DOI:
10.1039/C9RA07709J
(Paper)
RSC Adv., 2020,
10, 4817-4824
Two d10 luminescent metal–organic frameworks as dual functional luminescent sensors for (Fe3+,Cu2+) and 2,4,6-trinitrophenol (TNP) with high selectivity and sensitivity†
Received
23rd September 2019
, Accepted 15th January 2020
First published on 29th January 2020
Abstract
Two luminescent 3D supramolecular structures [Cd3(L)2(2,2-bipy)2](DMF)3(CH3CH2OH)2(H2O) (1) and [Zn3(L)2(2,2-bipy)2(DMF)2](DMF)2(CH3CH2OH)2(H2O) (2) (H3L = 4,4′,4′′-nitrilotribenzoic acid) have been successfully synthesized under solvothermal conditions using Cd(NO3)2·4H2O or Zn(NO3)2·6H2O as the metal sources, and 4,4′,4′′-nitrilotribenzoic acid (H3L), 2,2-bipy as the ligands in DMF solvent. Compound 1 displays a bi-nodal (2,3,6)-coordinated net with {83}2{86·126·163}{8}6 topology, compound 2 can be described as a (3,6)-connected 2-nodal net with kgd topology. The phase purity of compound 1 and 2 is characterized by X-ray powder diffraction (XRPD), thermogravimetric analysis (TGA) and Fourier transform infrared (FT-IR) spectroscopy. Compound 1 and 2 can serve as effective luminescent sensors for Fe3+, Cu2+ and TNP via luminescent quenching.
Introduction
The detection of hazardous explosives and heavy metal ions is of current interest for environmental conservation and national security.1–4 Nitro aromatics such as nitrobenzene (NB), 1,3-dinitrobenzene (1,3-DNT), 2,4,6-trinitrotoluene (TNT) and 2,4,6-trinitrophenol (TNP), are ingredients of industrial explosives which are found in many unexploded land mines worldwide.2,3,5–10 Among these nitro explosives, TNP shows more explosive power compared to TNT. TNP is released into the environment during its commercial production and use, leading to the contamination of soil and aquatic systems. Moreover, picramic acid (2-amino-4,6-dinitrophenol), which is the byproduct of metabolism, has ten times more mutagenic activity than TNP.11–14 Thus, it is very important for the selective and sensitive detection of TNP present in soil and ground water for security screening, homeland security and environmental monitoring.
On the other hand, Fe3+ and Cu2+ ions are not only the most important elements in environmental systems, but also play important roles in biological processes. In particular, Fe3+ ions are of great importance in oxygen uptake and transportation, oxygen metabolism and electron transfer. However, accumulation of Fe3+ ions in the human body would damage biomolecules by generating reactive oxygen species (ROS). Meanwhile, iron deficiency could result in diseases such as anemia and insomnia.15–18 Similarly, exposure to excess Cu2+ ions, which may have resulted from environmental contamination and occupational hazards, could lead to oxidative damage. Excess Cu2+ uptake can result in copper metabolism disorders in the brain and give rise to diseases such as Alzheimer's disease.19–22 Hence, selective detection or sensing of Fe3+ and Cu2+ seems to be very important for human health.
Various traditional methods have been used for monitoring explosives and metal ions including gas chromatography, liquid chromatograph/mass spectrometer, gel chromatography, inductively coupled plasma, atomic absorption spectrophotometry, electrochemical methods, etc.23–27 nevertheless, these detection techniques are limited by their inconvenience of carrying, time-consuming and the like. Compared to the traditional methods, fluorescence detection has obvious advantages and gains more attention owing to its high sensibility, simplicity, short response time, and its ability to be employed both in solution and solid phase. In fact, the materials are usually used for fluorescence detection are still defective in the respects of stability, toxicity, sensitivity, and biodegradability, thus it is a challenging task to synthesize novel materials for fluorescence detection of explosives and metal ions.28–30
Metal–organic frameworks are well known for their potential applications in a wide range of areas such as gas storage, separation, catalysis, sensing, drug delivery, food safety and so on. Among these applications, luminescence metal–organic frameworks (LMOFs) provide several advantages over conventional fluorophores.31–40 Their designable architectures allow improved host–guest interactions and serve as pre-concentrators for target analytes. There are many LMOFs had been developed for the detection of explosives and metal ions, for example, Li et al. reported the first highly LMOF which is able to detect trace amounts of nitroaromatic explosives (NACs) such as 2,4-dinitrotoluene (DNT) and 2,3-dimethyl-2,3-dinitrobutane (DMNB) in the vapor phase.41 Subsequently, a series of LMOF based on transition metal ions or lanthanide were documented for sensing and detection of NACs with a fast and highly sensitive response via partial luminescence quenching, for example, Shi et al. studied the luminescence quenching of Zn3(TDPAT)(H2O)3·G (G = guest solvent) with the addition of nitrobenzene, and its limit detection is 50 ppm.42 Bu et al. reported a highly sensitive luminescent Cd(II)-based MOF that quenched at 100 ppm of TNP and display a high quenching efficiency of 92.5%.43 Ghosh's group demonstrated a luminescent 3D MOF [Cd(NDC)0.5(PCA)]·Gx (G = guest molecules, NDC 2,6-napthalenedicarboxylic acid, PCA = 4-pyridinecaboxylic acid) for the highly selective detection of 2,4,6-trinitrophenol (TNP).44
However, multifunctional sensors based on LMOFs are relatively rare.45–47 Therefore the exploitation of dual functional chemosensor for detecting metal ions (i.e. Fe3+, Cu2+) that are necessary for the human body and nitroaromatic compounds that harm personal and environmental safety is of great significance.
In this article, we are presenting two 3D supermolecular MOF structures [Cd3(L)2(2,2-bipy)2(DMF)3](CH3CH2OH)2(H2O) and [Zn3(L)2(2,2-bipy)2](DMF)3(H2O) (2), which have been utilized as dual luminescent sensor for the selective detection of metal ions (Fe3+,Cu2+) and TNP, and showed high selectivity and sensitivity through luminescence quenching.
Experimental section
Materials and physical measurements
All reagents and solvents were obtained commercially and used without further purification. Elemental analyses were performed on a PerkinElmer 2400 element analyzer. IR spectra were recorded on a Nicolet Impact 410 FTIR spectrometer using KBr pellet. PXRD data were collected on a Rigaku D/max 2550 X-ray Powder Diffractometer. TGA was measured using thermogravimetric experiments, which were performed with a TGA Q500 V20.10 Build 36; data were collected from room temperature to 800 °C at a heating rate of 10 °C min−1 in a flowing N2 atmosphere.
Synthesis of compound 1
Cd(NO3)2·4H2O (0.1 mmol, 30.8 mg), H3L (0.05 mmol, 18.8 mg), and 2,2′-bipy (0.1 mmol, 15.6 mg) were dissolved in DMF (6 mL). The resulting reaction mixture was stirred at room temperature for 30 min. Then it was transformed to a 15 mL teflon-lined stainless steel vessel and heated at 100 °C for three days. After this time block brown crystals of 1 were obtained in 57% yield (based on H3L), IR (KBr 4000–400 cm−1) 3126 (w), 1669 (s), 1591 (s), 1384 (s), 1172 (m), 1081 (m), 1017 (m), 838 (s), 784 (s), 668 (m), 636 (m), 539 (w), 442 (w). Elemental analysis (%): calcd for: C75H75Cd3N9O18: C 52.10 H 4.34 N 7.29; found: C 52.15 H 4.27 N 7.32.
Synthesis of compound 2
A mixture of Zn(NO3)2·6H2O (0.1 mmol, 29.1 mg), H3L (0.05 mmol, 18.8 mg), 2,2-bipyridine (0.1 mmol, 15.6 mg), DMF (6 mL) were stirred at room temperature for 10 min, then sealed in a 15 mL teflon-lined stainless steel vessel, and heated at 100 °C for three days, after this time columnar colorless crystals of 2 were obtained in 50% yield (based on H3L), IR (KBr 4000–400 cm−1) 3469 (w), 3056 (w), 1688 (s), 1585 (s), 1391 (s), 1165 (m), 1101 (m), 849 (w), 778 (s), 668 (m), 520 (s), 436 (w). Elemental analysis (%): calcd for: C74H70Zn3N10O17: C 56.69 H 4.47 N 8.94; found: C 56.73 H 4.51 N 8.97.
X-ray crystallography
The data collection and structural analysis were performed on a Rigaku RAXIS-RAPID equipped with a narrow-focus, 5.4 kW sealed tube X-ray source (graphite-monochromated Mo Kα radiation, λ = 0.71073 Å). The data were collected at a temperature of 20 ± 2 °C. The data processing was accomplished with the PROCESS-AUTO processing program. The structures were solved with the direct methods of SHELXL crystallographic software package and refined on F2 by full-matrix least square techniques. All non-hydrogen atoms of the two compounds were refined with anisotropic thermal parameters. All hydrogen atoms of the organic molecule were geometrical placed and added to the structure factor calculation. CCDC-1580391 (1) and CCDC-1813685 (2) contain the supplementary crystallographic data for this paper.† Basic information pertaining to crystal parameters and structure refinement is summarized in Table 1. As for the molecular formula of as synthesized compounds 1 and 2, it is difficult to obtain the exact solvent molecules in the structure, so we further determined it using Platon/Squeeze, TGA, IR and elemental analysis.
Table 1 Crystallographic data and structure refinement summary for compounds 1, 2
Compound |
1 |
2 |
R1 = ||Fo| − |Fc||/Σ|F|o. wR2 = [Σw(Fo2 − Fc2)2/Σw(Fo2)2]1/2. w = 1/[σ2(Fo2) + (ap)2 + (bp)], p = [max(Fo2 or 0) + 2(Fc2)]/3. |
Molecular formula |
C75H75Cd3N9O18 |
C74H70Zn3N10O17 |
Formula weight |
1727.23 |
1566.23 |
Crystal system |
Monoclinic |
Triclinic |
Space group |
C2/c |
P![[1 with combining macron]](https://www.rsc.org/images/entities/char_0031_0304.gif) |
a, Å |
14.005(3) |
13.3539(7) |
b, Å |
22.005(4) |
13.6334(7) |
c, Å |
23.006(5) |
13.6928(7) |
V, Å3 |
7083(3) |
2164.6(2) |
Z |
4 |
1 |
Dcalc, g cm−3 |
1.311 |
1.077 |
F(000) |
2776.0 |
720.0 |
GoF |
1.086 |
1.085 |
R1, wR2 [I > 2σ(I)]a,b |
R1 = 0.0564 |
R1 = 0.0501 |
wR2 = 0.1556 |
wR2 = 0.1247 |
R1, wR2 (all data) |
R1 = 0.039 |
R1 = 0.0844 |
wR2 = 0.0836 |
wR2 = 0.1141 |
Fluorescence experiments
The synthesized sample compound 1 and 2 were immersed in methanol for 24 h, and the extract was decanted. Fresh methanol was subsequently added, and the crystals were allowed to stay for an additional 24 h to remove the methanol solvents. After the removal of methanol by decanting, the sample was activated by drying under a dynamic vacuum at 373 K overnight to obtain the activated samples. The fluorescence experiments of compound 1a and 2b were investigated in the solid state and in different solvent emulsions, such as DMF, DMSO, DMA, ethanol, methanol, acetonitrile, water and 1,4-dioxane. The concentration for the suspension was fixed at 3.0 mg compound 1a or 2b per 3.0 mL solvents. The luminescence intensities were measured under an excitation of 330, 335 nm for compound 1a and 2b respectively. As for the metal ion sensing experiments, M(NO3)x (M = Cd2+, Co2+, Cu2+, Fe3+, K+, Na+, Pb2+, Zn2+) were separately added to DMF suspension of compound 1a or 2b. The resulted concentration for the metal ions was 10−2 mol L−1 and the mixtures were treated by ultra-sonication for 1 h to form a stable suspension. The fluorescence spectra were recorded for the suspensions. For the fluorescence experiments, the solution of Fe(NO3)3 ([M] = 10−3 mol L−1), Cu(NO3)3 ([M] = 10−3 mol L−1), TNP ([M] = 10−3 mol L−1) were added gradually into compound 1a or 2b DMF suspension, respectively. The fluorescent spectra of the samples were recorded on an Edinburgh Instruments FLS920 spectrofluorimeter equipped with both continuous (450 W) and pulsed xenon lamps.
Results and discussion
Description of crystal structures
Single crystal X-ray crystallographic analysis reveals that compound 1 crystallizes in the monoclinic space group C2/c. In this structure, the asymmetric unit of 1 consists of two independent Cd atoms, one L3− ligand, one 2,2-bipy molecule (Fig. 1a). The Cd1 atom is coordinated by four carboxylate O atoms from three L3− ligands, and two N atoms from one 2,2-bipy molecule, adopting an octahedral coordination geometry, Cd1–N and Cd1–O are in the range of 2.314–2.321 Å and 2.186–2.444 Å, respectively, the Cd2 atom is six-coordinated by six carboxylate O atoms from six L3− ligands, has a distorted octahedral coordination geometry (Cd2–O = 2.228–2.298 Å) (Fig. 1b).48–50 In particular, Cd1, Cd2 atoms are united together through carboxylate groups of L3− ligands to give a central symmetric trinuclear Cd(II) cluster (Cd⋯Cd separation, 3.380–6.760 Å), within the cluster, the Cd2 octahedron is bridged to two symmetry related Cd1 in a corner-sharing manner, each cluster is linked by six L ligands to form a unique 2D bilayered framework containing quadrangle grids with an approximate dimension of 7.0 Å × 8.8 Å (Fig. 1c). The adjacent bilayers adopt an ABAB mode, with further packing into a 3D supramolecular structure (Fig. 1d), it is noteworthy that π–π interactions between the adjacent 2D layers stabilize the coordination structure. To get deep insight into the structure of compound 1, topological analysis was carried out. The Cd atom is treated as six-connected nodes, and the L3− ligands are defined as the three-connected nodes, 2,2-bipyridine is considered as two-connected nodes, the layer is simplified as a bi-nodal (2,3,6)-coordinated net with the point symbol {83}2{86·126·163}{8}6 (Fig. 2a), the calculated solvent accessible volume was 2186.6 Å3 (unit cell volume of 7083.0 Å3), and the porosity was as high as 30.9% by using PLATON.
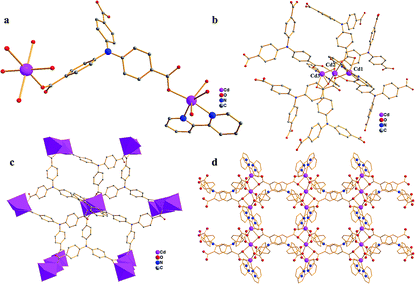 |
| Fig. 1 (a) The asymmetric unit of compound 1; (b) the coordination sphere of Cd atoms in compound 1; (c) the 2D layer of compound 1; (d) the 3D supramolecular structure of compound 1 (purple, Cd; red, O; blue, N; gray, C). | |
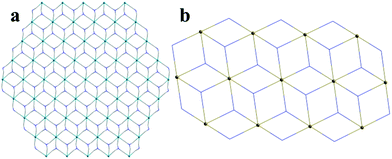 |
| Fig. 2 (a) The topology of compound 1; (b) the topology of compound 2. | |
Single crystal X-ray diffraction analysis reveals that compound 2 crystallizes in the triclinic P
space group. As shown in Fig. 3a, the asymmetric unit of 2 contains one Zn(II) ion, one L3− unit, one 2,2-bipyridine molecule, and one DMF molecule. Zn1 atom is coordinated by four O atoms from three L3− ligands, two N atoms from one 2,2-bipyridine molecule, showing a distorted octahedral geometry (Zn–O = 1.984–2.381 Å, Zn–N = 2.114–2.188 Å). The Zn2 ion has a six coordinated octahedral geometry which is defined by six O atoms from four L3− ligands (Zn–O = 2.018–2.160 Å), while Zn3, similar to Zn1, exhibits a distorted octahedral geometry which is formed by four O atoms from three L3− ligands and two N atoms from one 2,2-bipyridine molecule(Zn–O = 1.984–2.381 Å, Zn–N = 2.114–2.188 Å).49,52 Zn1, Zn2 and Zn3 are associated together by six carboxylate groups of six H3L ligands to form a trinuclear [Zn3(CO2)6] unit, which can be thought of as the secondary building unit (SBU) of the network (Fig. 3b), with distances of Zn1–Zn2 3.4187 Å and Zn1–Zn3 6.836 Å. It is interesting to note that the L3− ligands show two different coordination modes (La and Lb), La adopts a μ6-η1:η1:η1:η2:η1:η1 bridging coordination mode to connect six ZnII ions; meanwhile Lb adopts a μ5-η1:η1:η1:η1:η1:η1 bridging coordination mode to connect six ZnII ions (Scheme 1). The L3− ligands link three adjacent trinuclear Zn(II) cluster to form a 2D sheet with quadrilateral lattices, with a cavity dimension of 8.5 Å × 9.6 Å running along b axis (regardless of the van der Waals radii, Fig. 3c), Furthermore, the 2D layers are further linked into a 3D supramolecular architecture through π–π stacking interactions between the 2,2-bipy ligands. Without guest molecules, the total accessible volume in compound 2 was estimated to be 36.4% using PLATON software.12
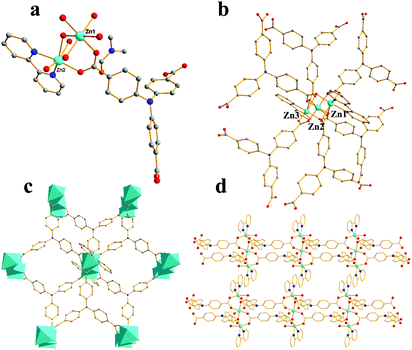 |
| Fig. 3 (a) The asymmetric unit of compound 2; (b) the coordination sphere of Zn atom in compound 2; (c) the 2D layer of compound 2; (d) the 3D supramolecular structure of compound 2 (turquoise, Zn; red, O; blue, N; gray, C). | |
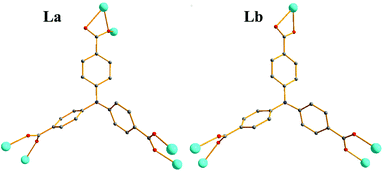 |
| Scheme 1 Coordination modes of L3−. | |
To better understand the structure of compound 2, the topological analysis approach was employed. If defining the L3− ligands as three-connected nodes and the trinuclear Zn(II) cluster as 6-connected nodes, the whole 2D framework can be described as a (3,6)-connected 2-nodal net with a Schläfli symbol of {43}2{46·66·83}, display the kgd topology (Fig. 2b).
Luminescent emission
MOFs have been investigated for fluorescence properties owing to their application in the area of luminescent materials, especially for the compounds with d10 metal centers. To further examine the solid state luminescent properties of the d10 metal complexes, we measured the solid state fluorescent spectrum of the H3L ligand and the two compounds at room temperature, which are depicted in Fig. 4. The free ligand H3L displays fluorescence with an emissions maximum at 448 nm (λex = 330 nm), compound 1 and 2 show fluorescence with emissions maximum at 532 nm and 528 nm respectively (1: λex = 330 nm, 2: 335 nm), which has the red shift of 84 or 80 nm, compared with the free H3L ligand. With Zn(II) and Cd(II) as a typical d10 configuration, the observed fluorescence emissions should be assigned to ligand-to-metal charge transfer (LMCT), metal-to-ligand charge transfer (MLCT), or intraligand (π* → n/π* or π* → π) emission. The luminescent emission of MOFs is influenced by both central metal coordination and the property of coordinated ligands, which need to be considered when attempting to assemble new functional luminescent MOFs.51,52
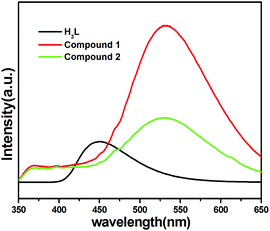 |
| Fig. 4 Solid-state fluorescent spectral of H3L and compound 1a, 2b. | |
The luminescence of the two MOFs encouraged us to examine their potentials for sensing solvent molecules. The fluorescence properties of compound 1a and 2b in different solvent emulsions were investigated. The solvents used were DMF, DMSO, DMA, ethanol, methanol, acetonitrile, water and 1,4-dioxane. The fluorescent spectra of the two compounds (3 mg), suspended in the different solvents (3 mL), were measured. As shown in Fig. 5a and b, the emission intensities were dependent on the solvent, meanwhile, there are strong luminescence emissions in DMF solvent compared with other solvents in the two compounds.
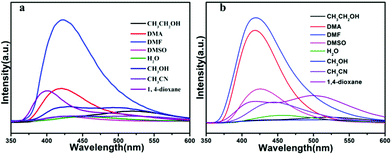 |
| Fig. 5 (a) Fluorescent spectral of compound 1a in different solvent; (b) fluorescent spectral of compound 2b in different solvent. | |
Owing to their good fluorescent performance, compound 1a and 2b were selected to further study their sensing properties towards various metal ions in DMF solvent. The activated samples 1a or 2b (3 mg) were ground into powder, and dispersed in 3 mL of 10−2 M suspensions of metal ions: M(NO3)x (M = Cd2+, Co2+, Cu2+, Fe3+, K+, Na+, Pb2+, Zn2+), and then the luminescence experiment was conducted. As depicted in Fig. 6a and b. The solutions that contain different species of metal ions displayed remarkably different luminescent intensities. The results show that the metal ions of Na+, Zn2+ can enhance the fluorescence intensity of activated 1a with the enhancing percentage of 33%, 35% respectively, we explored the sensitivity of compound 1 for them, after 1000 μL addition of Na+ or Zn2+ (0.01 M) to a 3.0 mL suspension of activated 1a, it was found that the emission intensity only slightly increased with the enhancing percentage of 4.6% or 4.3%. Meanwhile, part of the metal ions (Cd2+, Na+ and Zn2+) can enhance the fluorescence intensity of activated 2b with the enhancing percentage of 13%, 15.5% and 16.3%, respectively, to further explore the enhancing efficiency of Na+, Zn2+ and Cd2+ towards activated 2b, we added 1000 μL Cd2+, Na+ and Zn2+ (0.01 M) to a 3.0 mL suspension of activated compound 2, it was found that the emission intensity slightly increased with the enhancing percentage of 9.3%, 1.86%, 5.4% for Cd2+, Na+, Zn2+. The result showed that the quenching effect of these ions towards activated 1a and 2b is subtle (Fig. S1 and S2†). As to activated 1a and 2b, The other metal ions exhibit different fluorescence quenching efficiency, and the quenching efficiency of compound 1 was calculated to be 16.9, 83.6, 98.9, 99.8, 45.3, 85.8% for Cd2+, Co2+, Cu2+, Fe3+, K+ and Pb2+. For compound 2, it is estimated that the quenching efficiency is 17.6, 89.8, 93.2, 100, 99.6% for Co2+, Cu2+, Fe3+, K+ and Pb2+. In particular, Fe3+ and Cu2+ exhibit an excellent quenching effect with sharply decreased luminescence intensities towards activated compound 1 and 2, indicating that they can act as promising selective sensor. The possible mechanism of fluorescence quenching is discussed, although framework collapse is always a common way to quench the fluorescence, but as indicated for the PXRD of compound 1a and 2b (Fig. S3†), the framework retained its structural integrity after adding into metal ions. Hence, the reason for fluorescence quenching is probably that the interaction between metal ions and the framework changes the electron energy level of ligand, resulting in the inefficient energy transfer between ligand and metal ions.53,54 In order to further evaluate the fluorescence sensing selectivity performance of the activated samples 1a and 2b towards Fe3+ and Cu2+, all solid samples (30 mg) were added into 30 mL DMF and got the well-dispersed suspension of probes. Fe3+ or Cu2+ solution was prepared by dissolving Fe(NO3)3 or Cu(NO3)2 in DMF to obtain the desired concentrations (10−3 M), the Fe3+ or Cu2+ ion sensing experiments were performed by adding diverse amounts of above Fe3+ or Cu2+ ions solution to a quartz cuvette containing 3 mL of DMF suspension of probes and then detected by the PL spectra. The emission intensity was monitored after each addition, the luminescence emission intensity of activated 1a and 2b suspended in DMF at 430 nm was leveled off sequentially as a result of gradually increased the volume of Fe3+ solution. As shown in Fig. 7a, for the Fe3+ towards compound 1, when the volume of Fe3+ increased to 700 μL, which displays a prominent quenching effect with an efficiency of 100%. Meanwhile, in Fig. 7b, when the volume of Fe3+ increased to 1000 μL, the fluorescence emission of activated compound 2 is quenched completely, this quenching effect can be rationalized by the Stern–Volmer equation: I0/I = 1 + Ksv × [M], where the values I0 and I are the luminescence intensity of the activated compound 1 or 2 without and with addition of Fe3+ solution, respectively, Ksv is the quenching constant, [M] is the Fe3+ concentration. On the basis of the experimental data in Fig. 7a and b, the linear correlation coefficient (R2) in the Ksv curves of activated 1a and 2b are 0.991, 0.995 respectively, which suggests that the quenching effect of Fe3+ on the luminescence of activated 1a and 2b fit the Stern–Volmer mode well. The Ksv value is calculated as 15.5 ×104 M−1 and 1.96 ×104 M−1 (Fig. S4 and S6†), which reveals a strong quenching effect on the luminescence of the compounds.
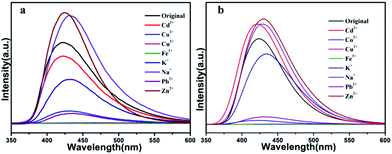 |
| Fig. 6 (a) Fluorescent spectral of compound 1a in different metal ions (10−2 M); (b) fluorescent spectral of compound 2b in different metal ions (10−2 M). | |
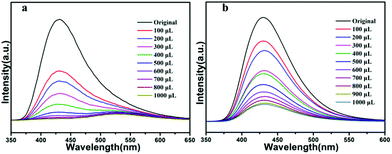 |
| Fig. 7 (a) Fluorescent spectral of compound 1a dispersed in DMF upon progressive addition of Fe3+ solution (10−3 M), and the quenching of the original emission and the appearance of a new peak for compound 1; (b) fluorescent spectral of compound 2b dispersed in DMF upon progressive addition of Fe3+ solution (10−3 M). | |
With the addition of Cu2+ ions, the fluorescence intensity of activated 1 and 2 decrease gradually (Fig. 8a and b). When the concentration of Cu2+ ions reaches 1000 μL for activated 1, the quenching efficiency is 74.2%, and for 2, when the concentration of Cu2+ ions reaches 1000 μL the quenching efficiency is 92.6%, which from S–V curve, the linear correlation coefficient in the Ksv curves of activated compound 1 and 2 are 0.9612, 0.964 respectively, the value of Ksv is calculated as 1.339 × 104 M−1 and 5.64 × 104 M−1 (Fig. S5 and S7†), as far as we know, the Ksv value is larger than other reported sensors, that is to say, activated 1a and 2b can not only detect of Cu2+ ions, but also show high sensitivity.55–57
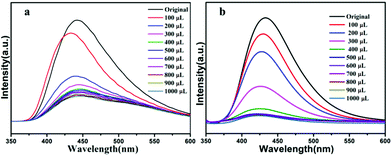 |
| Fig. 8 (a) Fluorescent spectral of compound 1a dispersed in DMF upon progressive addition of Cu2+ solution (10−3 M); (b) fluorescent spectral of compound 2b dispersed in DMF upon progressive addition of Cu2+ solution (10−3 M). | |
Fortunately, the luminescence intensity of activated 1a and 2b were easily recovered centrifuging the suspensions after sensing Fe3+ or Cu2+ ions, and washing more than three times using DMF solvent. Significantly, the samples can be regenerated, the framework retained its structural integrity, and reused for five cycles and the quenching efficiency of the five cycles maintain high values (Fig. S12a, b, S13a and b†). PXRD pattern of the original is completely consistent with the recovered samples with five times of quenching and recovery (Fig. S10a, b, S11a and b†). The results indicate that activated 1a and 2b can act as promising chemical sensors for Fe3+ and Cu2+ ions. The mechanism of luminescence quenching caused by Fe3+ and Cu2+ might result from interactions between the cations and the framework or central metals.
The nitro aromatic compounds (NACs) are important chemical reactants and are extensively applied in chemical industry, which can cause explosions and environmental damage. Therefore, it is important for fast and effective detection of NACs. As shown from Fig. 9a, the 4-NT, 2-NT, NB have weak quenching efficiency, and 2,6-DNT has weak enhanced luminescent intensities for compound 1, and 2,6-DNT, and 4-NT, 2-NT, NB, 2,6-DNT have different quenching efficiency for compound 2 in Fig. 9b, meanwhile, preliminary studies show TNP have good quenching efficiency compared with other NACs for both 1 and 2. For further study the luminescent quenching efficiency of TNP, the fluorescence sensing experiments were carried out by gradual addition of 10−3 M solutions of TNP. As shown in Fig. 10a and b, the emission band of compound 1a and 2b both undergoes a bathochromic shift (Δλ = 50 nm), which can be ascribed to the energy transfer between electron-rich compound 1 or 2 and electron-deficient TNP.58,59 the quenching efficiency is 100% at 425 nm when TNP addition reached to 1000 μL for 1 and 2. To further understand the luminescent quenching degree, the quenching curves were quantitatively studied by the Stern–Volmer equation: I0/I = 1 + Ksv × [M], the S–V plots exist good linear relationship between the luminescence intensities and the concentration of TNP, and linear correlation coefficient of compound 1 and 2 are up to 0.975, 0.977, according to the S–V equation, the Ksv values are up to 2.46 × 104 M−1 and 11.76 × 104 M−1 for TNP (Fig. S8 and S9†), in addition, in order to study whether the complex can be used as stability material, the dispersed solution was centrifuged after recycled use more than three times. As shown in Fig. S12c and S13c,† compound 1 and 2 can be restored and recycle at least five times. At the same time, the PXRD pattern we tested show good performance compared with the original materials compound 1a and 2b (Fig. S10c and S11c†).
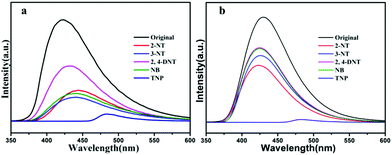 |
| Fig. 9 (a) The fluorescent spectral of 1a dispersed in 10−2 M different DMF solutions of NACs; (b) the fluorescence spectral of 2b dispersed in 10−2 M different DMF solutions of NACs. | |
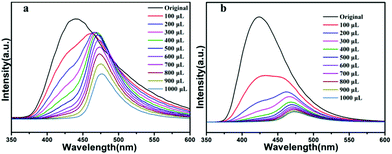 |
| Fig. 10 (a) Fluorescent spectral of compound 1a dispersed in DMF upon progressive addition of 10−3 M TNP solution; (b) fluorescent spectral of compound 2b dispersed in DMF upon progressive addition of 10−3 M TNP solution. | |
Conclusions
Two new 3D supramolecular structures [Cd3(L)2(2,2-bipy)2(DMF)3(CH3CH2OH)2(H2O) (1) and [Zn3(L)2(2,2-bipy)2](DMF)3(H2O) (2) based on a fluorescent ligand H3L have been assembled and completely characterized, compound 1 displays a bi-nodal (2,3,6)-coordinated net with {83}2{86·126·163}{8}6 topology, compound 2 shows a (3,6)-connected 2-nodal net of kgd topological type. The luminescent properties of the two compounds are measured and investigated in detail, the sensing ability of the two compounds toward metal ions and NACs is studied, revealing their high detection ability to sense Fe3+/Cu2+ or TNP via luminescence quenching. Hence, the present study demonstrates that the two compounds have the potential applications as dual functional fluorescent sensors for metal ions and NACs.
Conflicts of interest
There are no conflicts to declare.
Acknowledgements
This project is supported by Open Project of State Key Laboratory of Inorganic Synthesis and Preparation of Jilin University 2017-35, Research Fund for the Doctoral Program of Weifang University (2016BS06), High-tech Industrial Development Zone Science and Technology Huimin Plan of Weifang (2019KJHM18), Natural Science Foundation of Shandong Province of China (ZR2017MB056), National Natural Science Foundation Youth Fund 201802104, Shandong Provincial Natural Science Foundation (ZR2018MB007), the Science and Technology Program of Weifang (2017GX014) and Open Project Program of Shandong Key Laboratory of Biochemical Analysis (QUSTHX201802).
Notes and references
- X. Fang, B. Y. Zong and S. Mao, Nano-Micro Lett., 2018, 1–19 Search PubMed.
- S. A. Diamantis, A. Margariti, A. Pournara, G. S. Papaefstathiou, M. J. Manos and T. Lazarides, Inorg. Chem. Front., 2018, 5, 1493–1511 RSC.
- Z. C. Hu, B. J. Deibert and J. Li, Chem. Soc. Rev., 2014, 43, 5815–5840 RSC.
- B. Y. Li, Y. M. Zhang, D. X. Ma, Z. Shi and S. Q. Ma, Nat. Commun., 2014, 5(5537), 1–7 Search PubMed.
- W. F. Xu, H. H. Chen, Z. Q. Xia, C. T. Ren, J. Han, W. J. Sun, Q. Wei, G. Xie and S. P. Chen, Inorg. Chem., 2019, 58, 8198–8207 CrossRef PubMed.
- C. Liu, X. J. Bo and L. P. Guo, Sens. Actuators, B, 2019, 297, 126741 CrossRef CAS.
- X. D. Yu, G. B. Guo, P. Peng, F. J. Shen, Y. J. Li, L. J. Geng and T. Wang, Appl. Surf. Sci., 2019, 487, 473–479 CrossRef CAS.
- L. N. Wang, Y. H. Zhang, S. Jiang and Z. Z. Liu, CrystEngComm, 2019, 21, 4557–4567 RSC.
- J. H. Wei, J. W. Yi, M. L. Han, B. Li, S. Liu, Y. P. Wu, L. F. Ma and D. S. Li, Chem.–Asian J., 2019, 14, 3694–3701 CrossRef CAS PubMed.
- W. Wang, J. Yang, R. M. Wang, L. L. Zhang, J. F. Yu and D. F. Sun, Cryst. Growth Des., 2015, 15, 2589–2592 CrossRef CAS.
- S. N. Zhao, X. Z. Song, M. Zhu, X. Meng, L. L. Wu, S. Y. Song, C. Wang and H. J. Zhang, RSC Adv., 2015, 5, 93–98 RSC.
- E. L. Zhou, P. Huang, C. Qin, K. Z. Shao and Z. M. Su, J. Mater. Chem. A, 2015, 3, 7224–7228 RSC.
- K. M. Wollin and H. H. Dieter, Arch. Environ. Contam. Toxicol., 2005, 49, 18–26 CrossRef CAS PubMed.
- K. W. Hofmann, H. J. Knackmuss and G. Heiss, Arch. Environ. Contam. Toxicol., 2004, 70, 2854–2860 CAS.
- C. H. Chen, X. S. Wang, L. Li, Y. B. Huang and R. Cao, Dalton Trans., 2018, 47, 3452–3458 RSC.
- F. L. Hu, Y. X. Shi, H. H. Chen and J. P. Lang, Dalton Trans., 2015, 44, 18795–18803 RSC.
- B. B. Andrea, A. M. Costero, G. Salvador, P. Margarita, J. Soto, M. M. Ramon and F. Sancenonz, Chem. Commun., 2012, 48, 3000–3002 RSC.
- J. L. Bricks, A. Kovalchuk, C. Trieflinger, N. Marianne, B. Michael, A. I. Tolmachev, J. Daub and K. Rurack, J. Am. Chem. Soc., 2005, 127, 13522–13529 CrossRef CAS PubMed.
- H. Li, Y. J. Li, Z. Zhang, X. L. Pang and X. D. Yu, Mater. Des., 2019, 172, 107712 CrossRef CAS.
- Y. Y. Liang, L. J. Luo, Y. Li, B. K. Ling, B. W. Chen, X. W. Wang and T. G. Luan, Eur. J. Inorg. Chem., 2019, 206–211 CrossRef CAS.
- Y. L. Wu, G. P. Yang, Y. Q. Zhao, W. P. Wu, B. Liu and Y. Y. Wang, Dalton Trans., 2015, 44, 3271–3277 RSC.
- L. M. Gaetke and C. K. Chow, Toxicology, 2003, 189, 147–163 CrossRef CAS PubMed.
- M. Petrovic, M. Farréa, M. L. Aldaa, S. Perez, C. Postigo, K. Marianne, J. Radjenovic, G. Merixell and D. Barcelo, J. Chromatogr. A, 2010, 1217, 4004–4017 CrossRef CAS PubMed.
- Z. O. Tesfaldet, J. F. Staden and R. I. Stefan, Talanta, 2004, 64, 1189–1195 CrossRef CAS PubMed.
- K. Håkansson, R. V. Coorey, R. A. Zubarev and V. L. Talrose, J. Mass Spectrom., 2000, 35, 337–346 CrossRef.
- A. C. Liu, D. C. Chen, C. C. Lin, H. H. Chou and C. H. Chen, Anal. Chem., 1999, 71, 1549–1552 CrossRef CAS.
- V. A. Elrod, K. S. Johnson and K. H. Coale, Anal. Chem., 1991, 63, 893–898 CrossRef CAS.
- M. Saleem and K. H. Lee, RSC Adv., 2015, 5, 72150–72287 RSC.
- P. Kovacic and R. Somanathan, J. Appl. Toxicol., 2014, 34, 810–824 CrossRef CAS PubMed.
- M. Formica, V. Fusi, L. Giorgi and M. Micheloni, Coord. Chem. Rev., 2012, 256, 170–192 CrossRef CAS.
- G. M. Espallargas and E. Coronado, Chem. Soc. Rev., 2018, 47, 533–557 RSC.
- H. X. Liang, X. L. Jiao, C. Li and D. R. Chen, J. Mater. Chem. A, 2018, 6, 334–341 RSC.
- P. L. Wang, L. H. Xie, E. A. Joseph and J. R. Li, Chem. Rev., 2019, 119, 10638–10690 CrossRef CAS PubMed.
- Z. X. Kang, L. L. Fan and D. F. Sun, J. Mater. Chem. A, 2017, 5, 10073–10091 RSC.
- A. H. Chughtai, N. Ahmad, H. A. Younus, A. Laypkov and F. Verpoort, Chem. Rev., 2015, 44, 6804–6849 CAS.
- J. W. Liu, L. F. Chen, H. Cui, J. Y. Zhang, L. Zhang and C. Y. Su, Chem. Soc. Rev., 2014, 43, 6011–6061 RSC.
- R. B. Getman, Y. S. Bae, C. E. Wilmer and R. Q. Snurr, Chem. Rev., 2012, 112, 703–723 CrossRef CAS PubMed.
- J. R. Li, J. Sculley and H. C. Zhou, Chem. Rev., 2012, 112, 869–932 CrossRef CAS PubMed.
- Y. J. Cui, Y. F. Yue, G. D. Qian and B. L. Chen, Chem. Rev., 2012, 112, 1126–1162 CrossRef CAS PubMed.
- A. Corma, H. García and F. X. Liabrés i Xamena, Chem. Rev., 2010, 110, 4606–4655 CrossRef CAS PubMed.
- A. J. Lan, K. H. Li, H. H. Wu, D. H. Olson, T. G. Emge, W. Ki, M. C. Hong and J. Li, Angew. Chem., Int. Ed., 2009, 48, 2334–2338 CrossRef CAS PubMed.
- D. X. Ma, B. Y. Li, X. J. Zhou, Q. Zhou, K. Liu, G. Zeng, G. H. Li, Z. Shi and S. H. Feng, Chem. Commun., 2013, 49, 8964–8966 RSC.
- D. Tian, Y. Li, R. Y. Chen, Z. Chang, G. Y. Wang and X. H. Bu, J. Mater. Chem. A, 2014, 2, 1465–1470 RSC.
- S. S. Nagarkar, B. Joarder, A. K. Chaudhari, S. Mukherjee and S. K. Ghosh, Angew. Chem., Int. Ed., 2013, 52, 2881–2885 CrossRef CAS PubMed.
- J. Wang, J. Wu, L. Lu, H. J. Xu, M. Trivedi, A. Kumar, J. Q. Liu and M. B. Zheng, Front. Chem., 2019, 7 DOI:10.3389/fchem.2019.00244.
- E. L. Zhou, C. Qin, D. Tian, X. L. Wang, B. X. Yang, L. Huang, K. Z. Shao and Z. M. Su, J. Mater. Chem. C, 2018, 6, 7874–7879 RSC.
- J. S. Hu, T. T. Cheng, S. J. Dong, C. H. Zhou, X. H. Huang and L. Zhang, Microporous Mesoporous Mater., 2018, 272, 177–183 CrossRef CAS.
- Z. Z. Shi, Z. R. Pan, H. L. Jia, S. G. Chen, L. Qin and H. G. Zheng, Cryst. Growth Des., 2016, 16, 2747–2755 CrossRef CAS.
- L. Hu, X. J. Hong, X. M. Lin, J. Lin, Q. X. Cheng and B. Lokesh, Cryst. Growth Des., 2018, 18, 7088–7093 CrossRef CAS.
- X. J. Zhou, B. Y. Li, G. H. Li, Q. Zhou, Z. Shi and S. H. Feng, CrystEngComm, 2012, 14, 4664–4669 RSC.
- Z. H. Yan, W. Wang, L. L. Zhang, X. W. Zhang, L. Wang and D. F. Sun, RSC Adv., 2015, 5, 16190–16198 RSC.
- J. G. Lin, S. Q. Zang, Z. F. Tian, Y. Z. Li, Y. Y. Xu, H. Z. Zhu and Q. J. Meng, CrystEngComm, 2007, 9, 915–921 RSC.
- Y. P. Li, X. H. Zhu, S. N. Li, Y. C. Jiang, M. C. Hu and Q. G. Zhai, ACS Appl. Mater. Interfaces, 2019, 11, 11338–11348 CrossRef CAS PubMed.
- J. C. Jin, L. Y. Pang, G. P. Yang, L. Hou and Y. Y. Wang, Dalton Trans., 2015, 44, 17222–17228 RSC.
- J. Y. Liang, G. P. Li, R. C. Gao, N. N. Bai, W. Q. Tong, L. Hou and Y. Y. Wang, Cryst. Growth Des., 2017, 17, 6733–6740 CrossRef CAS.
- J. M. Zhou, W. Shi, H. M. Li, H. Li and P. Cheng, J. Phys. Chem. C, 2014, 118, 416–426 CrossRef CAS.
- Y. Q. Xiao, Y. G. Cui, Q. Zheng, S. C. Xiang, G. D. Qian and B. L. Chen, Chem. Commun., 2010, 46, 5503–5505 RSC.
- A. Gogia and S. K. Mandal, Dalton Trans., 2019, 48, 2388–2398 RSC.
- S. Mukherjee, A. V. Desai, B. Manna, A. I. Inamdar and S. K. Ghosh, Cryst. Growth Des., 2015, 15, 4627–4634 CrossRef CAS.
Footnote |
† Electronic supplementary information (ESI) available: Selected bond distances and angles (Table S1 and S2), Stern–Volmer plots of compounds 1 and 2 (Fig. S1–S6), the PXRD data of compounds 1 and 2 (Fig. S7 and S8), IR of compounds 1 and 2 (Fig. S9 and S10), TGA of compound 1 and 2 (Fig. S11), crystallographic data in CIF. CCDC 1580391 (1) and 1813685 (2). For ESI and crystallographic data in CIF or other electronic format see DOI: 10.1039/c9ra07709j |
|
This journal is © The Royal Society of Chemistry 2020 |