DOI:
10.1039/C9RA09293E
(Paper)
RSC Adv., 2020,
10, 7751-7757
Cooperative CO2 absorption by amino acid-based ionic liquids with balanced dual sites†
Received
8th November 2019
, Accepted 4th February 2020
First published on 25th February 2020
Abstract
In this study, a variety of functionalized ILs with dual sites including amino acid group (AA) and basic anion (R) were synthesized to investigate the suppression and cooperation between the sites in CO2 absorption. The basic anions selected in this study with different basicity include sulfonate (Su), carboxylate (Ac), imidazolium (Im), and indolium (Ind). These ILs ([P66614]2[AA–R]) were applied to CO2 absorption. The results present that CO2 capacity increases first and then decreases later with the continuous increase in the activity of the anion site. Combined with CO2 absorption experiments, IR and NMR spectroscopic analyses and DFT calculation demonstrate that the ability of one site to capture CO2 would be suppressed when the activity of another site is much stronger. Thus, the cooperation of dual site-functionalized ILs and high CO2 capacity might be achieved through balancing the two sites to be equivalent. Based on this point, [P66614]2[5Am–iPA] was further synthesized by taking the advantage of the conjugated benzene ring. As expected, [P66614]2[5Am–iPA] showed capacity as high as 2.38 mol CO2 per mol IL at 30 °C and 1 bar without capacity decrease even after 10 times recycling performance of CO2 absorption and desorption.
Introduction
Rapid anthropogenic climate change caused by a large number of greenhouse gases is one of the most significant environmental problems in the world today.1 The development of sustainable and environmentally friendly technology to reduce greenhouse gas emissions, particularly carbon dioxide (CO2), is the focus of attention in many countries. Unique physicochemical properties of ionic liquids (ILs) such as high thermal stability, low vapor pressure, and tunable properties make ILs suitable for CO2 absorption.2,3 Functionalized ILs, such as amine,4,5 azolium,6 phenolate,7 and carbene-based ILs8 were reported as potential CO2 absorption solvents owing to their electron-rich property, or the basicity of ILs. High CO2 absorption capacity is one of the performance evaluations of ILs. In this regard, a series of strategies, including tuning the basicity of the active sites,9–11 changing the steric hindrance of the ILs,12,13 utilization of entropic effects14,15 and hydrogen bond formation,16,17 were developed to enhance the CO2 absorption capacity. However, the CO2 capacity of ILs with single site was low compared to those multiple-sites, which have attracted attention to enhance the CO2 capacity.
Designing ILs with cooperative sites was considered as attractive to enhance the CO2 capacity as well as gas adsorption materials. Vaidhyanathan and Woo18 reported CO2 capture by an amine-functionalized nanoporous solid with cooperative sites for the low-pressure binding and large uptake of CO2. McDonald19 reported an energy-saving CO2 separation by small temperature or pressure swings via the cooperative insertion of CO2 in diamine-appended MOFs such as mmen-Mg2(dobpdc). It has been found that cooperative CO2 capture is a much feasible way to achieve high capacity and reversibility.20,21 Recently, cooperative sites were considered in designing ILs. Wang et al.22 synthesized hydroxyl-pyridinium based ILs with dual cooperative sites to fix CO2 for the delocalized π electrons, which enhanced by 85% of the capacity to 1.58 mol mol−1 IL. Similarly, the cooperation of Lewis acid–basic reaction and the hydrogen bond interactions of IL with CO2 were put to good use to improve CO2 capture with imidazolium ILs.16 Dai et al.23 found the dual sites of [P4442]2[IDA] could be activated by weakening the depression of amine and high capacity of 1.69 mol CO2 per mol [P4442]2[IDA] was achieved. Wang and Cui24 synthesized [P4442][Suc] with structural preorganization for improving the capture to 1.65 mol mol−1 IL of low-concentration CO2 as 10 vol% through multiple site cooperation, the capacity would be further enhanced to 2.21 mol mol−1 IL at 20 °C and 1.0 bar by tuning the anion substituent group.25 However, [apmim]26 with the active sites of imidazolium anion and amine group in cation, [aemmim][Tau]27 and [aP4443][AA]28 with an amine group both in cation and anion, [DAIL]29 with a dual amine group in cation as well as [Arg], [Lys], [AA–Im]-based ILs30,31 with a dual amine group in cation were reported as a CO2 absorbent but have capacity just up to equimolar similar to the ILs with a single site, which means one site might be suppressed or inactive. Mu27 thought that there is interplay of the dual amine in ILs, which might restrict their ability. As can be seen, cooperation is the key to the high capacity of ILs with dual sites,32,33 but the depression of one site is a common problem making the active site to be suppressed and have low CO2 capacity.
A dual site-functionalized IL consists of three parts including cation, site A and site B in anion, as shown in Fig. 1. Two possible causes influence the activity of sites, including the interactions between a cation and anion, and the interactions between site A and site B. In our previous study, the effects of cation was investigated and the results indicated that strong interactions between cation and anion would deactivate one site.34 In this study, the interplay between dual sites was investigated; amino acids with binary acids are considered as an anion precursor to investigate the depression effects of CO2 absorption sites. Phosphonium ions [P66614] and [P4442] are selected as the cations. The structure of the used ILs is presented in Chart 1. There are two potential sites including amino acid (AA) and anion site (R) in [AA–R]. Thereinto, it has been reported that [P66614][AA] could capture CO2 efficiently via the reaction of an amine group with CO2 to carbamic acid. Indolium (Ind) and imidazolium (Im) ions are also good choices for CO2 absorption with high capacity, while carboxylate (Ac) anion prefers to react with CO2 and should be active to fix CO2 efficiently, sulfonate (Su) ion would not react with CO2. The results in this study indicate that the dual sites in ILs could cooperate and do their best in the CO2 capture if two sites have quite an activity; otherwise, the less active site would be suppressed by another site. Furthermore, [P66614]2[Am–iPA] was synthesized with equivalent dual sites to cooperative CO2 absorption, and the results showed that it presented high capacity as 2.38 mol CO2 per mol IL at 30 °C and 1 bar.
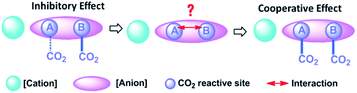 |
| Fig. 1 Schematic of the interactions of CO2 with dual site-functionalized IL. | |
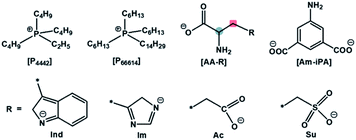 |
| Chart 1 The structure of cations and dual-site anions of ILs. | |
Results and discussion
Properties of ILs
Some properties of these synthesized ILs such as their thermal property and viscosity were detected, and are shown in Table 1. The decomposition temperature of [P66614]2[AA–Im] and [P66614]2[AA–Ind] containing azole groups are 258 °C and 252 °C, respectively. [P66614]2[Am–PA] with a benzene substituent was stable until the temperature reached above 300 °C, and they are thermally stable enough for the application as a CO2 absorbent. The viscosity of these ILs are thousands of cPa and it is considered that the high viscosity was derived from the hydrogen bond formation in amine-based ILs.
Table 1 The viscosity and decomposition temperature of typical ILs
Entry |
IL |
Viscositya (cPa) |
Decomposition temperatureb (°C) |
Viscosity date were obtained using a Bookfield DV-II+ Pro viscometer at 30 °C. Decomposition temperature was measured by DTG with temperature increase from 30 °C to 600 °C at a rate of 10 °C min−1 under an argon gas flow. |
1 |
[P66614]2[AA–Im] |
2693 |
258 |
2 |
[P66614]2[AA–Su] |
4432 |
296 |
3 |
[P66614]2[AA–Ac] |
3076 |
264 |
4 |
[P66614]2[AA–Ind] |
4220 |
252 |
5 |
[P66614]2[Am–iPA] |
5135 |
320 |
CO2 absorption
These ILs were applied to CO2 absorption under 1 bar CO2 pressure at 30 °C (Fig. 2). [P66614]2[AA–Ac] showed a high capacity of 1.97 mol CO2 per mol IL. With an exchange of a cation, the IL [P4442]2[AA–Ac] shows a capacity of 2.05 mol CO2 per mol IL, with no obvious distinction with the exchange of a phosphonium cation, and this phenomenon is similar to that observed in the previous studies.34,35 Thus, the following experiments take [P66614] as the ILs' cation. Otherwise, [P66614]2[AA–Su] presents a CO2 capacity of 0.49 mol mol−1 IL, which is less than [P66614]2[AA–Ac] for the sulfonic moiety that does not react with CO2. According to the ref. 6, azolium ions such as [Ind] and [Im] prefer to react with CO2. [P66614]2[AA–Ind] and [P66614]2[AA–Im] with azolium ions were applied to CO2 absorption. However, their CO2 absorption capacities are 1.45 and 1.55 mol mol−1 IL, respectively, which are lower than that of [P66614]2[AA–Ac]. This means the activity of the CO2 absorption site AA or azolium ions might be suppressed.
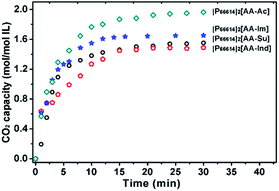 |
| Fig. 2 Properties of the CO2 absorption of dual site-functionalized ILs at 30 °C and 1 bar CO2 pressure. | |
The possible mechanism for CO2 absorption
The possible interaction process controlled by enthalpy was speculated via theoretical calculations performed using the Gaussian 03 program at the B3LYP/6-31G++(d,p) level, the optimized structures of the [AA–R], and its CO2 complexes are listed in Fig. S1† and the enthalpies are listed in Table 2. It is reported that CO2 could be chemisorbed when the reaction enthalpy is less than about −50 kJ mol−1.6 As seen from Table 2, the amine groups are all active for CO2 absorption according to the reaction enthalpy ΔH(AA–CO2). The reaction enthalpy of CO2 with an Ac anion in [AA–Ac] is −61.08 kJ mol−1, which means that Ac could react with CO2 efficiently, and this result coincides with the absorption capacity of [P66614]2[AA–Ac] as 1.97 mol CO2 per mol IL. The Su anion is not an active site for CO2 absorption because the reaction enthalpy of CO2 with the Su anion in [AA–Su] is −27.15 kJ mol−1, which causes the CO2 capacity of [P66614]2[AA–Su] presenting 0.49 mol mol−1 less than [P66614]2[AA–Ac]. The reaction enthalpies of the amine group and Im anion in [AA–Im] with CO2 are −102.28 and −130.68 kJ mol−1, which indicates that two sites might react with CO2 preferentially. However, [P66614]2[AA–Im] also showed 0.42 mol CO2 per mol IL less than [P66614]2[AA–Ac] and is similar to [P66614]2[AA–Ind]. There is an inhibiting effect between sites including anion and amine, where the amine site competes with anion in CO2 reaction. The CO2 capacity was associated with the relative activation of two sites, which was considered as the value of ΔH(R–CO2) divided by ΔH(AA–CO2). The results in Fig. 3 indicate that there is a high CO2 capacity when the activation of two sites are almost equivalent, whereas the CO2 capacity is much lower.
Table 2 Reaction enthalpies of CO2 with one site of [AA–R]
[AA–R] |
Reaction enthalpya |
CO2 capacityb |
Relative activation of two sitesc |
ΔH(AA–CO2) |
ΔH(R–CO2) |
kJ mol−1, the reaction enthalpies were calculated by the Gaussian program at B3LYP/6-31G++(d,p) level. mol mol−1 IL, CO2 absorption was operated at 30 °C, 1 bar. Relative activation of two sites was presented as ΔH(AA–CO2) divide by ΔH(R–CO2). |
[AA–Ac] |
−106.15 |
−61.08 |
1.97 |
0.58 |
[AA–Su] |
−103.67 |
−27.15 |
1.48 |
0.26 |
[AA–Im] |
−102.28 |
−130.68 |
1.55 |
1.28 |
[AA–Ind] |
−105.32 |
−136.78 |
1.45 |
1.30 |
[Am–iPA] |
−75.85 |
−64.53 |
2.38 |
0.85 |
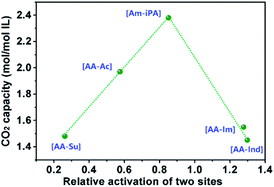 |
| Fig. 3 Properties of CO2 absorption capacity of dual sites ILs varied with relative activation of two sites presented as ΔH(R–CO2) divide by ΔH(AA–CO2). | |
The CO2 absorption with these ILs was investigated via IR and 13C NMR spectroscopy, as shown in Fig. 4. There are 2 new peaks at 160.4 ppm and 158.2 ppm in the 13C NMR spectra compared with CO2 saturated [P66614]2[AA–Ac] and its fresh state, while the chemical shift of the CH (marked with green circle) and CH2 (marked with red square) groups have a few ppm changes, which indicates that CO2 is fixed in two forms. As seen from the IR spectra of [P66614]2[AA–Ac] in Fig. 4(b), the vibration absorption of the carboxylate anion at 1585 cm−1 shift to 1610 cm−1 and the IR absorption intensity of the captured CO2 between 1630–1760 cm−1 increases with a gradual increase in the CO2 content, which indicates that the carboxylate anion assists in CO2 absorption.
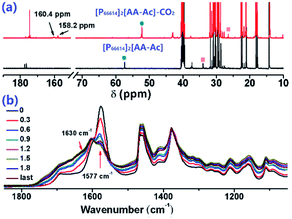 |
| Fig. 4 (a) 13C NMR spectra of [P66614]2[AA–Ac] compared with its CO2 saturated complex, (b) IR spectra of [P66614]2[AA–Ac] varied with CO2 content. | |
The 2D IR spectroscopy is a usual method to study the dynamics of interactions.36,37 Thereinto, the IR spectra of ILs associated with the CO2 content in 2D correction forms are shown in Fig. 5. Compared with the synchronous and asynchronous correction of [P66614]2[AA–Ac], it is interesting that the absorption between 1630–1780 cm−1 consists of several peaks. There are cross-correlation peaks marked with the elliptical line that appeared at (1715 cm−1, 1585 cm−1), where 1585 cm−1 belongs to the carboxylate anion and 1715 cm−1 belongs to the fixed CO2. The Ψ(1715 cm−1, 1585 cm−1) in Fig. 5(a) is opposite in signs to Φ(1715 cm−1, 1585 cm−1) in Fig. 5(c), which indicates that the change of 1585 cm−1 precedes 1715 cm−1. Similarly, with the analysis of the correlation among the peaks of [P66614]2[AA–Ac] at 1585, 1660 and 1715 cm−1, it indicates that the absorption at 1660 cm−1 belongs to the fixed CO2 with the amine group in [AA–Ac] also follows 1585 cm−1, while 1715 cm−1 and 1660 cm−1 appear simultaneously for no cross-correlation peak at Φ(1713 cm−1, 1660 cm−1), which is marked with dotted square in Fig. 5(c). Thus, the amine site of [AA–Ac] reacts with one CO2, then another CO2 fixed by an anion site, dual sites of ILs such as [P66614]2[AA–Ac] could be listed as path (a) in Fig. 6, it presents high capacity of up to 1.97 mol CO2 per mol IL owing to the cooperation of amine group and the carboxylate anion.
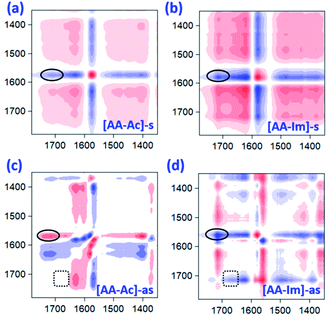 |
| Fig. 5 Two-dimensional correction of the IR spectra of typical ILs with CO2 content as a variable factor. (a) Synchronous correction of [P66614]2[AA–Ac]; (b) synchronous correction of [P66614]2[AA–Im]; (c) asynchronous correction of [P66614]2[AA–Ac]; (d) asynchronous correction of [P66614]2[AA–Im]. Red represents positive intensity and blue negative intensity. | |
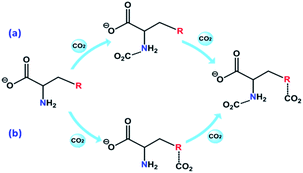 |
| Fig. 6 Possible reaction mechanism of dual sites ILs. | |
However, what happens on using [P66614]2[AA–Im] as a CO2 absorption agent?
As can be seen from Fig. S3† that the partial IR spectra of [P66614]2[AA–Im] varied with the CO2 content, the IR absorption of the carboxylate anion at 1578 cm−1 gets blueshift with the uptake of CO2, and the absorption of the fixed CO2 with the Im and amine group appear at 1713 and 1664 cm−1, respectively. Compared with the synchronous and asynchronous 2D correction IR spectra of [P66614]2[AA–Im] associated with the CO2 content, the sign of Ψ(1713 cm−1, 1578 cm−1) in Fig. 5(b) and Φ(1713 cm−1, 1578 cm−1) in Fig. 5(d) indicate the absorption of fixed CO2 by imidazolium at 1713 cm−1 before the change in the vibration absorption of the carboxylate anion at 1578 cm−1. Similarly, the IR absorption of the fixed CO2 with the amine group in [AA–Im] at 1664 cm−1 follows 1713 cm−1, while the absorption appears at 1664 cm−1 prior to 1578 cm−1. Thus, the reaction of CO2 with dual site ILs could be listed as path (b) in Fig. 6, that the amine site of [AA–Im] reacts with CO2 following with CO2 fixed by anion site, which is different from [P66614]2[AA–Ac]. The 13C NMR spectra in Fig. 7 shows that one new carbon appeared at 157.9 ppm compared with CO2 saturated [P66614]2[AA–Im] and its fresh state. It is noticed that the chemical shift of CH (marked with a green circle) group shifts tiny, which would be attributed to the reaction of the amine group with CO2 be suppressed. A similar phenomenon occurs in CO2 absorption with [P66614]2[AA–Ind]. We thought that cooperative CO2 absorption with dual site-functionalized ILs might be liable to occur when the dual sites have the equivalent ability.
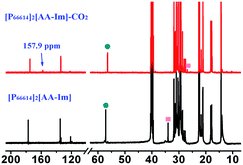 |
| Fig. 7 Partial 13C NMR spectra of [P66614]2[AA–Im] compared with [P66614]2[AA–Im]–CO2. | |
Application of cooperation
Based on this opinion that the sites with equivalent ability are more likely to form cooperative CO2 absorption, we know that the valence electron delocalizes in the conjugated plane, so that the charge can be dispersed. Thereinto, [P66614]2[Am–iPA] was synthesized by taking advantage of a benzene ring, which is one of the most common conjugated planes. The reaction enthalpy of CO2 with the amine group and carboxylate anion were calculated to predict the possibility of the cooperative CO2 absorption of dual sites. The reaction enthalpy of CO2 with carboxylate anion and amine in [Am–iPA] are −64.53 and −75.85 kJ mol−1, respectively, which indicate that two sites might react with CO2, and the reactions are more moderate than with [AA–Im] and [AA–Ind], which is a benefit to CO2 desorption. [P66614]2[Am–iPA] was applied to CO2 absorption with capacity as high as 2.38 mol mol−1 IL within 20 min at 30 °C. There are two new carbon peaks of captured CO2 with [P66614]2[Am–iPA] that appeared at 157.7 and 156.5 ppm in the 13C NMR spectra from Fig. 8(c). In the carbon capture utilization, moisture may be one of the strongest competitors to CO2 in the absorption with ILs; therefore, the humid CO2 absorption performance with [P66614]2[Am–iPA] was tested at 30 °C (Fig. S5†). The results indicated that [P66614]2[Am-iPA] was diluted with 2.5 wt% water, and the CO2 absorption capacity of [P66614]2[Am–iPA] with the copresence of water remained at 8.56 wt%. Compared with the dry CO2 absorption capacity of 9.14 wt% (2.38 mol CO2 per mol IL), it was demonstrated that a small amount of water in IL did not significantly reduce the CO2 capture capability.33,34 The influence of temperature and pressure were investigated. The results in Fig. 8(a and b) indicate that the CO2 capacity decreases to 1.19 mol mol−1 when temperature increase to 70 °C under 1.0 bar, or decreases to 1.79 mol mol−1 when the CO2 partial pressure decreases to 0.1 bar at 30 °C, which means that the captured CO2 could be desorbed by increasing the temperature and decreasing the CO2 partial pressure. The thermal stability of [P66614]2[Am–iPA] was characterized via TGA measurement with its decomposition temperature set as 322 °C. Ten consecutive absorption cycles of CO2 by [P66614]2[Am–iPA] are displayed in Fig. 8(d) and exhibit reversibility with the captured CO2 being desorbed at 80 °C and 1 kPa vacuum for 1 hour. With the cooperation of dual sites, higher CO2 capacity as well as weaker interactions between CO2 and IL could be achieved.
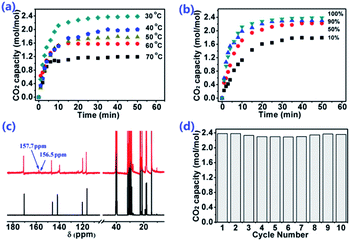 |
| Fig. 8 Properties of CO2 absorption with [P66614]2[Am–iPA]. (a) CO2 capacity varied with temperature. (b) CO2 capacity varied with the CO2 content of CO2 and N2 mixed gas at 1 bar pressure. (c) 13C NMR spectra of [P66614]2[Am–iPA] compared with the [P66614]2[Am–iPA]–CO2 complex. (d) 10 recycle of CO2 absorption at 30 °C and 1 bar CO2 pressure, and desorption at 80 °C and 1 kPa vacuum for 1 hour. | |
Experimental
Materials and methods
The used reagents such as DL-aspartic acid (AA–Ac), L-histidine (AA–Im), tryptophan (AA–Ind), DL-homocysteic acid (AA–Su), and 5-aminoisophthalic acid (Am–iPA) were purchased from Aladdin Industrial Co. Tributylethylphosphonium bromide and trihexyl(tetradecyl)phosphonium bromide were purchased from Nanjing Chemlin Biomedical Technology Company. An anion exchange resin in the chloride form (95% purity) and tributylamine (98% purity) were purchased from Sinopharm Group Chemical Company.
Synthetic procedures
ILs were prepared via synthesizing halide ILs, anion exchange, and neutralization with amino acids. Taking the synthesis of trihexyl(tetradecyl)phosphonium tryptophan ([P66614]2[AA–Ind]) as an example. Trihexyl(tetradecyl)phosphonium bromide ([P66614][Br]) was synthesized according to the reported ref. 38. A solution of trihexyl(tetradecyl)phosphonium hydroxide ([P66614][OH]) in ethanol was obtained from [P66614][Br] by the anion-exchange method,4 and equimolar tryptophan was added to the ethanol solution of [P66614][OH]; the mixture was dealt with a vacuum-rotary evaporation procedure at 60 °C to remove the most of ethanol after stirring at room temperature for 24 h. Then, all ILs were dried under vacuum at 80 °C for 24 h and freeze-dried to remove possible traces of water.
Characterization
The synthesized ILs were confirmed via NMR and FTIR spectroscopies (see the NMR and IR absorption data in ESI†), and FTIR spectra were recorded using a Nicolet iS50 FT-IR spectrometer. 1H and 13C NMR spectra were recorded on a 500 MHz Bruker Avance III spectrometer in a deuterated reagent using tetramethylsilane as the standard, and the purities of ILs were calculated based on the NMR spectra. Moreover, the content of the ILs used in this work in higher than 95%. Furthermore, the water content of these ILs was determined by Karl Fischer titration, which was lower than 0.5 wt%. The viscosity of ILs was detected on a Brookfield DV-II+ Pro viscometer at 30 °C. Their thermal stability was analysed via TGA on Shimadzu DTG-60H with an increase in temperature from 25 °C to 600 °C with an increased ratio of 10 °C min−1 under N2 gas protection.
CO2 absorption
CO2 absorption experiments with the IL were carried out according to our previous report. CO2 desorption was operated under a vacuum pressure of 1 kPa for an hour to recover the IL. CO2 capture properties with IL were proposed via calculation, and all calculations were performed in this study using the Gaussian 03 programs package. For each set of calculations, we calculated the geometry optimization for the free anion and the cation–CO2 complex, and their energies at the B3LYP/6-31G++(d,p) level. 2D correlation IR spectroscopy was acquired via a 2D software based on Fourier transform and the analysis was according to the so-called Noda's rule. The intensity of the synchronous 2D correlation spectrum Φ(ν1, ν2) represents the simultaneous or coincidental changes in two spectral intensity variations measured at ν1 and ν2 during the measurement interval of the NH3 content, while the intensity of an asynchronous spectrum Ψ(ν1, ν2) represents sequential or successive but not coincidental changes in spectrum measured separately at ν1 and ν2.
Conclusions
In summary, a variety of dual site-functionalized ILs [P66614]2[AA–R] were synthesized with an amino acid group and basic anion including sulfonate, carboxylate, imidazolium, and indolium to investigate the suppression and cooperation between each site in CO2 absorption. Combined with CO2 absorption experiments, IR and NMR spectroscopic analyses, and DFT calculations, CO2 absorption results indicated that the CO2 capacity increases first but decreases later with the continuous increase in the CO2 absorption ability of R. The ability of the amine group to capture CO2 would be suppressed when the interactions of another site with CO2 is stronger. Thus, the dual site-functionalized ILs might be cooperative to achieve high CO2 capacity by balancing two sites to be equivalent. Based on this point, [P66614]2[Am–iPA] was further synthesized by taking the advantage of a conjugated benzene ring. As expected, [P66614]2[Am–iPA] showed capacity as high as 2.38 mol CO2 per mol IL and without capacity decrease within 10 times recycle performance of CO2 absorption and desorption. Cooperation exists widely in a variety of fields as well as gas absorption, and the investigation of the knowledge of suppression would be better to achieve cooperation.
Conflicts of interest
There are no conflicts to declare.
Acknowledgements
This work was supported by the National Natural Science Foundation of China (21803021), Natural Science of Fujian Educational Foundation (JZ160407), Fujian Provincial Natural Science Foundation of China (2016J01060, 2017J01016), Education and Scientific Research Project from Fujian (JAT170032). We acknowledge the Subsidized Project for Postgraduates' Innovative Fund of Scientific Research of Huaqiao University and the Instrumental Analysis Centre of Huaqiao University.
References
- M. R. Raupach, G. Marland, P. Ciais, C. Le Quere, J. G. Canadell, G. Klepper and C. B. Field, Proc. Natl. Acad. Sci. U. S. A., 2007, 104, 10288–10293 CrossRef CAS PubMed.
- J. H. Huang and T. Ruther, Aust. J. Chem., 2009, 62, 298–308 CrossRef CAS.
- M. Aghaie, N. Rezaei and S. Zendehboudi, Renewable Sustainable Energy Rev., 2018, 96, 502–525 CrossRef CAS.
- B. E. Gurkan, J. C. de la Fuente, E. M. Mindrup, L. E. Ficke, B. F. Goodrich, E. A. Price, W. F. Schneider and J. F. Brennecke, J. Am. Chem. Soc., 2010, 132, 2116–2117 CrossRef CAS PubMed.
- E. D. Bates, R. D. Mayton, I. Ntai and J. H. Davis, J. Am. Chem. Soc., 2002, 124, 926–927 CrossRef CAS PubMed.
- C. M. Wang, X. Y. Luo, H. M. Luo, D. E. Jiang, H. R. Li and S. Dai, Angew. Chem., Int. Ed., 2011, 50, 4918–4922 CrossRef CAS PubMed.
- T. Umecky, M. Abe, T. Takamuku, T. Makino and M. Kanakubo, J. CO2 Util., 2019, 31, 75–84 CrossRef CAS.
- G. Gurau, H. Rodriguez, S. P. Kelley, P. Janiczek, R. S. Kalb and R. D. Rogers, Angew. Chem., Int. Ed., 2011, 50, 12024–12026 CrossRef CAS PubMed.
- C. M. Wang, H. M. Luo, H. R. Li, X. Zhu, B. Yu and S. Dai, Chem.–Eur. J., 2012, 18, 2153–2160 CrossRef CAS PubMed.
- K. Huang, Y. T. Wu and S. Dai, Ind. Eng. Chem. Res., 2015, 54, 10126–10133 CrossRef CAS.
- S. Seo, M. Quiroz-Guzman, M. A. DeSilva, T. B. Lee, Y. Huang, B. F. Goodrich, W. F. Schneider and J. F. Brennecke, J. Phys. Chem. B, 2014, 118, 5740–5751 CrossRef CAS PubMed.
- A. H. Liu, R. Ma, C. Song, Z. Z. Yang, A. Yu, Y. Cai, L. N. He, Y. N. Zhao, B. Yu and Q. W. Song, Angew. Chem., Int. Ed., 2012, 51, 11306–11310 CrossRef CAS PubMed.
- B. F. Goodrich, J. C. de la Fuente, B. E. Gurkan, Z. K. Lopez, E. A. Price, Y. Huang and J. F. Brennecke, J. Phys. Chem. B, 2011, 115, 9140–9150 CrossRef CAS PubMed.
- X. Y. Luo, F. Ding, W. J. Lin, Y. Q. Qi, H. R. Li and C. M. Wang, J. Phys. Chem. Lett., 2014, 5, 381–386 CrossRef CAS PubMed.
- S. Seo, M. A. DeSilva, H. Xia and J. F. Brennecke, J. Phys. Chem. B, 2015, 119, 11807–11814 CrossRef CAS PubMed.
- F. Ding, X. He, X. Y. Luo, W. J. Lin, K. H. Chen, H. R. Li and C. M. Wang, Chem. Commun., 2014, 50, 15041–15044 RSC.
- B. Hong, L. D. Simoni, J. E. Bennett, J. F. Brennecke and M. A. Stadtherr, Ind. Eng. Chem. Res., 2016, 55, 8432–8449 CrossRef CAS.
- R. Vaidhyanathan, S. S. Iremonger, G. K. H. Shimizu, P. G. Boyd, S. Alavi and T. K. Woo, Science, 2010, 330, 650–653 CrossRef CAS PubMed.
- T. M. McDonald, J. A. Mason, X. Q. Kong, E. D. Bloch, D. Gygi, A. Dani, V. Crocella, F. Giordanino, S. O. Odoh, W. S. Drisdell, B. Vlaisavljevich, A. L. Dzubak, R. Poloni, S. K. Schnell, N. Planas, K. Lee, T. Pascal, L. W. F. Wan, D. Prendergast, J. B. Neaton, B. Smit, J. B. Kortright, L. Gagliardi, S. Bordiga, J. A. Reimer and J. R. Long, Nature, 2015, 519, 303–304 CrossRef CAS PubMed.
- R. Vaidhyanathan, S. S. Iremonger, G. K. H. Shimizu, P. G. Boyd, S. Alavi and T. K. Woo, Angew. Chem., Int. Ed., 2012, 51, 1826–1829 CrossRef CAS PubMed.
- R. L. Siegelman, T. M. McDonald, M. I. Gonzalez, J. D. Martell, P. J. Milner, J. A. Mason, A. H. Berger, A. S. Bhovvn and J. R. Long, J. Am. Chem. Soc., 2017, 139, 10526–10538 CrossRef CAS PubMed.
- X. Y. Luo, Y. Guo, F. Ding, H. Q. Zhao, G. K. Cui, H. R. Li and C. M. Wang, Angew. Chem., Int. Ed., 2014, 53, 7053–7057 CrossRef CAS PubMed.
- F. F. Chen, K. Huang, Y. Zhou, Z. Q. Tian, X. Zhu, D. J. Tao, D. E. Jiang and S. Dai, Angew. Chem., Int. Ed., 2016, 55, 7166–7170 CrossRef CAS PubMed.
- Y. J. Huang, G. K. Cui, Y. L. Zhao, H. Y. Wang, Z. Y. Li, S. Dai and J. J. Wang, Angew. Chem., Int. Ed., 2017, 56, 13293–13297 CrossRef CAS PubMed.
- Y. J. Huang, G. K. Cui, H. Y. Wang, Z. Y. Li and J. J. Wang, J. CO2 Util., 2018, 28, 299–305 CrossRef CAS.
- Y. Zhang, Z. Wu, S. Chen, P. Yu and Y. Luo, Ind. Eng. Chem. Res., 2013, 52, 6069–6075 CrossRef CAS.
- Z. M. Xue, Z. F. Zhang, J. Han, Y. Chen and T. C. Mu, Int. J. Greenhouse Gas Control, 2011, 5, 628–633 CrossRef CAS.
- Y. Q. Zhang, S. J. Zhang, X. M. Lu, Q. Zhou, W. Fan and X. P. Zhang, Chem.–Eur. J., 2009, 15, 3003–3011 CrossRef CAS PubMed.
- J. Z. Zhang, C. Jia, H. F. Dong, J. Q. Wang, X. P. Zhang and S. J. Zhang, Ind. Eng. Chem. Res., 2013, 52, 5835–5841 CrossRef CAS.
- X. F. Wang, N. G. Akhmedov, Y. H. Duan, D. Luebke and B. Y. Li, J. Mater. Chem. A, 2013, 1, 2978–2982 RSC.
- Y. S. Sistla and A. Khanna, Chem. Eng. J., 2015, 273, 268–276 CrossRef CAS.
- M. Vafaeezadeh, J. Aboudi and M. M. Hashemi, RSC Adv., 2015, 5, 58005–58009 RSC.
- J. Guzmán, C. Ortega-Guevara, R. G. de León and R. Martínez-Palou, Chem. Eng. Technol., 2017, 40, 2339–2345 CrossRef.
- X. Y. Luo, X. Y. Chen, R. X. Qiu, B. Y. Pei, Y. Wei, M. Hu, J. Q. Lin, J. Y. Zhang and G. G. Luo, Dalton Trans., 2019, 48, 2300–2307 RSC.
- P. Brown, B. E. Gurkan and T. A. Hatton, AIChE J., 2015, 61, 2280–2285 CrossRef CAS.
- R. F. Yuan and M. D. Fayer, J. Phys. Chem. B, 2019, 123, 7628–7639 CrossRef CAS PubMed.
- F. K. Wang, Y. Zhang, Y. Gao, P. Luo, J. W. Su, W. Han, K. L. Liu, H. Q. Li and T. Y. Zhai, Small, 2019, 15, 1901347 CrossRef PubMed.
- Y. Y. Jiang, G. N. Wang, Z. Zhou, Y. T. Wu, J. Geng and Z. B. Zhang, Chem. Commun., 2008, 4, 505–507 RSC.
Footnote |
† Electronic supplementary information (ESI) available: Tables S1–S3, Fig. S1–S5, and the data of characterization. See DOI: 10.1039/c9ra09293e |
|
This journal is © The Royal Society of Chemistry 2020 |