is more favorable than that with –SS– groups of NF-SS probably due to the steric impediment, thus affording higher removal rate for Hg(II).62 On the other hand, there exist strong electrostatic repulsions among the multivalent cations (Hg2+) and the positively charged membrane surface contributed by the attachment of Hg(II) ions firstly. Here, the variation in the surface charges of NF-SS and NF-SH were investigated through measurements of the zeta potentials. Obviously, NF-SS and NF-SH surfaces exhibited more positive charge after Hg–S coordination (Fig. S8†), thereby greatly enhancing the electrostatic repulsion and increasing the transfer resistance. It is worth noting that the Hg2+ ions in aqueous solution were firmly anchored onto the surface of the membranes and could not drift around, so they would not fill the membrane pores, thereby maintaining high water permeance. Finally, the combination of size exclusion, Hg–S coordinative interactions, and electrostatic repulsion would bring selective and efficient elimination of Hg(II) from polluted water. This heavy metal-scavenging process is fast, highly effective, and the approach is relatively facile, cheap, and highly recyclable, therefore promising the sulfur-impregnated NF membrane with great potential applications in industrial drinking water treatment and environmental remediation.
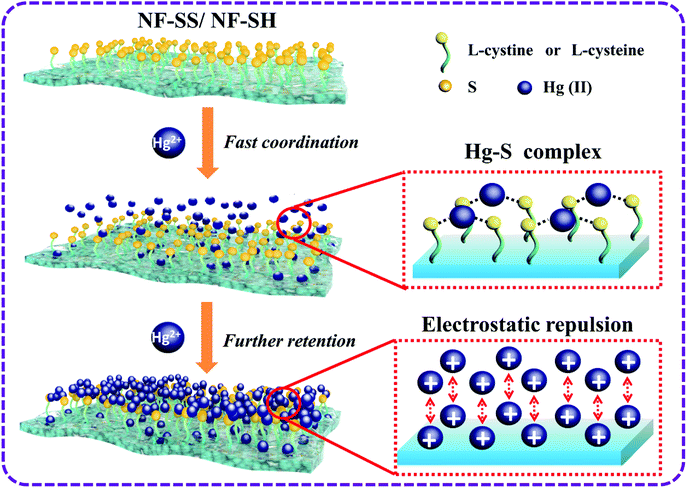 |
| Fig. 8 Suggested mechanism of NF-SS and NF-SH nanofiltration active layers for the removal of Hg(II) ion in multi-metal solution. | |
4. Conclusions
In summary, we have successfully fabricated two sulfur-impregnated composite nanofiltration active layers, NF-SS and NF-SH, that exhibit highly effective and highly efficient removal of selected soft heavy metal ions, especially Hg(II), from heavily contaminated aqueous solutions. Specifically, NF-SH was highly capable of reducing Hg(II) ion concentration from 10 ppm to the extremely low level of less than 0.18 ppb within the drinkable limits of 2 ppb and an unprecedentedly high removal rate of 99.99% for Hg(II) was achieved, outperforming those of the recently reported thiol/thio-functionalized adsorbents and other Hg(II)-scavenging materials. Additionally, the thiol/disulfide-functionalized layers are fully regenerable after six cycles of filtration and demetallization operations, and more than 91% and 96% of the Hg(II) ions can be retained. The sulfur-impregnated TFC membranes can remain effective in the presence of competing metal ions Ca(II), Mg(II), and Na(I), thus illustrating superior selectivity. We surmised that the introduction of sulfur species possessing a high affinity towards soft metals coupled with size exclusion of the NF barrier layer is responsible for the very selective and efficient purification process. When using the sulfur-impregnated NF active layers for desalination, an excellent permeance of 63 L m−2 h−1 and high rejection greater than 99.6% against Na2SO4 can be obtained. Overall, this work provides a facile and time-efficient approach to construct novel NF materials with high desalting capability and outstanding removal performance for heavy metal ions, and demonstrate the prospective application of sulfur-functionalized polyamide NF membrane in the fields of drinking water treatment and environmental remediation.
Conflicts of interest
There are no conflicts to declare.
Acknowledgements
This work was supported by the National Natural Science Foundation of China (51473097), the Sichuan Science and Technology Project (2019YJ0107), and the Opening Project of State Key Laboratory of Polymer Materials Engineering (Sichuan University) (sklpme2014-3-14).
References
- C. J. Vörösmarty, P. B. McIntyre, M. O. Gessner, D. Dudgeon, A. Prusevich, P. Green, S. Glidden, S. E. Bunn, C. A. Sullivan, C. R. Liermann and P. M. Davies, Global threats to human water security and river biodiversity, Nature, 2010, 467, 555–561 CrossRef PubMed
. - M. M. Mekonnen and A. Y. Hoekstra, Four billion people facing severe water scarcity, Sci. Adv., 2016, 2, e1500323 CrossRef PubMed
. - I. Haddeland, J. Heinke, H. Biemans, S. Eisner, F. Martina, N. Hanasaki, M. Konzmann, F. Ludwig, Y. Masaki, J. Schewe, T. Stacke, Z. D. Tessler, Y. Wada and D. Wisser, Global water resources affected by human interventions and climate change, Proc. Natl. Acad. Sci. U. S. A., 2014, 111, 3251–3256 CrossRef CAS PubMed
. - J. Guo, Q. Peng, H. Fu, G. Zou and Q. Zhang, Heavy-metal adsorption behavior of two-dimensional alkalization intercalated mxene by first-principles calculations, J. Phys. Chem. C, 2015, 119, 20923–20930 CrossRef CAS
. - V. Diana, P. Jemish, Z. Yongfei, Z. Yanli and S. Sánchez, Graphene-based microbots for toxic, heavy metal removal and recovery from water, Nano Lett., 2016, 16, 2860–2866 CrossRef PubMed
. - X. Li, C. Bian, X. Meng and F. S. Xiao, Design and synthesis of an efficient nanoporous adsorbent for Hg2+ and Pb2+ ions in water, J. Mater. Chem. A, 2016, 4, 5999–6005 RSC
. - M. Mcnutt, Mercury and health, Science, 2013, 341, 1430 CrossRef CAS PubMed
. - C. J. Clifton, Mercury Exposure and Public health, Pediatr. Clin. North Am., 2007, 54, 237.e1–237.e45 CrossRef PubMed
. - X. Wang, Z. Z. Zhang, Y. H. Zhao, K. Xia, Y. F. Guo, Z. Qu and R. B. Bai, A Mild and Facile Synthesis of Amino Functionalized CoFe2O4@SiO2 for Hg(II) Removal, Nanomaterials, 2018, 8(9), 673–694 CrossRef PubMed
. - Y. H. Zhao, K. Xia, Z. Z Zhang, Z. M. Zhu, Y. F. Guo and Z. Qu, Facile Synthesis of Polypyrrole-Functionalized CoFe2O4@SiO2 for Removal for Hg(II), Nanomaterials, 2019, 9, 455–476 CrossRef CAS PubMed
. - K. Xia, Y. F. Guo, Q. J. Shao, Q. Zan and R. B Bai, Removal of Mercury(II) by EDTA-Functionalized Magnetic CoFe2O4@SiO2 Nanomaterial with Core–Shell Structure, Nanomaterials, 2019, 9, 1532–1555 CrossRef CAS PubMed
. - M. M. Matlock, B. S. Howerton and D. A. Atwood, Chemical precipitation of heavy metals from acid mine drainage, Water Res., 2002, 36, 4757–4764 CrossRef CAS PubMed
. - A. Dabrowski, Z. Hubicki, P. Podkošcielny and E. Robens, Selective removal of the heavy metal ions from waters and industrial wastewaters by ion-exchange method, Chemosphere, 2004, 56, 91–106 CrossRef CAS PubMed
. - H. M. Xua, J. P. Jia, Y. F. Guo, Z. Qua, Y. Liao, J. K. Xie, W. F. Shangguan and N. Q. Yan, Design of 3D MnO2/Carbon sphere composite for the catalytic oxidation and adsorption of elemental mercury, J. Hazard. Mater., 2018, 342, 69–76 CrossRef PubMed
. - Z. Z. Zhang, K. Xia, Z. W. Pan, C. X. Yang, X. Wang, G. W. Zhang, Y. F. Guo and R. B. Bai, Removal of mercury by magnetic nanomaterial with bifunctional groups and core–shell structure: Synthesis, characterization and optimization of adsorption parameters, Appl. Surf. Sci., 2020, 500, 143970–143984 CrossRef CAS
. - C. Zhou, H. Zhu, Q. Wang, J. X. Wang, J. Cheng, Y. F. Guo, X. J. Zhou and R. B. Bai, Adsorption of mercury(II) with an Fe3O4 magnetic polypyrrole-graphene oxide nanocomposite, RSC Adv., 2017, 7, 18466–18479 RSC
. - I. C. Escobar and B. Van der Bruggen, Modern applications in membrane science and technology, American Chemical Society Books, Washington, DC, 2011 Search PubMed
. - H. A. Qdais and H. Moussa, Removal of heavy metals from wastewater by membrane processes: a comparative study, Desalination, 2014, 164, 105–110 CrossRef
. - C. Bellona, J. E. Drewes, P. Xu and G. Amy, Factors affecting the rejection of organic solutes during NF/RO treatment-a literature review, Water Res., 2004, 38, 2795–2809 CrossRef CAS PubMed
. - Z. W. Jiang, S. Karan and A. G. Livingston, Water transport through ultrathin polyamide nanofims used for reverse osmosis, Adv. Mater., 2018, 30, 1705973 CrossRef PubMed
. - J. Gao, S. P. Sun, W. P. Zhu and T. S. Chung, Chelating polymer modified p84 nanofiltration (NF) hollow fiber membranes for high efficient heavy metal removal, Water Res., 2014, 63, 252–261 CrossRef CAS PubMed
. - W. P. Zhu, J. Gao, S. P. Sun, S. Zhang and T. S. Chung, Poly(amidoamine) dendrimer (PAMAM) grafted on thin film composite (TFC) nanofiltration (NF) hollow fiber membranes for heavy metal removal, J. Membr. Sci., 2015, 487, 117–126 CrossRef CAS
. - A. K. Geim, Graphene: status and prospects, Science, 2009, 324, 1530–1534 CrossRef CAS PubMed
. - H. Huang, Y. Ying and X. Peng, Graphene oxide nanosheet: an emerging star material for novel separation membranes, J. Mater. Chem. A, 2014, 2, 13772–13782 RSC
. - Y. Zhang, S. Zhang and T. S. Chung, Nanometric graphene oxide framework membranes with enhanced heavy metal removal via nanofiltration, Environ. Sci. Technol., 2015, 49, 10235–10242 CrossRef CAS PubMed
. - Y. Zhang, S. Zhang, J. Gao and T. S. Chung, Layer-by-layer construction of graphene oxide (GO) framework composite membranes for highly efficient heavy metal removal, J. Membr. Sci., 2016, 515, 230–237 CrossRef CAS
. - P. Zhang, J. L. Gong, G. M. Zeng, C. H. Deng, H. C. Yang, H. Y. Liu and S. Y. Huan, Cross-linking to prepare composite graphene oxide-framework membranes with high-flux for dyes and heavy metal ions removal, Chem. Eng. J., 2017, 322, 657–666 CrossRef CAS
. - R. G. Pearson, Hard and soft acids and bases, J. Am. Chem. Soc., 1963, 85, 3533–3539 CrossRef CAS
. - J. Bao, Y. Fu and Z. Bao, Thiol-functionalized magnetite/graphene oxide hybrid as a reusable adsorbent for Hg2+ removal, Nanoscale Res. Lett., 2013, 8, 1–6 CrossRef PubMed
. - A. Mousavi, Are thiolate anions the only natural organic matter ligands for which mercury(II) has an exceptionally high affinity in aquatic ecosystems?, Rev. Chim., 2015, 66, 774–777 CAS
. - Y. Li, X. Zhang, B. Zhu, J. Xue and J. Yan, A disulfide-linked naphthalimide dimer for Hg(II) detection in aqueous solution, J. Fluoresc., 2011, 21, 1343–1348 CrossRef CAS PubMed
. - A. Denizli, K. Kesenci, Y. Arica and E. Pişkin, Dithiocarbamate-incorporated monosize polystyrene microspheres for selective removal of mercury ions, React. Funct. Polym., 2000, 44, 235–243 CrossRef CAS
. - S. Bao, K. Li, P. Ning, J. Peng, X. Jin and L. Tang, Highly effective removal of mercury and lead ions from wastewater by mercaptoamine-functionalised silica-coated magnetic nano-adsorbents: behaviours and mechanisms, Appl. Surf. Sci., 2017, 393, 457–466 CrossRef CAS
. - C. Zhang, J. Sui, J. Li, Y. Tang and W. Cai, Efficient removal of heavy metal ions by thiol-functionalized superparamagnetic carbon nanotubes, Chem. Eng. J., 2012, 210, 45–52 CrossRef CAS
. - F. Rouhani and A. Morsali, Fast and selective heavy metal removal by a novel metal–organic framework designed with in situ ligand building block fabrication bearing free nitrogen, Chem.–Eur. J., 2018, 24, 5529–5537 CrossRef CAS PubMed
. - K. Chen, Z. Z. Zhang, K. Xia, X. J. Zhou, Y. F. Guo and T. Y. Huang, Facile Synthesis of Thiol-Functionalized Magnetic Activated Carbon and Application for the Removal of Mercury(II) from Aqueous Solution, ACS Omega, 2019, 4, 8568–8579 CrossRef CAS PubMed
. - H. Zhu, Y. Shen, Q. Wang, K. Chen, X. Wang, G. W. Zhang, J. J. Yang, Y. F. Guo and R. B. Bai, Highly promoted removal of Hg(II) with magnetic CoFe2O4@SiO2 core-shell nanoparticles modified by thiol groups, RSC Adv., 2017, 7, 39204–39215 RSC
. - Q. Sun, B. Aguila, J. Perman, L. D. Earl, C. W. Abney, Y. Cheng, H. Wei, N. Nguyen, L. Wojtas and S. Ma, Postsynthetically modified covalent organic frameworks for efficient and effective mercury removal, J. Am. Chem. Soc., 2017, 139, 2786–2793 CrossRef CAS PubMed
. - N. Huang, L. Zhai, H. Xu and D. Jiang, Stable covalent organic frameworks for exceptional mercury removal from aqueous solutions, J. Am. Chem. Soc., 2017, 139, 2428 CrossRef CAS PubMed
. - D. T. Sun, L. Peng, W. S. Reeder, S. M. Moosavi, D. Tiana, D. K. Britt, E. Oveisi and W. L. Queen, Rapid, selective heavy metal removal from water by a metal–organic framework/polydopamine composite, ACS Cent. Sci., 2018, 4, 349–356 CrossRef CAS PubMed
. - M. Mon, X. Qu, J. Ferrando-Soria, I. Pellicer-Carreño, A. Sepúlveda-Escribano, E. V. Ramos-Fernandez, J. C. Jansen, D. Armentano and E. Pardo, Fine-tuning of the confined space in microporous metal–organic frameworks for efficient mercury removal, J. Mater. Chem. A, 2017, 5, 20120–20125 RSC
. - X. Sun, J. Y. Hwang and S. Xie, Density functional study of elemental mercury adsorption on surfactants, Fuel, 2011, 90, 1061–1068 CrossRef CAS
. - N. M. Bandaru, N. Reta, H. Dalal, A. V. Ellis, J. Shapter and N. H. Voelcker, Enhanced adsorption of mercury ions on thiol derivatized single wall carbon nanotubes, J. Hazard. Mater., 2013, 261, 534–541 CrossRef CAS PubMed
. - S. Ravi and M. Selvaraj, Incessant formation of chain-like mesoporous silica with a superior binding capacity for mercury, Dalton Trans., 2014, 43, 5299 RSC
. - O. Hakami, Y. Zhang and C. J. Banks, Thiol-functionalised mesoporous silica-coated magnetite nanoparticles for high efficiency removal and recovery of Hg from water, Water Res., 2012, 46, 3913–3922 CrossRef CAS
. - Y. Shin, G. E. Fryxell, W. Um, K. Parker, S. V. Mattigod and R. Skaggs, Sulfur-functionalized mesoporous carbon, Adv. Funct. Mater., 2007, 17, 2897–2901 CrossRef CAS
. - S. M. Xue, Z. L. Xu, Y. J. Tang and C. H. Ji, Polypiperazine-amide nanofiltration membrane modified by different functionalized multiwalled carbon nanotubes (MWCNTs), ACS Appl. Mater. Interfaces, 2016, 8, 19135–19144 CrossRef CAS
. - H. L. Zhang, Y. B. Gao and J. G. Gai, Guanidinium-functionalized nanofiltration membranes integrating anti-fouling and antimicrobial effects, J. Mater. Chem. A, 2018, 6(15), 6442–6454 RSC
. - M. B. M. Y. Ang, Y. L. Ji, S. H. Huang, H. A. Tsai, W. S. Hung, C. C. Hu, K. R. Lee and J. Y. Lai, Incorporation of carboxylic monoamines into thin-film composite polyamide membranes to enhance nanofiltration performance, J. Membr. Sci., 2017, 539, 52–64 CrossRef CAS
. - H. L. Zhang, B. H. Liu, M. B. Yang, P. Zhang and J. G. Gai, Sulfaguanidine nanofiltration active layer towards anti-adhesive and antimicrobial attributes for desalination and dye removal, RSC Adv., 2019, 9(36), 20715–20727 RSC
. - S. H. Huang, C. J. Hsu, D. J. Liaw, C. C. Hu, K. R. Lee and J. Y. Lai, Effect of chemical structures of amines on physicochemical properties of active layers and dehydration of isopropanol through interfacially polymerized thin-film composite membranes, J. Membr. Sci., 2008, 307, 73–81 CrossRef CAS
. - H. Zhou, X. Wang, J. Tang and Y. W. Yang, Tuning the growth, crosslinking, and gating effect of disulfide-containing PGMAs on the surfaces of mesoporous silica nanoparticles for redox/pH dual-controlled cargo release, Polym. Chem., 2016, 7, 2171–2179 RSC
. - S. Park, S. Yang, N. Shin, E. Lee and H. Lee, Adsorption configuration for cysteine on Ge(100): coverage-dependent surface reorientation, J. Phys. Chem. C, 2010, 114, 14528–14531 CrossRef CAS
. - Y. Gao, S. Zhao, Z. Qiao, Y. Zhou, B. Song, Z. Wang and J. Wang, Reverse osmosis membranes with guanidine and amine enriched surface for biofouling and organic fouling control, Desalination, 2018, 430, 74–85 CrossRef CAS
. - E. M. Hoek, S. Bhattacharjee and M. Elimelech, Effect of membrane surface roughness on colloid-membrane DLVO interactions, Langmuir, 2003, 19, 4836–4847 CrossRef CAS
. - R. Dawson, A. I. Cooper and D. J. Adams, Nanoporous organic polymer network, Prog. Polym. Sci., 2012, 37, 530–563 CrossRef CAS
. - J. Wang, Z. Wang, J. Wang and S. Wang, Improving the water flux and bio-fouling resistance of reverse osmosis (RO) membrane through surface modification by zwitterionic polymer, J. Membr. Sci., 2015, 493, 188–199 CrossRef CAS
. - J. M. M. Peeters, J. P. Boom, M. H. V. Mulder and H. Strathmann, Retention measurements of nanofiltration membranes with electrolyte solutions, J. Membr. Sci., 1998, 145, 199–209 CrossRef CAS
. - A. Seidel, J. J. Waypa and M. Elimelech, Role of charge (Donnan) exclusion in removal of arsenic from water by a negatively charged porous nanofiltration membrane, Environ. Eng. Sci., 2004, 18, 105–113 CrossRef
. - R. J. Petersen, Composite reverse osmosis and nanofiltration membranes, J. Membr. Sci., 1993, 83, 81–150 CrossRef CAS
. - U. S. Environmental Protection Agency, 2012 ed. of the Drinking Water Standards and Health Advisories, 2012 Search PubMed
. - C. C. Chen, E. J. Mckimmy, T. J. Pinnavaia and K. F. Hayes, XAS study of mercury(II) ions trapped in mercaptan-functionalized mesostructured silicate with a wormhole framework structure, Environ. Sci. Technol., 2004, 38, 4758–4762 CrossRef CAS PubMed
. - T. Gebremedhin-Haile, M. T. Olguín and M. Solache-ríos, Removal of mercury ions from mixed aqueous metal solutions by natural and modified zeolitic minerals, Water, Air, Soil Pollut., 2003, 148, 179–200 CrossRef CAS
. - Y. Oh, C. D. Morris and M. G. Kanatzidis, Polysulfide chalcogels with ion-exchange properties and highly efficient mercury vapor sorption, J. Am. Chem. Soc., 2012, 134, 14604–14608 CrossRef CAS PubMed
. - Y. Oh, S. Bag, C. D. Malliakas and M. G. Kanatzidis, Selective surfaces: high-surface-area zinc tin sulfide chalcogels, Chem. Mater., 2011, 23, 2447–2456 CrossRef CAS
. - M. Khazaei, S. Nasseri, M. R. Ganjali, M. Khoobi, R. Nabizadeh, E. Gholibegloo and S. Nazmara, Selective removal of mercury(II) from water using a 2,2-dithiodisalicylic acid-functionalized graphene oxide nanocomposite: Kinetic, thermodynamic, and reusability studies, J. Mol. Liq., 2018, 265, 189–198 CrossRef CAS
. - I. L. Lagadic, M. K. Mitchell and B. D. Payne, Highly effective adsorption of heavy metal ions by a thiol-functionalized magnesium phyllosilicate clay, Environ. Sci. Technol., 2001, 35, 984–990 CrossRef CAS PubMed
. - A. Denizli, K. Kesenci, Y. Arica and E. Pi şkin, Dithiocarbamate-incorporated monosize polystyrene microspheres for selective removal of mercury ions, React. Funct. Polym., 2000, 44, 235–243 CrossRef CAS
.
Footnotes |
† Electronic supplementary information (ESI) available. See DOI: 10.1039/c9ra09380j |
‡ These authors contributed equally to this work and should be considered co-first authors. |
|
This journal is © The Royal Society of Chemistry 2020 |
Click here to see how this site uses Cookies. View our privacy policy here.