DOI:
10.1039/D0QM00255K
(Review Article)
Mater. Chem. Front., 2020,
4, 1930-1953
Biosafety materials: an emerging new research direction of materials science from the COVID-19 outbreak
Received
21st April 2020
, Accepted 8th May 2020
First published on 12th May 2020
Abstract
The coronavirus disease 2019 (COVID-19) pandemic is a serious biosafety event that is causing a severe impact on the global society and economy. Thus, the importance of biosafety is once again being valued worldwide. Due to the outbreak of COVID-19, most national governments have been encouraged to speed up the development of biosafety, which places higher requirements on researchers in biosafety and relevant fields. Many problems have been exposed due to the outbreak of COVID-19, including unavailability of effective drugs and vaccines, difficulty in fast or real-time virus detection, insufficient protective equipment, and shortage of transportation equipment for infected patients. To a certain extent, these biosafety problems are partly due to the limited biosafety-related research in materials science. Currently, the tremendous research efforts in materials science around the world have provided a wide variety of materials with peculiar properties to solve biosafety problems. This review attempts to give a perspective on how the development of novel materials can help scientists tackle the challenges in biosafety. Considering the importance of materials science in the biosafety field, it is urgent for us to officially propose the brand new concept of “biosafety materials”, which can be a future scientific discipline that utilizes materials science and theory simultaneously to produce materials, related products, and equipment to solve biosafety problems. Herein, we have aimed to draw worldwide attention on the new discipline of biosafety materials and the active cooperation between materials scientists and biosafety-related scientists to propel its development.
1. Biosafety: in the spotlight once again
1.1 The outbreak of COVID-19: a reconsideration of the importance of biosafety
The rapid development of modern biotechnology and the process of economic globalization have resulted in a series of biosafety issues, such as the escape of genetically modified organisms, invasion of alien species, and the global outbreak of infectious diseases, which pose a huge threat to the diversity of species, ecological environment and human society.1 To date, the continued deterioration of the COVID-19 pandemic has severely affected the society and economic development around the world.2–4 In response to the unprecedented challenges from the COVID-19 pandemic, the Chinese government immediately decided to include biosafety in its national security system, which brings the concept of “biosafety” into the spotlight again.5
The anthrax envelope bioterrorism attack resulted in several people being infected after the event of September 11, 2001.6,7 In 2014, the occurrences of laboratory safety accidents such as the infection of Bacillus anthracis and H5N1 influenza virus were mainly due to poor awareness of biosafety.8–10 Furthermore, multiple consecutive reports of biosafety events have attracted international attention to biosafety issues. Currently, the outbreak of COVID-19 brings biosafety to the forefront of people's consciousness, which fully strengthens the necessity for the related scientific research in the field of biosafety.11,12 After the outbreak of COVID-19, most national governments have been encouraged to speed up the development of biosafety, which puts forward higher requirements for researchers in biosafety and relevant fields.13 Therefore, it is necessary to enhance our awareness toward biosafety and implement dynamic real-time detection, identification and tracking of biosafety issues such as environmental disasters and biological threats and carry out surveillance and control measures immediately.
1.2 The research areas of biosafety
The concept of biosafety has already been defined by the scientific community.14–16 It refers to the prevention and control of hazards caused by biological risk factors such as biotechnology and pathogens. However, there are two easily confused concepts: biosafety and biosecurity.17 Biosafety is the prevention of the large-scale loss of biological integrity, focusing both on ecology and human health. It emphasizes the passive prevention and control of unintentionally induced biotechnology and microbial biological hazards.18 In contrast, biosecurity refers to proactive measures to prevent intentional biological hazards and often refers to areas such as national security, biological weapon control, epidemic prevention management, food security, and species invasion.19 Although the two words biosafety and biosecurity have some subtle differences in meaning, without special emphasis, biosafety is generally used. Therefore, herein, the term “biosafety” is used in all subsequent sections.
The research purpose of biosafety is taking effective measures against biological threats. As summarized in Fig. 1, the research areas of biosafety cover a wide range of topics, including controlling infectious diseases, monitoring biotechnology risks, ensuring laboratory biological safety, protecting biological resources, preventing invasion of alien species, defending against biological warfare and biological terrorist attacks.
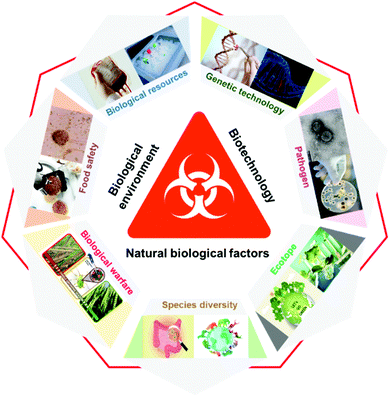 |
| Fig. 1 Research areas of biosafety. | |
2. The proposal of biosafety materials science
2.1 Definition of biosafety materials science
Materials science has shaped the world around us ever since the dawn of civilization. A variety of game changing materials with peculiar properties have been developed to achieve unmet needs.20–24 The Swiss Federal Laboratories for Materials Testing and Research (EMPA) briefly categorizes materials as nanostructured materials, materials for energy technology, materials for natural resources and pollutants, materials for health and performance, and materials for a sustainable built environment (Fig. 2).25 Over the past few decades, we have witnessed a revolution in materials science and how it has pushed forward the development of technology.
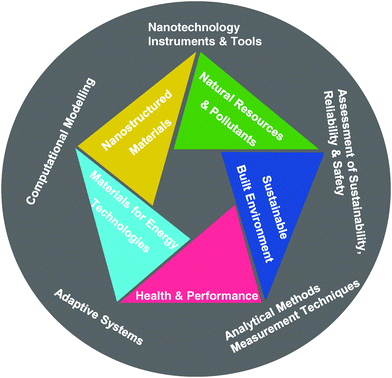 |
| Fig. 2 Classification of materials. | |
At this stage, the entire world is still experiencing a shortage of effective materials in response to biosafety threats. In the COVID-19 pandemic, the lack of biological safety personal protection equipment (PPE) such as masks, protective clothing, goggles, and negative pressure ambulance has resulted in a failure to protect numerous medical professionals.26–30 Moreover, insufficient sensitive and fast virus detection kits greatly impeded patients from being diagnosed, resulting in rapid spread of this pandemic.31,32 Hence, the marriage of biosafety with materials science will greatly help in resolving the existing challenges in biosafety fields, including the detection and disinfection of pathogens, viral vaccines, PPE and biological species preservation (Fig. 3).33–38 Therefore, here, we attempt to give a perspective on how the development of novel materials can help scientists tackle the challenges in biosafety. It is urgent and timely for us to officially propose the brand-new concept of “biosafety materials”, which can be a future scientific discipline that utilizes materials science and theory simultaneously to produce materials, related products, and equipment to solve biosafety problems. To the best of our knowledge, the concept of “biosafety materials” as well as “biosafety material science” has never been officially proposed; therefore, the development of biosafety materials may still lack basic guiding ideology. As a result, researchers in materials science may not realize the problems and understand the challenges in biosafety, while researchers in biosafety may have no idea how materials can be applied to solve the biosafety problems they face. Overall, it is of great significance and timely to put forward the brand-new concept of biosafety materials. By clarifying the concepts and role as well as the importance of biosafety materials, relevant researchers can work together to design advanced biosafety prevention and control materials, which will eventually improve our ability to tackle biosafety-related problems.
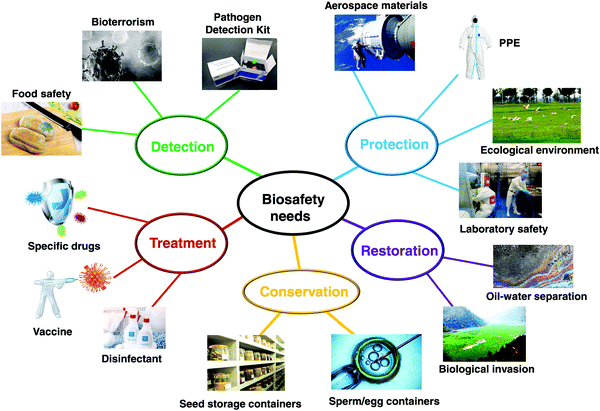 |
| Fig. 3 Biosafety needs and their corresponding biosafety materials. | |
2.2 Current challenges in the field of biosafety
To address biosafety-related issues with materials, the current challenges in this field need to be specified.39–41 For example, the current mainstream pathogen detection method still largely relies on polymerase chain reaction (PCR), which is time-consuming and laborious. Point-of-care testing (POCT) devices are highly desired due to their convenience.42–45 Luminescent materials provided a powerful tool to achieve sensitive and timely detection.46–48Table 1 briefly summarizes the major challenges in biosafety from the perspective of materials science.
Table 1 Current challenges in biosafety
Biosafety field |
Category |
Challenges |
Pathogen detection |
Cultivation and identification |
Detection process is time consuming |
Pathology instrumentation |
Low sensitivity, specificity |
Immunological detection |
False negative results |
Molecular biological detection |
|
|
Pathogen disinfection |
Physical disinfection (ultraviolet rays, high temperature, ionizing radiation, etc.) |
Low efficiency |
Chemical disinfection (alcohols, chlorine-containing disinfectants, phenols, quaternary ammonium salts, and iodine-containing disinfectants) |
Scented |
|
Hazardous chemical residues |
|
Drug resistance |
|
Corrosive |
|
Personnel must leave the field during disinfection |
|
Treatment drugs |
Anti-bacterial drugs |
Drug resistance |
Anti-viral drugs |
The virus has no cell structure, few targets, and drug development is difficult |
|
Vaccines |
Inactivated vaccine |
Slow R & D |
Live attenuated vaccine |
Invalid after virus mutation |
Subunit vaccine |
Safety issues |
Genetic engineering vaccine |
Lack of good adjuvant |
|
Protective equipment |
Protective suit |
Poor anti-toxicity, breathability, and heat dissipation |
Mask |
Low protection against aerosols |
Gloves |
Unable to be reused |
|
Protection of biological resources |
Protect plant resources |
Extraction is easily contaminated |
Protect blood resources |
Plant seeds are vulnerable to environmental restrictions |
Protection of human genetic resources |
|
|
Detection of biological invasion |
Natural intrusion protection |
Difficult to detect at the initial introduction stage |
Unintentional introduction of protection |
Gene drift is prone to occur after invasion |
Intentional introduction of protection |
|
|
Protection of ecosystem |
Control of environmental pollution |
Low oil–water classification efficiency |
Improving climate warming |
Difficulty in detecting radiation species |
Protecting biodiversity |
Low harmful gas adsorption rate |
|
Low removal rate of heavy metal pollution |
|
Biochemical weapon |
Biological weapon protection |
Investigation difficulties |
Biological warfare agent protection |
Large-scale popular |
Chemical weapon protection |
Easily misdiagnosed |
Bioterrorism protection |
Ineffective vaccine |
|
Genetic technology |
Optimizing GMOs |
Gene drift |
Suppression of gene mutations |
Negative changes in resistance |
Reduce or increase resistance |
Genetic mutation |
Pharmaceutical biotechnology |
Genes affecting non-target organisms |
|
Food biosafety |
Detection of carcinogens |
Food shelf-life is too short |
Screening for contaminants |
Easy to be contaminated by microorganisms |
Food preservation |
Hard to detect carcinogens |
Pesticide drug testing |
|
|
Aerospace biosafety |
Control of body environment |
Cosmic radiation |
Personal protection |
Physical and chemical corrosion |
Aerospace lifesaving |
Mechanical wear |
2.3 “Biomaterials” and “biomedical materials”
The term “biosafety materials” is also different from “biomaterials” and “biomedical materials”. A biomaterial is any substance that has been engineered to interact with biological systems for a medical purpose, either a therapeutic (treat, augment, repair or replace a tissue function of the body) or diagnostic one.49–52 As a science, biomaterials is about fifty years old. The study of biomaterials is called biomaterials science or biomaterials engineering. It has experienced steady and strong growth over its history, with many companies investing large amounts of money into the development of new products. Biomaterials science encompasses elements of medicine, biology, chemistry, tissue engineering and materials science.53–55 Biomedical materials are biomaterials that are manufactured or processed to be suitable for use as medical devices (or components thereof), which are usually intended to be in long-term contact with biological materials.54,56 However, biosafety materials emphasize the use of materials for the prevention and control of biological safety issues. Taken together, biosafety materials are different from both “biomaterials” and “biomedical materials”.
2.4 “Biosafety materials” and “biosafety of materials”
The biological safety of materials is mentioned frequently when people study biomaterials.57–59 Herein, considering that the concepts of “biosafety materials” and “biosafety of materials” are confusing, a comprehensive comparison between them is provided here. “Biosafety materials” in essence can be “materials for biosafety”, which denote the application of materials and related theory to tackle biological safety issues. However, the latter “biosafety of materials” denotes whether materials are safe to biological systems, how toxicity occurs and to which extent there is toxicity. In general, the “biosafety” in the term “biosafety materials” refers to biological safety, namely, the biological parameters, such as virus and bacteria may cause safety issues to the environment and human body; however, the “biosafety” in the term “biosafety of materials” refers to the safety of certain materials to bio-organisms. The two biosafety terms are the same in written word, but they are terms used in different research fields from different backgrounds, and thus their meanings and connotations vary greatly.
Taking nanomaterials as an example, when the concept of nanomaterials was first proposed, most scientists were attracted by the fascinating properties nanomaterials brought with them, but few realized and considered their safety issues. In 2001, Prof. Yuliang Zhao from The National Center for Nanoscience and Technology in China firstly proposed the concept “biosafety of nanomaterials”, which is also termed nanotoxicity (nano-safety).60 Since then, the importance of the negative side (such as toxicity) of nanomaterials has been realized by scientists around the world. They further established the CAS (Chinese Academy of Sciences) Key Laboratory for Biological Effects of Nanomaterials and Nanosafety in China. However, biosafety nanomaterials refer to the use of nanotechnology and nanomaterials to address biosafety issues, which is significantly different from the concept of nanotoxicity and biosafety of nanomaterials. To better understand this, these two terms, “biosafety of materials” and “biosafety materials”, are compared in detail in Table 2.
Table 2 Biosafety materials and biosafety of materials
Item |
Biosafety materials |
Biosafety of materials |
Definition |
Develop materials for prevention and control biological safety issues. |
Evaluate the side effects and toxicity of materials. |
|
Research content |
Design and develop new materials for biosafety issues; evaluate their abilities for prevention and control of the biological threat. |
Study the toxicity and toxicology of materials to biological species. |
|
Research aim |
Protect human health and other species. |
Determine whether there is any toxicity of materials. |
Conserve the biodiversity. |
Understand to what extent the toxicity of materials is and how the toxicity occurs. |
Protect the ecological environment. |
Determine the possible acceptable dosage of materials |
Prevention and control other biological issues. |
|
|
Research direction |
Find the most efficient, cheapest and best materials to solve biosafety issues. |
Reduce the toxicity of materials to the human body. |
3. State-of-the-art of biosafety materials
Although the concept of “biosafety material science” has not been officially proposed to date, we can still find a number of studies related to the biosafety materials, including pathogen detection,61–63 virus detection,64–68 prevention and control of infectious diseases,69–71 PPE,72–75 preservation of biological and human resources,76–79 biological invasion monitoring,80–82 biological weapons and biological terrorist defense.83–87 Herein, the state-of-the-art biosafety materials are discussed in depth, which provides a comprehensive understanding for researchers in the related field and can help propel this concept in the newly emerging field.
3.1 Biosafety materials for viral vaccines
The long battle history between viruses and humans has proven that vaccination is the best solution to completely contain the spread of viruses.88–90 It effectively prevents disease through boosting the immune system against a pathogen. Since there are safety concerns on viral delivery systems, several biosafety materials have been extensively investigated for the in vivo delivery and controlled release of viral vaccines, including liposomes, polymers, cationic proteins and biological membranes.91,92 Viral vaccines can be delivered in the form of DNA, mRNA or protein,93 all of which can be easily enzymatically degraded upon entering the blood circulation.94,95 Taking SARS-CoV-2 for example, it contains 1273 amino acids with a molecular weight of about 140 kDa. The DNA encoding SARS-CoV-2 is more than 3800 bp.96 Thus, to protect the vaccine in circulation and help it be endocytosed into the cells, delivery vehicles are required. Moderna utilizes ionizable liposomes to carry negatively charged mRNA for the SARS-CoV-2 spike protein.97,98 A phase I clinical trial is underway in Seattle, (NCT04283461, USA). Moreover, research has shown that polymers such as low-molecular-weight polyethyleneimine (PEI) modified with fatty chains and poly(β-amino) esters (PABEs) can be designed to deliver DNA and mRNA.92,99 In addition, protamine, as a natural cationic protein, can form complexes with negatively charged nucleic acids, and thereby be utilized to deliver mRNA-based therapeutics and stimulate an immune response.92 Furthermore, biological membranes such as red blood cell membranes and extracellular vesicles including exosomes, apoptotic bodies and microvesicles can be isolated and utilized for the delivery of biomolecule-based vaccines.100,101 Recently, the microneedle patch, as a novel drug delivery system, has attracted extensive scientific interest due to its excellent properties such as painless penetration and excellent therapeutic efficacy. It provides a highly efficient transdermal delivery system to create sophisticated devices with superior features for biomedical applications. Gambotto's group in the University of Pittsburgh developed the SARS-CoV-2 vaccine based on microneedle arrays (Fig. 4).102 Compared to the traditional subcutaneous needle injection, MNA SARS-CoV-2 subunit vaccines elicited strong and long-lasting antigen-specific antibody responses. In summary, biosafety materials are of great importance in the development of biomolecule-based therapeutics and are already used to combat viral infections.
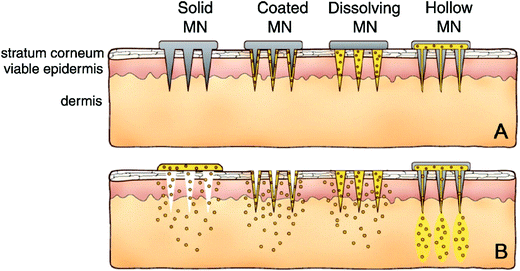 |
| Fig. 4 Biosafety materials based on the microneedle patch for SARS-CoV-2 vaccine delivery. (A) Solid MNs pierce through the outer layers of the skin, leaving open space. (B) Vaccine diffuses into the skin through the open pores. Reproduced with permission from ref. 102. Copyright 2012, Elsevier. | |
3.2 Biosafety materials for pathogen detection
3.2.1 Biosafety materials for viral detection.
The development of vaccines and drugs usually takes as long as several years, which means there is possibly no effective way to protect against infectious pathogenic microorganisms in the early stage of infectious disease outbreaks.103 Hence, the early detection of viruses is the key to combating the spread of infectious diseases. This is because the information from tests can help governments make public health decisions about measures for the control and prevention of an outbreak. Reverse transcriptase polymerase chain reaction (RT-PCR) has been widely used as a gold standard for virus detection. Although it can provide a reliable result, the process for detection is laborious and time-consuming. Normally, it can take several days or up to even one week to obtain results on an exceptional circumstance, which can cause the best timing to control the disease outbreak to be missed. Thus, rapid virus detection systems such as POCT, with high sensitivity and selectivity are highly desired in the clinic. Accordingly, emerging materials with peculiar properties provide new options to meet this unmet demand.
Microfluidics is a revolutionary technology that manipulates small amounts of fluidics (10−9–10−18 L), thereby becoming powerful for viral detection (Fig. 5B). Yeh et al. reported a portable and high-throughput microfluidic VIRRION platform containing carbon nanotube arrays (Fig. 5A).104 VIRRION not only effectively captures different viruses by size, but also performs real-time nondestructive identification of virus using surface-enhanced Raman spectroscopy (SERS) coupled to a machine learning and database. The research team validated this device using different subtypes of avian influenza A viruses and human samples with respiratory infections, reporting the successful enrichment of rhinovirus, influenza virus and parainfluenza viruses. This device is also reported to maintain stoichiometric viral proportions when samples contain more than one type of virus, suggesting it can also be functional in cases where coinfection has occurred. The processing time (including viral enrichment as well as detection) takes only a few minutes with a 70-fold enrichment enhancement. The sensitivity of this system can reach as little as 102 EID50 per mL, with a virus specificity of 90%, indicating its potential to realize POCT. Furthermore, Sun et al. designed a point-of-care micro-fluid system integrated with a smartphone for live virus detection. The detection limit is comparable to the traditional RT-PCR, with the result achieved within 30 minutes.105 Hence, microfluid technology endows detection systems with appealing versatility and reliability, exhibiting distinct advantages over traditional RT-PCR.
The outbreak of the Ebola virus (EBOV) in West Africa underscored the need to develop highly sensitive tests for diagnosis as early as possible. Chou et al. developed a 3D plasma nanoantenna measurement sensor as an on-chip immunoassay platform for the ultra-sensitive detection of the EBOV antigen (Fig. 5B).106 Compared with the flat gold substrate, the EBOV sensor exhibited a significant increase in fluorescence intensity. The nano-antenna-based biosensor could detect EBOV soluble glycoprotein at a concentration as low as 220 fg mL−1. The sensitivity of this biosensor is 240
000 times higher than the existing FDA (Food and Drug Administration)-recommended immunoassay-based tests. This sensor can be further adapted to a universal biosensing platform for other viruses. Zheng et al. utilized carbon nanotubes to develop a portable device that can selectively capture viruses based on their size.107 This carbon nanotube assay can selectively capture and aggregate viruses in diluted samples based on the size of the virus, thus increasing the detection threshold of the virus by a factor of 100. During the whole isolation process, no antibody is required for detection, which simplifies the operation for viral detection.
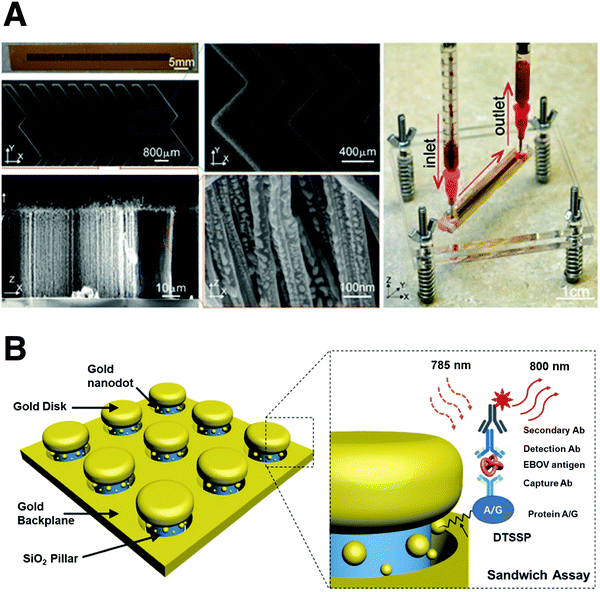 |
| Fig. 5 Biosafety materials for virus capture and detection. (A) High-throughput microfluidic VIRRION platform containing carbon nanotube arrays for influenza virus capture and identification. Reproduced with permission from ref. 104. Copyright 2020, National Academy of Sciences. (B) Schematic illustration of 3D plasmonic nanoantenna array for EBOV sensor. Reproduced with permission from ref. 106. Copyright 2019, Wiley-VCH. | |
Nanoenzymes, as functional nanomaterials with enzyme-like characteristics, have gained tremendous attention in viral detection.108,109 For example, nanoenzymes demonstrated remarkable sensitivity and specificity in detecting the avian influenza A (H5N1) virus. Ahmed et al. utilized Au NP as peroxidase to amplify the signal for avian influenza A virus detection (Fig. 6A).110 This dual enhanced colorimetric immunosensor enabled the detection of H5N1 with a limit of detection (LOD) as low as 1.11 pg mL−1, suggesting that it was more sensitive than ELISA or bioassays based on plasmonics. It has been further applied to detect other avian influenza A viruses such as H4N6 and H9N2. In addition, Yan's group developed an Fe3O4 magnetic nanoparticle (MNP)-based nanoenzyme-strip for the detection of EBOV, which is 100-fold more sensitive than the standard strip method, thereby providing a valuable simple screening tool for the diagnosis of various pathogens (Fig. 6B).111
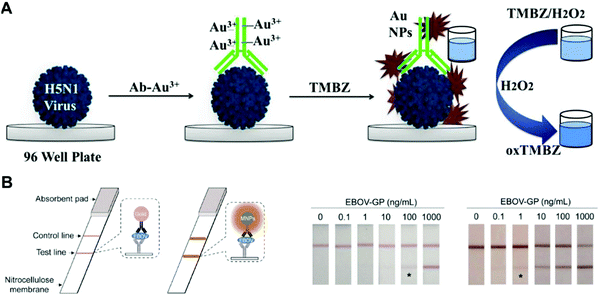 |
| Fig. 6 Nanoenzyme-based biosafety materials applied in virus detection. (A) Mechanism of immunosensor with Au NPs for the detection of the avian influenza virus. Reproduced with permission from ref. 110. Copyright 2017, Ivyspring International Publisher. (B) Nanozyme strip based on MNP for the detection of EBOV. Reproduced with permission from ref. 111. Copyright 2015. Elsevier. | |
3.2.2 Biosafety materials for bacteria detection.
Most bacteria are harmless and even beneficial, but some bacteria are pathogens that can cause many infectious diseases such as tuberculosis, which result in about two million deaths each year.112,113 Moreover, the worldwide trend of the increasing prevalence of antimicrobial resistance resulting from the outright abuse of antibiotics has become a major public health problem. Thus, the development of POCT can significantly help identify an effective antibiotic for the infection of a patient. Xiao et al. developed a novel biosensor for the high-throughput detection of bacteria based on the DNA molecular machine.114 The platform successfully realized single-step, fast and multi-channel high-throughput bacterial detection, as shown in Fig. 7A. The SDwalker drop biosensor platform adopts the trigger spontaneous motion mechanism of a walking molecular machine, which has the characteristics of rapid analysis and single bacterial analysis. The signal amplification strategy based on SDwalker drop operation can be used for the highly sensitive detection of bacteria (1 CFU mL−1), exhibiting a promising platform for the detection of bacteria.
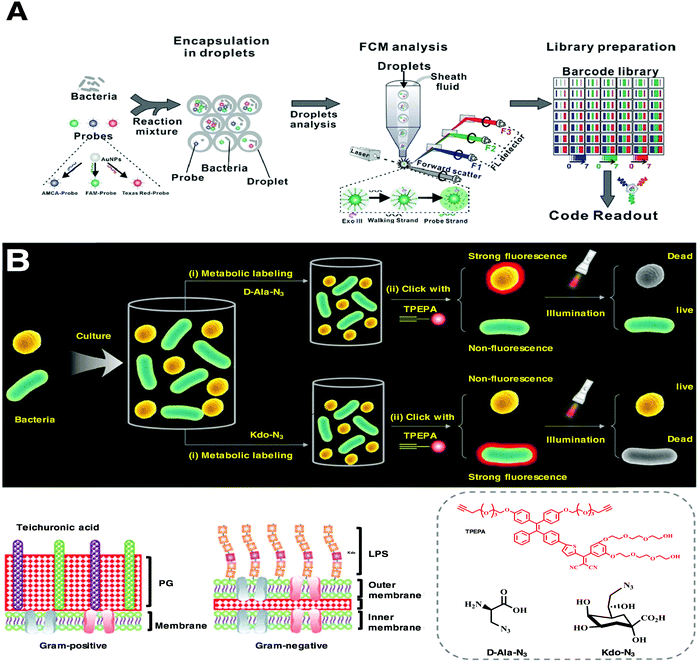 |
| Fig. 7 Biosafety materials for the detection of bacteria. (A) Schematic of the SDwalker-Drop platform for super-multiplex bacterial phenotype analysis. Reproduced with permission from ref. 114. Copyright 2019, Wiley-VCH. (B) Schematic illustration of the use of an AIE material for the discrimination and precise ablation of bacterial pathogens. Reproduced with permission from ref. 122. Copyright 2019, American Chemical Society. | |
Traditional fluorescent probes have the effect of aggregation-induced quenching (ACQ), but the complex physiological environment can reduce the selectivity and sensitivity of fluorescent probes.115,116 In contrast, molecules with the aggregation-induced emission (AIE) effect can overcome the shortcomings of traditional fluorescent molecules, and no complicated washing steps are required due to their property of low fluorescent background.117,118 These advantages of AIE molecules endow sensitivity and reliability to detection systems.119–121 Liu et al. designed a bioorthogonal fluorescence turn-on probe, TPEPA, for the discrimination and precise ablation of bacterial pathogens.122 TPEPA (tetraphenylene polyethylene glycol AIEgen) is a type of AIE photosensitizer with good water solubility, and its alkynyl group can be linked to an azide group via click reaction (Fig. 7B). Taking advantage of the difference in bacterial structures, TPEPA can discriminate pathogens via selective imaging of metabolically decorated Gram-negative bacteria with Kdo-N3 and Gram-positive bacteria with D-Ala-N3, respectively, thus achieving the selective detection and killing of bacteria in situ. The use of the fluorescent properties of organic materials solves the problems of difficulty in accurately identifying pathogens in a short time.123–126
3.3 Biosafety materials for disinfection
The wide use of disinfection is beneficial in preventing infectious diseases, and thus is beneficial for the public health. It relies on physical or chemical methods to eliminate pathogens that stay on different transmission vehicles, thereby cutting off the transmission route to prevent and control the spreading of infections. Various chemical compounds such as alcohol, iodine-containing disinfectants, chlorine-containing disinfectants, peroxides, phenols, and quaternary ammonium salts have been widely used as disinfectant. However, these compounds suffer from multiple drawbacks such as harmfulness and corrosive nature.
Nanomaterials exhibit antimicrobial effects owing to their high surface-area-to-volume ratio and unique physical and chemical properties. Particularly, tremendous attention has been paid to Ag NPs due to their practical applications in our daily life. They have been widely used in different sectors such as silver-based air/water filters, textiles, animal husbandry, biomedical industry and food packaging.127 Huang et al. designed a novel polymeric micelle for simultaneously decorating Ag NPs and encapsulating curcumin as a combination strategy to improve the antibacterial efficiency (Fig. 8).128 Through rational design, the aggregation of Ag NPs could be avoided, and the solubility of curcumin was improved simultaneously. Excellent antibacterial activity toward the Gram negative P. aeruginosa and Gram positive S. aureus has been well demonstrated, thereby proving its potential in disinfection.
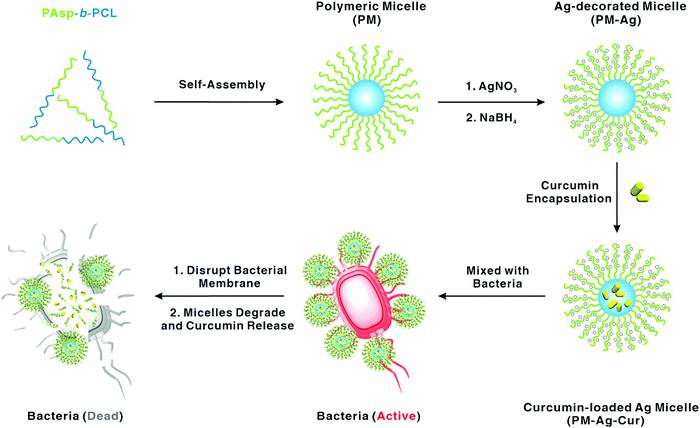 |
| Fig. 8 Biosafety materials based on Ag NPs for disinfection. Schematic illustration of the formation of silver-decorated polymeric micelles encapsulating curcumin simultaneously for enhanced antibacterial activity. Reproduced with permission from ref. 128. Copyright 2017, American Chemical Society. | |
3.4 Biosafety materials for drug treatment
The discovery of anti-microbial agents has revolutionized our strategy to treat bacterial diseases in the twentieth century.129,130 However, the misuse and outright abuse of antibiotics have resulted in multidrug-resistant (MDR) pathogenic bacteria. Nanotechnology-based antibacterial strategies significantly enrich the tools to fight against MDR bacteria. As shown in Fig. 9A, Liang's group designed near infrared (NIR)-activated TRIDENT (Thermo-Responsive-Inspired Drug-Delivery Nano-Transporter) for effectively eradicating clinical methicillin-resistant Staphylococcus aureus.131 Imipenem (IMP, a broad-spectrum antibiotic) and IR 780 (a photosensitizer molecule) were encapsulated to fabricate smart triple-functional thermo-responsive nanoparticles. The temperature increase generated by NIR not only melted the nanotransporter via a phase change mechanism, but also damaged the bacterial membranes to facilitate imipenem permeation, thereby achieving robust bactericidal capabilities via chemo-photothermal therapy. The unique properties of carbon-based materials significantly aid in dealing with antimicrobial resistance. As shown in Fig. 9B, Zheng et al. designed an La@GO nanocomposite library for killing antibiotic-resistant bacteria.132 Unlike conventional antibiotics or Ag, long-term exposure to La@GO at sub-MIC for 30 days did not induce detectable secondary resistance in E. coli. A novel extracellular multitarget invasion (EMTI) mechanism was also proposed to explain the result, which can help scientists develop more specific systems based on this system. The first antibiotic penicillin was discovered in 1928. Subsequently, numerous lives have been saved, and a variety of antibiotics have contributed to the control of infectious diseases. However, currently, there are very few antibiotics under development, and the number of effective antibiotics is declining. Thus, novel materials with versatile properties provide new strategies to treat MDR bacteria and viruses, providing a different route to overcome drug resistance.
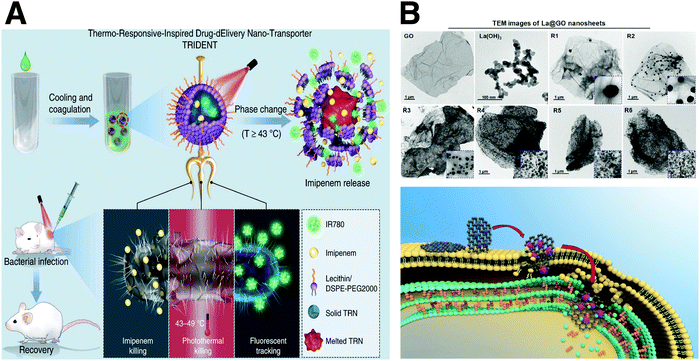 |
| Fig. 9 Biosafety materials for combatting MDR bacteria. (A) Schematic illustration of near infrared (NIR)-activated TRIDENT for killing antibiotic-resistant bacteria. Reproduced with permission from ref. 131. Copyright 2019, Nature. (B) Graphene oxide nanocomposite for preventing the evolution of antimicrobial resistance. Reproduced with permission from ref. 132. Copyright 2019, American Chemical Society. | |
3.5 Biosafety materials for PPE
Serving as the first line of defense in the fight against viruses, high-performance PPE is crucial for protecting frontline medical professionals and the general public.133,134 Current PPE suffers from poor light resistance, and no antimicrobial effect is provided. Furthermore, it is difficult for PPE materials to be effectively disinfected under the premise of good preservation.135,136 Hence, stable and protective materials with broad antimicrobial activity are in high demand.
For stopping the spread of highly infectious diseases, air filtration has become an efficient passive pollution control strategy. Nevertheless, most of the commercial air purifiers solely rely on dense fibrous filters, which can effectively remove particulate matter but lack antibacterial activity. Li et al. designed a series of metal–organic frameworks (MOFs) with photocatalytic bactericidal properties to fabricate a nano-fiber membrane.137 It can effectively produce biocidal reactive oxygen species (ROS), which is driven by sunlight (Fig. 10A). Specifically, a zinc-imidazolate MOF (ZIF-8) exhibits almost complete inactivation of Escherichia coli (E. coli) (>99.9999% inactivation efficiency) in saline within 2 h of simulated solar irradiation. This MOFilter provides new insights into the sustainable, self-charging, and adaptive development of protective materials, which represents the next generation of PPE. Moreover, a polymer material was developed to make face mask protection equipment (Fig. 10B). Liu et al. designed a novel self-powered electrostatic adsorption face mask (SEA-FM) based on a poly(vinylidene fluoride) electrospun nanofiber film (PVDF-ESNF) and a triboelectric nanogenerator (TENG).138 Up to 99.2% particulate removal efficiency could be achieved, which is much higher than that of the commercial mask. After the outbreak of COVID-19, the demand for masks has exploded worldwide. However, the disposal of the massive number of single-use masks poses a significant environmental threat to society. Thus, to ease this problem, reusable and recyclable graphene masks with outstanding superhydrophobic and photothermal performances were developed by Li et al. The high surface temperature of the masks under solar illumination can effectively sterilize the surface viruses. It is believed that more advance materials can be properly applied by scientists to produce multi-functional masks.135
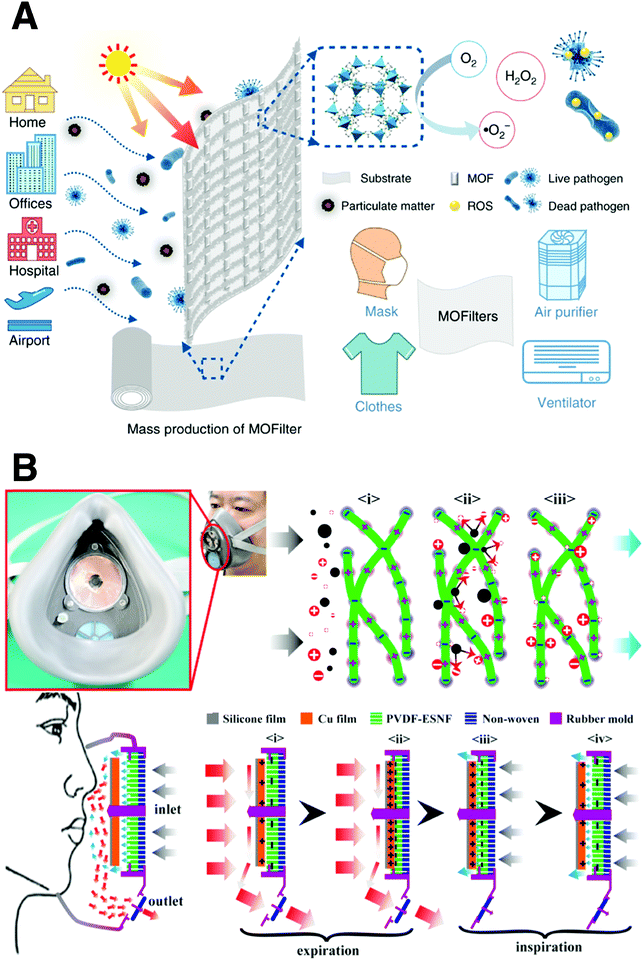 |
| Fig. 10 Biosafety materials for PPE with antimicrobial capacity. (A) Schematic illustration of metal–organic framework (MOF)-based filter. Reproduced with permission from ref. 137. Copyright 2019, Nature. (B) Schematic illustration of the filtration mechanism of the polymeric material mask. Reproduced with permission from ref. 138. Copyright 2018, American Chemical Society. | |
Surgical suits are special clothing required by doctors to perform surgical operations. The materials used need to possess protective properties to block viruses and bacteria from invading medical personnel, which should not only be sterile, dust-free and disinfection resistance, but also isolate bacteria, antibacterial and comfortable. Accordingly, a three-layer antiviral surgical suit with non-woven spunbond polypropylene, polyester and microporous PTFE film was developed.139 Plasma technology was used to treat the outer layer of the spunbond polypropylene. The results showed that the plasma-treated surgical gown had a 99.04% reduction in microbes compared to the non-plasma-treated surgical gown, providing a microbial barrier for medical staff.140–143
Respirators are a type of sanitary product, generally referring to that worn in the nose and mouth to filter the air entering the nose and mouth to block harmful gases, odors, droplets, viruses and other substances. Masks have a certain filtering effect on the air entering the lungs. When respiratory infectious diseases are prevalent and when working in a polluted environment such as a dusty area, wearing a mask is necessary to keep workers safe.144,145 Novel material fabrication technology such as 3D printing has been applied to manufacture masks.146 The N95 filtering facepiece respirator was custom designed for medical staff. Comfort and fit are essential while wearing an FFR, especially for medical professionals who need to work for a long period to treat patients. The face seal prototypes of masks were prepared with acrylonitrile butadiene styrene (ABS) plastic using 3D printing, which provide improved contact pressure for users.
3.6 Biosafety materials for protection and preservation of biological resources
With economic globalization, environmental pollution and global warming, biodiversity has faced unprecedented challenges. The preservation of biological resources provides a guarantee for the biodiversity of the ecological system. Generally, biological genetic resources mainly include the extraction and protection of animal and plant genetic materials such as biological genes, sperm and eggs.147–150 The major shortcomings for the current protection methods are the low survival rate of seeds and possibility of uncontrollable mutation. Cheng et al. discovered that PVP (polyvinylpyrrolidone) and PVPP (polyvinylpolypyrrolidone) could effectively remove multiple phenolic compounds and terpenoids during the process of extracting DNA.151 Therefore, the addition of an appropriate amount of PVP or PVPP to the DNA extraction solution can improve DNA purity, remove polysaccharides, and reduce polyphenol contamination. This is a good example that biosafety materials display protective significance on genetic resources. The PVP method has now been accepted as one of the most used DNA extraction techniques, thereby indicating that the development of materials actually changes the technic in protecting biological resouces.152–155
The protection of plant seeds is also a crucial biosafety issue.156,157 Seed enhancement technologies play a pivotal role in supporting food security by enabling the germination of seeds in degraded environments. Marelli et al. combined silkworm cocoon S molecules and trehalose to design a new seed coating method.158 As shown in Fig. 11A, this formulation is capable of precisely coating seeds with biofertilizers and releasing them in the soil to boost seed germination and mitigate soil salinity. The coated seeds yielded plants that grew faster and stronger in the presence of saline soil. Hence, this study opens the door to the application of advanced biosafety materials in precision agriculture, introducing the drug delivery concept to seed protection. Furthermore, polymeric materials have been proven to reduce environmental side effects and improve seed survival, suggesting the importance of composite materials in the protection of biological resources.159–162
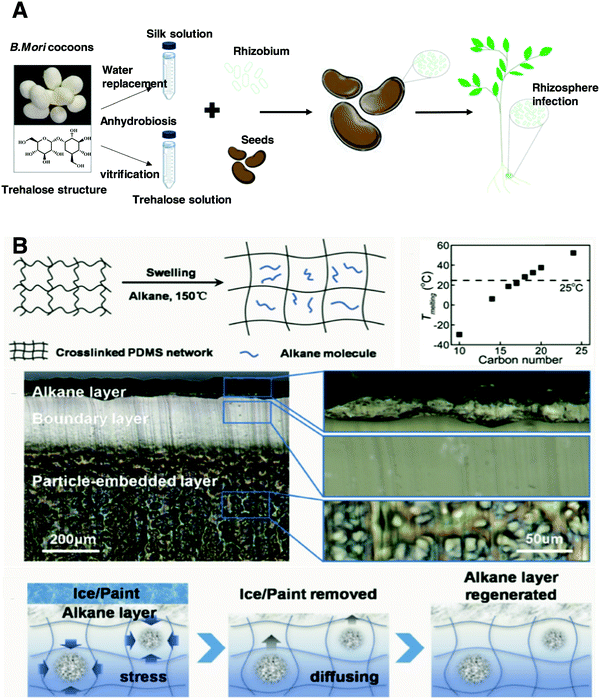 |
| Fig. 11 Biosafety materials for the protection and preservation of biological resources. (A) Biosafety materials based on silk fibroin and trehalose for seed coating, which can boost germination and mitigate abiotic stress. Reproduced with permission from ref. 158. Copyright 2019, National Academy of Sciences. (B) Principle of the solid organogel material to cryopreserve biological resources. Reproduced with permission from ref. 164. Copyright 2017, Wiley-VCH. | |
Cryopreservation is the classical way to preserve human genetic resources.163 However, the mechanical damage effect of ice crystals is the major problem in cryopreservation. Inspired by the regenerable solid epicuticular wax on the surface of land plant leaves, Wang et al. developed solid organogel materials with a regenerable sacrificial alkane surface (Fig. 11B).164 This type of surface material was demonstrated to be of great practical importance for tackling solid deposition, such as anti-icing, anti-graffiti, and antifouling. Compared with the ice adhesion strength of aluminum (372.2 + 47.4 kPa) and PDMS (146.3 + 9.5 kPa), the ice adhesion strength of the solid organic gel was reduced to 68.8 + 10.4 kPa. The adhesion value remained almost unchanged when the temperature decreased from −20 °C to −70 °C, indicating its potential for the cryopreservation of natural biological resources.
3.7 Biosafety materials for the prevention of biological invasion
Biological species invasion has been considered as one of the most critical ecological disturbances that threatens the native biodiversity.165–167 In 1996, the Global Invasive Species Program was launched. Since then, biological invasion has been a focus and heavily studied.168,169 Biological invasions can be divided into several stages, including introduction, escape, population establishment and harm. Except for purposeful introduction, the early detection of other invasion ways is challenging. If the invasion reaches a detectable range, it is hard to be removed. Hence, early detection is crucial to prevent biological invasion. The development of genetic detection technology has facilitated the detection of biological invasions. Wang et al.170 developed a sensitive nucleic-acid sensing platform based on superhydrophilic microwells spotted on a superhydrophobic substrate (Fig. 12). The difference in wettability facilitated the conversion of trace analyte between the micropores and surrounding substrate into superhydrophilic microporous materials. Due to the condensation enrichment effect, ultratrace DNA detection was realized, and the detection limit reached 2.3 × 10−16 M. The genome of certain species can be rapidly determined using a gene library, enabling the fast identification of invading species.
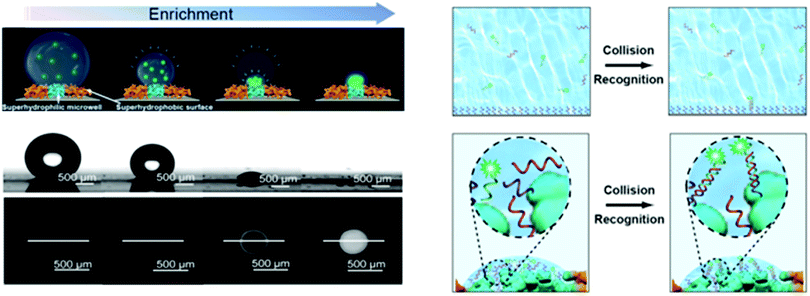 |
| Fig. 12 Biosafety materials for the early detection of biological invasion. Ultratrace DNA detection based on superhydrophilic microwells spotted on a superhydrophobic substrate. Reproduced with permission from ref. 170. Copyright 2015, Wiley-VCH. | |
Since invasive alien species have no natural enemy, they can rapidly settle in a new environment and quickly grow into an overlord community, eventually destroying the local ecosystem and inhibiting the growth of other local species. The loss of biodiversity in ecosystems further leads to irreversible consequences, such as the disappearance of forests, pastures degradation, and water pollution. Hence, in addition to the early detection of biological invasion, it is of great significance to effectively eliminate invaded alien species. Fortunately, the biosynthesis of metal nanoparticles provides an environmentally friendly approach to fight against invading alien species. Oscillatoria, as one of the most common cyanobacterial genera known to produce neurotoxins, has negative impacts on aquatic organisms. To stop the growth of Oscillatoria, the Ag-NPs biosynthesized by specific alga exert outstanding negative impacts on it, which significantly alleviates the environment burden through green synthesis.171 A similar biosynthesis strategy has also been found for controlling vector mosquitoes. The plant-synthesized AgNPs developed by Rajakumar et al. can effectively eliminate mosquitoes, providing an effective way to control mosquito-borne diseases.172 Hence, the development of materials science provides bio-friendly alternatives for the prevention of biological invasion, enriching the methods to deal with difficult environmental-related issues.
3.8 Biosafety materials for protection of ecological environment
The ecological environment is a prerequisite for ensuring species diversity. The 2010 Deepwater Horizon oil spill in the Gulf of Mexico has been regarded as the worst environmental disaster in the United States, which released about 4.9 million barrels of crude oil, and had a huge impact on the ecological system. Thus, efficient and cost-effective separation strategies for spilled oil are greatly needed. Janus particles are colloidal particles with more than one type of surface chemistry or composition, which provide a suitable system for oil separation. Song et al. developed magnetic Janus particles with a convex hydrophilic surface/concave oleophilic surface, realizing the rapid and efficient separation of microscale tiny oil droplets from water (Fig. 13).173 Ren et al.174 developed a phase-selective organic gelling agent, which not only showed the ability to selectively condense oil from oily water, but also could easily separate colloidal oil and water from the human body. This powder gelator exhibited remarkable ability to achieve rapid gelation of crude oils of widely ranging viscosities within minutes at room temperature.
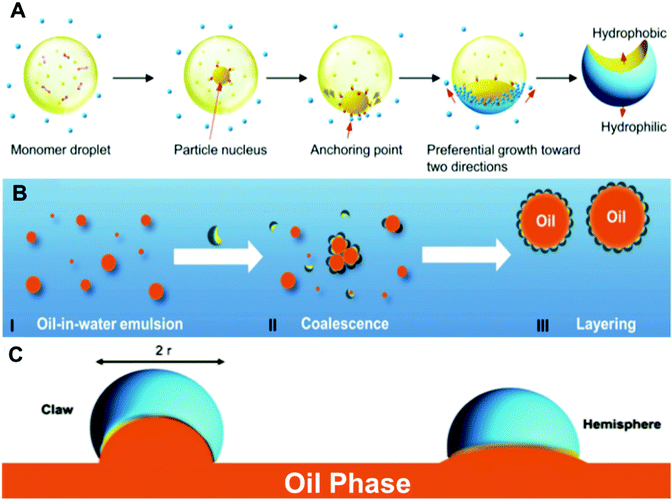 |
| Fig. 13 Biosafety materials for separating spilled oil from water. (A) Schematic illustration of the principle of hydrophilic/oleophilic magnetic Janus particles. (B) Schematic of the separation of tiny oil droplets by Janus particles. (C) Schematic of two different types of Janus particles. Reproduced with permission from ref. 173. Copyright 2018, Wiley-VCH. | |
Heavy metals are harmful and toxic pollutants that are difficult to degrade. They not only cause a degradation in soil quality, but also enter the human body through direct contact or the food chain. In recent years, scientists have gradually realized that heavy metals have a massive impact on the microbes in soil, which eventually influence the soil microbial activity, microbial community, and soil enzyme activity. Thus, to ameliorate this condition, scientists have developed novel materials to remove heavy metal pollution in the soil ecosystem.175 Lian et al. developed a simple and economical path to prepare mercapto-functionalized nanosilica.176 Nanosilica, which has excellent compatibility with soils, was chosen as the matrix. This biosafety material efficiently remediated Pb/Cd-contaminated soils, exhibiting a high immobilization efficiency of 99.12% and 98.23% towards Pb and Cd, respectively.176 In addition to silica, carbon-based materials have also been selected to deal with this problem due to their excellent absorption capacity, providing versatile methods to protect the ecological environment.177,178
3.9 Biosafety materials for protection against bioterrorism
Bioterrorist attacks have been regarded as the most important biosecurity threat in modern society. Monitoring and medical and health response are the two critical measures for preventing bioterrorism. The biological warfare agents that terrorists can use for attack include Bacillus anthracis, Brucella, Rickettsia przewalskii, and Yersinia pestis. These agents are mainly spread through aerosols, food and water sources. Bioterrorism possesses the characteristics of strong infectivity, strong concealment, simple production process, and wide impact. Anthrax spores are an ideal biological weapon since they are highly lethal to human beings and animals.179–181 Thus, rapid detection methods for anthrax spores are in great demand. As shown in Fig. 14, Tang et al. designed a rare earth-functionalized micelle nanoprobe for the ratiometric fluorescence detection of an anthrax spore biomarker, pyridine dicarboxylic acid (DPA).182 The detection strategy was ascribed to the Tb3+ ions in the lanthanide-functionalized micelle, which can be sensitized to emit intrinsic luminescence upon the addition of DPA due to the occurrence of energy transfer when the DPA chromophore coordinates with the Tb3+ ion. This nanoprobe can detect DPA within a linear range of 0–7 μM within a few seconds, and the detection limit is up to 54 nM. It is believed that the defense against biological weapons can be strengthened by incorporating novel materials with peculiar properties into the detection system.
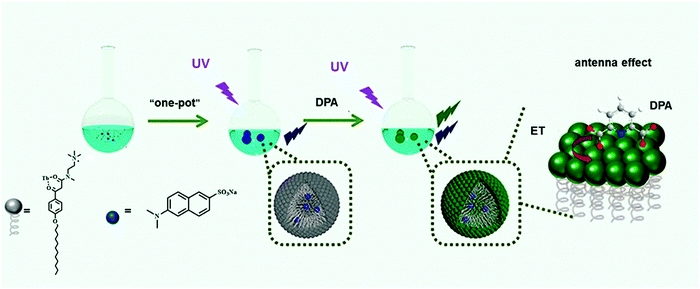 |
| Fig. 14 Biosafety materials based on a ratiometric fluorescence lanthanide-functionalized micelle for the detection of an anthrax spore biomarker. Schematic illustration of the “one-pot” self-assembly of terbium-functionalized micelle in H2O and its response property for DPA. Reproduced with permission from ref. 182. Copyright 2018, American Chemical Society. | |
Because of the acute neurotoxicity caused by biological weapons, saving victims after exposure remains challenging. Neuro-drugs are organophosphorus compounds (OPS) that block the communication between the nerves and organs, which have been used as biological weapons.183–185 Jiang et al. developed a nanoscavenger, which exhibited a long-term protective effect on OPS poisoning in rodents (Fig. 15).186 It could catalyze the decomposition of toxic OPS, and exhibited excellent pharmacokinetic characteristics and negligible immune response in OP-poisoned rats. In a guinea pig model, the single prophylactic administration of nanoscavengers effectively prevented death after repeated exposure to sarin within one week, demonstrating the translational significance of nanoscavengers in clinical and military settings.
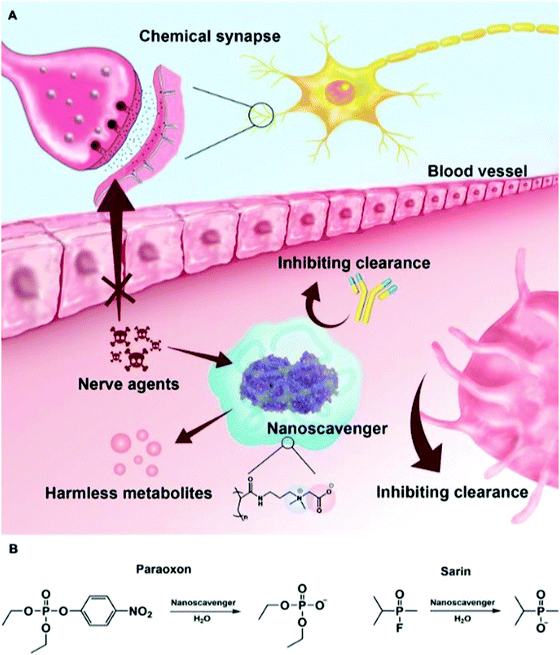 |
| Fig. 15 Biosafety materials as nanoscavengers to remove neuro-drugs. (A) Schematic illustration of the protection mechanism of nanoscavengers. (B) Hydrolysis of paraoxon and sarin mediated by nanoscavengers. Reproduced with permission from ref. 186. Copyright 2019, American Association for the Advancement of Science. | |
3.10 Biosafety materials for genetic technology
The emergence of genetic technology directly broke the original pattern of science and promoted the development of medical, agricultural and other fields. However, it also brought problems such as gene mutation and hybridization, indicating the double-sidedness of gene technology. The conventional genetic engineering technique targets the nuclear genome, resulting in problems in the proliferation of foreign genes to weedy relatives. Target delivery to specific organelles is highly desirable for plant genetic engineering, which can be achieved via nanoparticle-mediated transformation. Wong et al. designed a gene carrier based on chitosan-complexed single-walled carbon nanotubes (SWNTs) for chloroplast transformation.187 The nanotube carrier could deliver plasmid DNA to the chloroplast of different plant species without external biolistic or chemical aid, thereby rendering a chloroplast transgene delivery platform for mature plants across different species. In Fig. 16, Wang et al. summarized a series of functionalized nanomaterials that provide diverse platforms capable of traversing barriers (e.g., multilayered cell walls) to deliver exogenous plasmid pDNA and siRNA in to intact plant cells.188
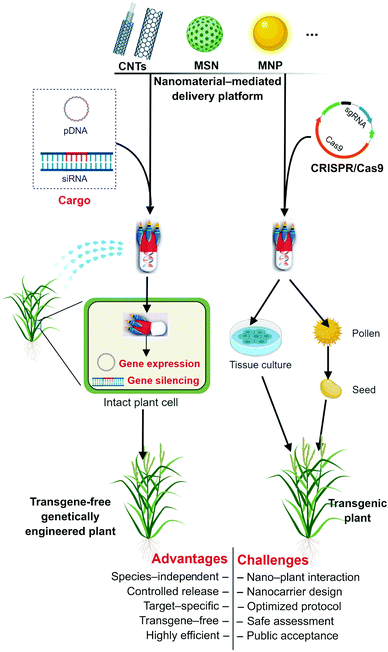 |
| Fig. 16 Biosafety materials for plant genetic engineering. Multiple nanotechnology-based materials and cargos for gene delivery have been developed, improving our ability to specifically deliver gene-editing agents. Reproduced with permission from ref. 188. Copyright 2019, Elsevier. | |
3.11 Biosafety materials for food safety
Food safety, as a global public health issue, has been regarded as a biosafety problem.189–191 The major issues in food safety are summarized as follows. (1) The presence of microorganisms directly causes mold, which can easily spread during the process of production, distribution and packaging.192–195 (2) The emergence of additives greatly helps in the manufacture of food that meets certain requirements, but if the amount of food additives used exceeds the standard level, it poses a serious threat to human health.196,197 (3) The overuse of chemical fertilizers and pesticides leads to severe pollution and food safety problems, which places a vast burden on human health.198,199 Thus, to tackle these challenges, materials scientists have proposed various solutions for food safety problems in testing, packaging, and storage.200–203
Hydrogen sulfide (H2S) with rotten egg odor is produced in rotten food. Thus, the rapid and sensitive detection of H2S is important to forewarn of food spoilage or pollution incidents with respect to this gas. Tang et al. prepared an Ag@Au core–shell nanoprobe combined with headspace single-drop microextraction (HS-SDME).204 Smartphone nanocolorimetry with the aid of a smartphone camera and color picker software was applied to detect and quantify H2S (Fig. 17). The limit of detection for this nanocolorimetric approach was as low as 65 nM, which represents an ideal in situ analytical approach for the determination of H2S. Hence, the development of biosafety materials provides technical support for food safety.
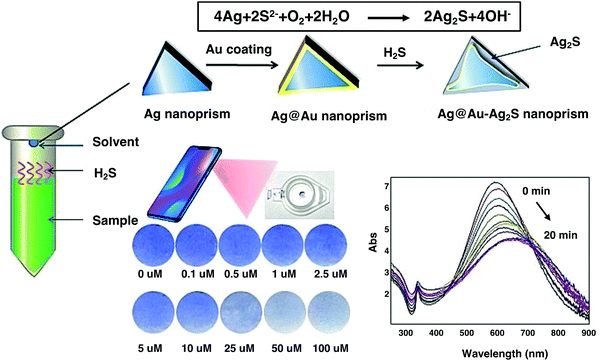 |
| Fig. 17 Biosafety materials for food safety. Schematic illustration of the H2S sensing mechanism based on the Ag@Au core–shell nanoprobe. Reproduced with permission from ref. 204. Copyright 2019, American Chemical Society. | |
3.12 Biosafety materials for aerospace safety
The space radiation environment is mainly composed of Milky Way cosmic rays, solar high-energy particles, and particles in the radiation zone of the near-Earth anomaly zone. After long-term analysis of the space radiation environment, NASA officially listed astronauts as radioactive workers in the 1980s, which indicates that aerospace safety is a biosafety problem. Doherty et al. reported a high-absorptivity/high-emissivity bone char-based thermal control surface known as SolarBlack for use on rigid and flexible metallic substrates, including titanium, aluminium, copper alloys (Fig. 18).205 This technology has been qualified for use on the front surface of the Solar Orbiter heat shield, thereby providing astronauts with biological safety protection.206
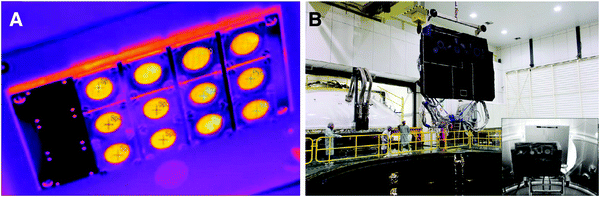 |
| Fig. 18 Biosafety materials for aerospace safety. (A) SolarBlack material samples during testing in the Synergist Temperature Accelerated Testing (STAR) facility in the ESA/ESTEC center. (B) Solar orbiter heatshield based on SolarBlack technology. Reproduced with permission from ref. 205. Copyright 2015, Elsevier. | |
The harm caused by an impact accident in a space environment can directly lead to a devastating consequence. Thus, a polymer material that can self-heal within one second after impact has been developed to solve this problem.207 The rapid reaction rates was achieved by thiol–ene-alkylborane formulations, providing astronauts with biological safety protection at the physical level, which ensures the safety of human life and improves the efficiency of related work in the process of exploring space.
4. Outlook and prospects
In summary, a variety of materials, such as polymers, MOFs, graphene, and carbon nanotubes, have dramatically changed our strategy in facing grand challenges for biosafety, but no clear definition and detailed plan on the development of biosafety materials have been given to date. Numerous reports on the progress of materials science prove its capacity to solve the difficult biosafety problems. To the best of our knowledge, we are the first to officially propose the brand-new concept of “biosafety materials” to specify the role of materials science in biosafety, aiming at raising the awareness of the scientific community to actively integrate the two different subjects and advocate the marriage of both concepts. This brand-new concept of “biosafety materials” can help solve problems related to biosafety. Since biosafety covers a wide range of topics including pathogen detection, biological invasion prevention, protection and prevention of biological resources, and genetic technology, solely relying on a single discipline to address these issues in biosafety is impossible. Thus, the integration of biosafety and materials science can significantly facilitate the development of effective biosafety materials, thus providing a powerful toolbox for professionals to solve biosafety-related problems.
Overall, despite the significant progress in recent years, the development of biosafety materials is still in the early stage, and great efforts should be made to improve the current technologies and facilitate the development of biosafety materials. Thus, the following measures are suggested.
Firstly, biosafety materials science majors should be opened in universities and research institutes to strengthen biosafety materials science disciplines, train professional teams, and direct biosafety materials professionals in basic research in the field of infectious diseases. The development of biosafety materials can support future work on the traceability and transmission of pathogens of high incidence, sudden infectious diseases, understanding infection and pathogenic mechanisms, and determining anti-infection means. Secondly, scientific research laboratories and research centers related to biosafety materials science and research platforms should be built to strengthen the integration of relevant scientific research, which can finally improve the biosafety research system. Thirdly, professional associations related to biosafety materials science should be established, and developing professional journals and magazines to expand the influence of biosafety materials science is necessary. Finally, well-known enterprises specialized in biosafety materials to develop related products and equipment for biosafety issues should be formed. Biosafety materials research and development centers could be further built in these enterprises to support human health, social stability, and national security.
Taken together, globalization makes the current biosafety threat not only a problem for a single country. No country can protect itself from biological risks without cooperation with other countries. Hence, we hope scientists worldwide will share data and information to use biosafety materials collaboratively to tackle biosafety risks. Finally, we sincerely hope that biosafety materials science will become an independent discipline in the near future, and can flourish worldwide. The government will soon pay more attention to the development of biosafety materials science. An increasing number of researchers can realize its importance and join the research community to explore more and more biosafety materials as well as related products and equipment. Ultimately, the development of biosafety materials science will provide a solid guarantee for the health and well-being of society, economic prosperity, and national security worldwide.
Conflicts of interest
There are no conflicts to declare.
Acknowledgements
This work was supported by National Natural Science Foundation of China (51873218).
Notes and references
- J. Y. Richmond, R. H. Hill, R. S. Weyant, S. L. Nesby-O'Dell and P. E. Vinson, What's hot in animal biosafety?, ILAR J., 2003, 44, 20–27 CrossRef CAS PubMed.
- M. U. Kraemer, C.-H. Yang, B. Gutierrez, C.-H. Wu, B. Klein, D. M. Pigott, L. du Plessis, N. R. Faria, R. Li and W. P. Hanage, The effect of human mobility and control measures on the COVID-19 epidemic in China, Science, 2020, 368, 493–497 Search PubMed.
- J. Thornton, Don’t forget chronic lung and immune conditions during covid-19, says WHO, BMJ, 2020, 368, m1192 Search PubMed.
- T. Wang, Z. Du, F. Zhu, Z. Cao, Y. An, Y. Gao and B. Jiang, Comorbidities and multi-organ injuries in the treatment of COVID-19, Lancet, 2020, 395, e52 CrossRef CAS.
- X. Wang, COVID-19 Epidemic and Enhancing China’s National Biosecurity System, J. Biosafety Biosecur., 2020 DOI:10.1016/j.jobb.2020.03.002.
- M. B. Forrester and S. K. Stanley, Calls about anthrax to the Texas Poison Center Network in relation to the anthrax bioterrorism attack in 2001, Vet. Hum. Toxicol., 2003, 45, 247–248 Search PubMed.
- W. H. Organization, Laboratory biosafety guidance related to coronavirus disease 2019 (COVID-19): interim guidance, 12 February 2020, World Health Organization, 2020 Search PubMed.
- O. Akan, Laboratory infections, Mikrobiyol. Bul., 1993, 27, 77–84 CAS.
- S. F. Lin, P. L. Jiang, J. S. Tsai, Y. Y. Huang, S. Y. Lin, J. H. Lin and D. Z. Liu, Surface assembly of poly (I: C) on polyethyleneimine-modified gelatin nanoparticles as immunostimulatory carriers for mucosal antigen delivery, J. Biomed. Mater. Res., Part B, 2019, 107, 1228–1237 CrossRef CAS PubMed.
- M. Lian, B. Sun, Z. Qiao, K. Zhao, X. Zhou, Q. Zhang, D. Zou, C. He and X. Zhang, Bi-layered electrospun nanofibrous membrane with osteogenic and antibacterial properties for guided bone regeneration, Colloids Surf., B, 2019, 176, 219–229 Search PubMed.
- C. Bachireddy, C. Chen and M. Dar, Securing the Safety Net and Protecting Public Health During a Pandemic: Medicaid’s Response to COVID-19, J. Am. Med. Assoc., 2020 DOI:10.1001/jama.2020.4272.
- T. Trevan, Biological research: rethink biosafety, Nat. News, 2015, 527, 155 CrossRef CAS PubMed.
-
D. P. Fidler, Medicine at the Border, Springer, 2007, pp. 196–218 Search PubMed.
- M. Liapin and V. Kutyrev, Actual problems of biosafety, Zh. Mikrobiol., Epidemiol. Immunobiol., 2013, 97–102 CAS.
- C. L. Briggs, Communicating biosecurity, Med. Anthropol., 2011, 30, 6–29 CrossRef PubMed.
- G. Borkow, S. S. Zhou, T. Page and J. Gabbay, A novel anti-influenza copper oxide containing respiratory face mask, PLoS One, 2010, 5(6), e11295 CrossRef PubMed.
- L. Bakanidze, P. Imnadze and D. Perkins, Biosafety and biosecurity as essential pillars of international health security and cross-cutting elements of biological nonproliferation, BMC Public Health, 2010, 10, S12 Search PubMed.
- L. A. Meyerson and J. K. Reaser, Biosecurity: Moving toward a comprehensive approach, Bioscience, 2002, 52, 593–600 CrossRef.
- S. E. Davies, Biosecurity interventions: global health and security in question, Int. Aff., 2009, 85, 639 Search PubMed.
- C. Humphreys, Materials science and engineering in Britain, Angew. Chem., Int. Ed. Engl., 1989, 28, 1077–1078 CrossRef.
- T. N. Nguyen, T. N. Huynh, D. Hoang, D. H. Nguyen, Q. H. Nguyen and T. H. Tran, Functional Nanostructured Oligochitosan–Silica/Carboxymethyl Cellulose Hybrid Materials: Synthesis and Investigation of Their Antifungal Abilities, Polymers, 2019, 11, 628 CrossRef PubMed.
- R. J. Nevagi, M. Skwarczynski and I. Toth, Polymers for subunit vaccine delivery, Eur. Polym. J., 2019, 114, 397–410 CrossRef CAS.
- M. Nautiyal, S. De Graef, L. Pang, B. Gadakh, S. V. Strelkov, S. D. Weeks and A. Van Aerschot, Comparative analysis of pyrimidine substituted aminoacyl-sulfamoyl nucleosides as potential inhibitors targeting class I aminoacyl-tRNA synthetases, European, J. Med. Chem., 2019, 173, 154–166 CAS.
- J. Li, Y. Yu, K. Myungwoong, K. Li, J. Mikhail, L. Zhang, C.-C. Chang, D. Gersappe, M. Simon and C. Ober, Manipulation of cell adhesion and dynamics using RGD functionalized polymers, J. Mater. Chem. B, 2017, 5, 6307–6316 Search PubMed.
- F. Eggiman, U. Meier and H. W. Fritz, EMPA and its activities in the field of building materials, elements and structures, Mater. Struct., 1995, 28, 101–102 CrossRef.
- S. Feng, C. Shen, N. Xia, W. Song, M. Fan and B. J. Cowling, Rational use of face masks in the COVID-19 pandemic, Lancet Respir. Med., 2020, 8, 434–436 CrossRef CAS PubMed.
- E. Mahase, Covid-19: UK could delay non-urgent care and call doctors back from leave and retirement, BMJ, 2020, 368, m854 CrossRef PubMed.
- E. Livingston, A. Desai and M. Berkwits, Sourcing personal protective equipment during the COVID-19 pandemic, J. Am. Med. Assoc., 2020, 323, 1912–1914 CrossRef PubMed.
- M. A. Matthay, J. M. Aldrich and J. E. Gotts, Treatment for severe acute respiratory distress syndrome from COVID-19, Lancet Respir. Med., 2020, 8, 433–434 CrossRef CAS PubMed.
- E. Mahase, Coronavirus: home testing pilot launched in London to cut hospital visits and ambulance use, BMJ, 2020, 368, m621 CrossRef PubMed.
- N. Greenberg, M. Docherty, S. Gnanapragasam and S. Wessely, Managing mental health challenges faced by healthcare workers during covid-19 pandemic, BMJ, 2020, 368, m1211 Search PubMed.
- J. Ma, X. Cheng, F. Peng, N. Zhang, R. Li, L. Sun, Z.-L. Li and H. Jiang, A polymer dots fluorescent sensor for detection of alkaline phosphatase activity and inhibitor evaluation, J. Mater. Sci., 2019, 54, 10055–10064 CrossRef CAS.
-
H. S. Maghdid, K. Z. Ghafoor, A. S. Sadiq, K. Curran and K. Rabie, A novel ai-enabled framework to diagnose coronavirus covid 19 using smartphone embedded sensors: Design study, 2020, arXiv preprint arXiv:2003.07434.
- L. Wang, Z. Yuan, H. E. Karahan, Y. Wang, X. Sui, F. Liu and Y. Chen, Nanocarbon materials in water disinfection: state-of-the-art and future directions, Nanoscale, 2019, 11, 9819–9839 RSC.
- W.-F. Lai and A. L. Rogach, Hydrogel-based materials for delivery of herbal medicines, ACS Appl. Mater. Interfaces, 2017, 9, 11309–11320 CrossRef CAS PubMed.
- D. Irvine, Material aid for vaccines, Nat. Mater., 2018, 17, 472–473 CrossRef CAS PubMed.
- S. P. Levine, M. A. Puskar, C. L. Geraci, A. A. Grote and M. Bolyard, Fourier Transform Infra-Red Spectroscopy Applied to Hazardous Waste: I-Preliminary Test of Material Analysis for Improvement of Personal Protection Strategies, Am. Ind. Hyg. Assoc. J., 1985, 46, 181–186 CrossRef CAS PubMed.
- M. Hussain, J. Wackerlig and P. A. Lieberzeit, Biomimetic strategies for sensing biological species, Biosensors, 2013, 3, 89–107 CrossRef PubMed.
- D. A. Stringfellow, M. D. Givens and J. G. Waldrop, Biosecurity issues associated with current and emerging embryo technologies, Reprod., Fertil. Dev., 2003, 16, 93–102 CrossRef.
- C. L. Hewitt, Marine biosecurity issues in the world oceans: global activities and Australian directions, Ocean Yearb. Online, 2003, 17, 193–212 Search PubMed.
- B. D. Nordmann, Issues in biosecurity and biosafety, Int. J. Antimicrob. Agents, 2010, 36, S66–S69 CrossRef CAS PubMed.
- H. Mukai and T. Nakagawa, Long and accurate PCR (LA PCR), Nihon rinsho. Jpn. J. Clin. Med., 1996, 54, 917–922 CAS.
- I. Y. Yoo, J.-Y. Kim, Y. K. Yoon, H. J. Huh and N. Y. Lee, Comparison Between the SFTS-QS Kit and the PowerChek SFTSV Real-time PCR Kit for the Detection of Severe Fever With Thrombocytopenia Syndrome Virus, Ann. Lab. Med., 2020, 40, 317–320 CrossRef PubMed.
- H. Hyodo and H. Furuta, POCT and system, Rinsho Byori. Jpn. J. Clin. Pathol., 2002, 50, 940–946 Search PubMed.
- X. Huang, J. Li, M. Lu, W. Zhang, Z. Xu, B.-Y. Yu and J. Tian, Point-of-Care Testing of MicroRNA based on Personal Glucose Meter and Dual Signal Amplification to Evaluate Drug-Induced Kidney Injury, Anal. Chim. Acta, 2020, 1112, 72–79 CrossRef CAS PubMed.
- H. Zhang, Z. Wang, Q. Zhang, F. Wang and Y. Liu, Ti3C2 MXenes nanosheets catalyzed highly efficient electrogenerated chemiluminescence biosensor for the detection of exosomes, Biosens. Bioelectron., 2019, 124, 184–190 CrossRef PubMed.
- Q. Sui, P. Li, N.-N. Yang, T. Gong, R. Bu and E.-Q. Gao, Differentiable Detection of Volatile Amines with a Viologen-Derived Metal–Organic Material, ACS Appl. Mater. Interfaces, 2018, 10, 11056–11062 CrossRef CAS PubMed.
- J. H. Kim, J. E. Park, M. Lin, S. Kim, G. H. Kim, S. Park, G. Ko and J. M. Nam, Sensitive, Quantitative Naked-Eye Biodetection with Polyhedral Cu Nanoshells, Adv. Mater., 2017, 29, 1702945 Search PubMed.
- C. Vepari and D. L. Kaplan, Silk as a biomaterial, Prog. Polym. Sci., 2007, 32, 991–1007 CrossRef CAS PubMed.
- T. Chandy and C. P. Sharma, Chitosan-as a biomaterial, Biomater., Artif. Cells Artif. Organs, 1990, 18, 1–24 CrossRef CAS PubMed.
- K. J. Burg, S. Porter and J. F. Kellam, Biomaterial developments for bone tissue engineering, Biomaterials, 2000, 21, 2347–2359 CrossRef CAS.
- A. Subramanian, U. M. Krishnan and S. Sethuraman, Development of biomaterial scaffold for nerve tissue engineering: Biomaterial mediated neural regeneration, J. Biomed. Sci., 2009, 16, 108 CrossRef PubMed.
- R. Rai, T. Keshavarz, J. Roether, A. R. Boccaccini and I. Roy, Medium chain length polyhydroxyalkanoates, promising new biomedical materials for the future, Mater. Sci. Eng., R, 2011, 72, 29–47 CrossRef.
- J. Jones and L. Hench, Biomedical materials for new millennium: perspective on the future, Mater. Sci. Technol., 2001, 17, 891–900 CrossRef CAS.
-
F. H. Silver and D. L. Christiansen, Biomaterials science and biocompatibility, Springer, 1999, pp. 1–26 Search PubMed.
- L. L. Hench and J. M. Polak, Third-generation biomedical materials, Science, 2002, 295, 1014–1017 Search PubMed.
- N. Nimi, A. Saraswathy, S. S. Nazeer, N. Francis, S. J. Shenoy and R. S. Jayasree, Biosafety of citrate coated zerovalent iron nanoparticles for Magnetic Resonance Angiography, Data Brief, 2018, 20, 1829–1835 CrossRef CAS PubMed.
- X. Huang, Y. Zhang, M. Shi, Y. Zhang and Y. Zhao, Study on a polymerizable visible light initiator for fabrication of biosafety materials, Polym. Chem., 2019, 10, 2273–2281 RSC.
- Y. Sun, W. Feng, P. Yang, C. Huang and F. Li, The biosafety of lanthanide upconversion nanomaterials, Chem. Soc. Rev., 2015, 44, 1509–1525 RSC.
- Y. Zhao, G. Xing and Z. Chai, Nanotoxicology: Are carbon nanotubes safe?, Nat. Nanotechnol., 2008, 3, 191 CrossRef CAS PubMed.
- L. Zhu, L. Wang, X. Zhang, T. Li, Y. Wang, M. A. Riaz, X. Sui, Z. Yuan and Y. Chen, Interfacial engineering of graphenic carbon electrodes by antimicrobial polyhexamethylene guanidine hydrochloride for ultrasensitive bacterial detection, Carbon, 2020, 159, 185–194 CrossRef CAS.
- J. Shi, M. Wang, Z. Sun, Y. Liu, J. Guo, H. Mao and F. Yan, Aggregation-induced emission-based ionic liquids for bacterial killing, imaging, cell labeling, and bacterial detection in blood cells, Acta Biomater., 2019, 97, 247–259 CrossRef CAS PubMed.
- Q. Li, Y. Wu, H. Lu, X. Wu, S. Chen, N. Song, Y.-W. Yang and H. Gao, Construction of supramolecular nanoassembly for responsive bacterial elimination and effective bacterial detection, ACS Appl. Mater. Interfaces, 2017, 9, 10180–10189 CrossRef CAS PubMed.
- M. S. Draz and H. Shafiee, Applications of gold nanoparticles in virus detection, Theranostics, 2018, 8, 1985 CrossRef CAS PubMed.
- B. Yang, J. Kong and X. Fang, Bandage-like wearable flexible microfluidic recombinase polymerase amplification sensor for the rapid visual detection of nucleic acids, Talanta, 2019, 204, 685–692 CrossRef CAS PubMed.
- K. Liu, D. Pan, Y. Wen, H. Zhang, J. Chao, L. Wang, S. Song, C. Fan and Y. Shi, Identifying the genotypes of hepatitis B virus (HBV) with DNA origami label, Small, 2018, 14, 1701718 CrossRef PubMed.
- Y. Yu, Q. Zhang, C.-C. Chang, Y. Liu, Z. Yang, Y. Guo, Y. Wang, D. K. Galanakis, K. Levon and M. Rafailovich, Design of a molecular imprinting biosensor with multi-scale roughness for detection across a broad spectrum of biomolecules, Analyst, 2016, 141, 5607–5617 RSC.
- V. Ricotta, Y. Yu, N. Clayton, Y.-C. Chuang, Y. Wang, S. Mueller, K. Levon, M. Simon and M. Rafailovich, A chip-based potentiometric sensor for a Zika virus diagnostic using 3D surface molecular imprinting, Analyst, 2019, 144, 4266–4280 RSC.
- Y. T. Lim, Vaccine adjuvant materials for cancer immunotherapy and control of infectious disease, Clin. Exp. Vaccine Res., 2015, 4, 54–58 CrossRef PubMed.
- K. Blecher, A. Nasir and A. Friedman, The growing role of nanotechnology in combating infectious disease, Virulence, 2011, 2, 395–401 CrossRef PubMed.
- L. Rao, R. Tian and X. Chen, Cell-Membrane-Mimicking Nanodecoys against Infectious Diseases, ACS Nano, 2020, 14, 2569–2574 CrossRef CAS PubMed.
- Z. Liu, L. Zhang, Q. Guan, Y. Guo, J. Lou, D. Lei, S. Wang, S. Chen, L. Sun and H. Xuan, Biomimetic Materials with Multiple Protective Functionalities, Adv. Funct. Mater., 2019, 29, 1901058 CrossRef.
- G. Gralewicz and B. Więcek, Active thermography in qualitative evaluation of protective materials, Int. J. Occup. Saf. Ergon., 2009, 15, 363–371 CrossRef CAS PubMed.
- N. Shimasaki, K. Shinohara and H. Morikawa, Performance of materials used for biological personal protective equipment against blood splash penetration, Ind. Health, 2017, 55, 521–528 CrossRef PubMed.
- Y. Zhang, X. Le, Y. Jian, W. Lu, J. Zhang and T. Chen, 3D Fluorescent Hydrogel Origami for Multistage Data Security Protection, Adv. Funct. Mater., 2019, 29, 1905514 CrossRef CAS.
-
P. F. Campos and T. M. Gilbert, Ancient DNA, Springer, 2012, pp. 81–85 Search PubMed.
- M. Pasha, C. Hare, M. Ghadiri, A. Gunadi and P. M. Piccione, Inter-particle coating variability in a rotary batch seed coater, Chem. Eng. Res. Des., 2017, 120, 92–101 CrossRef CAS.
- Y. Qiu, D. R. Myers and W. A. Lam, The biophysics and mechanics of blood from a materials perspective, Nat. Rev. Mater., 2019, 4, 294–311 CrossRef PubMed.
- M. R. I. Sarder, M. M. Sarker and S. K. Saha, Cryopreservation of sperm of an indigenous endangered fish species Nandus nandus (Hamilton, 1822) for ex situ conservation, Cryobiology, 2012, 65, 202–209 CrossRef PubMed.
- P. Alivisatos, The use of nanocrystals in biological detection, Nat. Biotechnol., 2004, 22, 47–52 CrossRef CAS PubMed.
- Y. Li, L. Zhao, Y. Yao and X. Guo, Single-molecule nanotechnologies: An evolution in the biological dynamics detection, ACS Appl. Bio Mater., 2020, 3, 68–85 CAS.
- Y. Yi, W. Liao, Q. Zhao and X. Lu, Separation and detection of tryptophan metabolites in biological samples, Chin. J. Chromatogr., 1999, 17, 158–161 CAS.
- L. Calvo and J. Casas, Sterilization of biological weapons in technical clothing and sensitive material by high-pressure CO2 and water, Ind. Eng. Chem. Res., 2018, 57, 4680–4687 CrossRef CAS.
- G. Zhao, C. He, D. Kumar, J. P. Hooper, G. H. Imler, D. A. Parrish and M. S. Jean'ne, 1,3,5-Triiodo-2,4,6-trinitrobenzene (TITNB) from benzene: Balancing performance and high thermal stability of functional energetic materials, Chem. Eng. J., 2019, 378, 122119 CrossRef CAS.
- M. Bray, Defense against filoviruses used as biological weapons, Antiviral Res., 2003, 57, 53–60 CrossRef CAS PubMed.
- A. J. Russell, J. A. Berberich, G. r. F. Drevon and R. R. Koepsel, Biomaterials for mediation of chemical and biological warfare agents, Annu. Rev. Biomed. Eng., 2003, 5, 1–27 CrossRef CAS PubMed.
- G. Zhao, C. He, D. Kumar, J. P. Hooper, G. H. Imler, D. A. Parrish and M. S. Jean'ne, Functional energetic biocides by coupling of energetic and biocidal polyiodo building blocks, Chem. Eng. J., 2019, 368, 244–251 CrossRef CAS.
- I. Van der Lubben, J. Verhoef, G. Borchard and H. Junginger, Chitosan for mucosal vaccination, Adv. Drug Delivery Rev., 2001, 52, 139–144 CrossRef CAS PubMed.
- B. Pulendran and R. Ahmed, Immunological mechanisms of vaccination, Nat. Immunol., 2011, 12, 509 CrossRef CAS PubMed.
- S. P. Sullivan, D. G. Koutsonanos, M. del Pilar Martin, J. W. Lee, V. Zarnitsyn, S.-O. Choi, N. Murthy, R. W. Compans, I. Skountzou and M. R. Prausnitz, Dissolving polymer microneedle patches for influenza vaccination, Nat. Med., 2010, 16, 915 CrossRef CAS PubMed.
- L. O. De Serrano and D. J. Burkhart, Liposomal vaccine formulations as prophylactic agents: design considerations for modern vaccines, J. Nanobiotechnol., 2017, 15, 83 CrossRef PubMed.
- M. A. Islam, E. K. Reesor, Y. Xu, H. R. Zope, B. R. Zetter and J. Shi, Biomaterials for mRNA delivery, Biomater. Sci., 2015, 3, 1519–1533 RSC.
- P. S. Kowalski, A. Rudra, L. Miao and D. G. Anderson, Delivering the Messenger: Advances in Technologies for Therapeutic mRNA Delivery, Mol. Ther., 2019, 27, 710–728 CrossRef CAS PubMed.
- N. B. Tsui, E. K. Ng and Y. M. Lo, Stability of endogenous and added RNA in blood specimens, serum, and plasma, Clin. Chem., 2002, 48, 1647–1653 Search PubMed.
- J. Houseley and D. Tollervey, The many pathways of RNA degradation, Cell, 2009, 136, 763–776 CrossRef CAS PubMed.
- J. Zhang, H. Zeng, J. Gu, H. Li, L. Zheng and Q. Zou, Progress and Prospects on Vaccine Development against SARS-CoV-2, Vaccines, 2020, 8, 153 CrossRef PubMed.
- T. T. Le, Z. Andreadakis, A. Kumar, R. G. Román, S. Tollefsen, M. Saville and S. Mayhew, The COVID-19 vaccine development landscape, Nat. Rev. Drug Discovery, 2020, 19, 305–306 CrossRef PubMed.
- J. Cohen, Vaccine designers take first shots at COVID-19, Science, 2020, 368, 14–16 CrossRef CAS PubMed.
- J. E. Dahlman, C. Barnes, O. Khan, A. Thiriot, S. Jhunjunwala, T. E. Shaw, Y. Xing, H. B. Sager, G. Sahay, L. Speciner, A. Bader, R. L. Bogorad, H. Yin, T. Racie, Y. Dong, S. Jiang, D. Seedorf, A. Dave, K. S. Sandu, M. J. Webber, T. Novobrantseva, V. M. Ruda, A. K. R. Lytton-Jean, C. G. Levins, B. Kalish, D. K. Mudge, M. Perez, L. Abezgauz, P. Dutta, L. Smith, K. Charisse, M. W. Kieran, K. Fitzgerald, M. Nahrendorf, D. Danino, R. M. Tuder, U. H. von Andrian, A. Akinc, A. Schroeder, D. Panigrahy, V. Kotelianski, R. Langer and D. G. Anderson, In vivo endothelial siRNA delivery using polymeric nanoparticles with low molecular weight, Nat. Nanotechnol., 2014, 9, 648–655 CrossRef CAS PubMed.
- X. Han, S. Shen, Q. Fan, G. Chen, E. Archibong, G. Dotti, Z. Liu, Z. Gu and C. Wang, Red blood cell-derived nanoerythrosome for antigen delivery with enhanced cancer immunotherapy, Sci. Adv., 2019, 5, eaaw6870 CrossRef PubMed.
- A. Tan, H. De La Pena and A. M. Seifalian, The application of exosomes as a nanoscale cancer vaccine, Int. J. Nanomed., 2010, 5, 889–900 CAS.
- Y. C. Kim, J. H. Park and M. R. Prausnitz, Microneedles for drug and vaccine delivery, Adv. Drug Delivery Rev., 2012, 64, 1547–1568 CrossRef CAS PubMed.
- L. C. Burnett, G. Lunn and R. Coico, Biosafety: guidelines for working with pathogenic and infectious microorganisms, Curr. Protoc. Microbiol., 2009, 13, 1A.1.1–1A.1.14 Search PubMed.
- Y.-T. Yeh, K. Gulino, Y. Zhang, A. Sabestien, T.-W. Chou, B. Zhou, Z. Lin, I. Albert, H. Lu and V. Swaminathan, A rapid and label-free platform for virus capture and identification from clinical samples, Proc. Natl. Acad. Sci. U. S. A., 2020, 117, 895–901 Search PubMed.
- F. Sun, A. Ganguli, J. Nguyen, R. Brisbin, K. Shanmugam, D. L. Hirschberg, M. B. Wheeler, R. Bashir, D. M. Nash and B. T. Cunningham, Smartphone-based multiplex 30-minute nucleic acid test of live virus from nasal swab extract, Lab Chip, 2020, 20, 1621–1627 RSC.
- F. Zang, Z. Su, L. Zhou, K. Konduru, G. Kaplan and S. Y. Chou, Ultrasensitive Ebola Virus Antigen Sensing via 3D Nanoantenna Arrays, Adv. Mater., 2019, 31, e1902331 CrossRef PubMed.
- Y.-T. Yeh, Y. Tang, A. Sebastian, A. Dasgupta, N. Perea-Lopez, I. Albert, H. Lu, M. Terrones and S.-Y. Zheng, Tunable and label-free virus enrichment for ultrasensitive virus detection using carbon nanotube arrays, Sci. Adv., 2016, 2, e1601026 CrossRef PubMed.
- P. Zhu, Y. Chen and J. Shi, Nanoenzyme-augmented cancer sonodynamic therapy by catalytic tumor oxygenation, ACS Nano, 2018, 12, 3780–3795 CrossRef CAS PubMed.
- L. Wang, L. Miao, H. Yang, J. Yu, Y. Xie, L. Xu and Y. Song, A novel nanoenzyme based on Fe3O4 nanoparticles@ thionine-imprinted polydopamine for electrochemical biosensing, Sens. Actuators, B, 2017, 253, 108–114 CrossRef CAS.
- S. R. Ahmed, J. C. Corredor, E. Nagy and S. Neethirajan, Amplified visual immunosensor integrated with nanozyme for ultrasensitive detection of avian influenza virus, Nanotheranostics, 2017, 1, 338–345 CrossRef PubMed.
- D. Duan, K. Fan, D. Zhang, S. Tan, M. Liang, Y. Liu, J. Zhang, P. Zhang, W. Liu, X. Qiu, G. P. Kobinger, G. F. Gao and X. Yan, Nanozyme-strip for rapid local diagnosis of Ebola, Biosens. Bioelectron., 2015, 74, 134–141 CrossRef CAS PubMed.
- A. Giraud, I. Matic, M. Radman, M. Fons and F. Taddei, Mutator bacteria as a risk factor in treatment of infectious diseases, Antimicrob. Agents Chemother., 2002, 46, 863–865 CrossRef CAS PubMed.
-
A. W. Stableforth and I. A. Galloway, Infectious diseases of animals. Diseases due to Bacteria, Butterworths Scientific Publications, London, 1959, vol. 2 Search PubMed.
- M. Xiao, K. Zou, L. Li, L. Wang, Y. Tian, C. Fan and H. Pei, Stochastic DNA Walkers in Droplets for Super-Multiplexed Bacterial Phenotype Detection, Angew. Chem., Int. Ed., 2019, 58, 15448–15454 Search PubMed.
- Y. Hu, Y. He, Y. Han, Y. Ge, G. Song and J. Zhou, Determination of the activity of alkaline phosphatase based on aggregation-induced quenching of the fluorescence of copper nanoclusters, Microchim. Acta, 2019, 186, 5 CrossRef PubMed.
- X. Gu, G. Zhang and D. Zhang, A new ratiometric fluorescence detection of heparin based on the combination of the aggregation-induced fluorescence quenching and enhancement phenomena, Analyst, 2012, 137, 365–369 RSC.
- Y. Hong, J. W. Lam and B. Z. Tang, Aggregation-induced emission, Chem. Soc. Rev., 2011, 40, 5361–5388 RSC.
- A. Qin, J. W. Lam and B. Z. Tang, Luminogenic polymers with aggregation-induced emission characteristics, Prog. Polym. Sci., 2012, 37, 182–209 CrossRef CAS.
- X. He, Y. Yang, Y. Guo, S. Lu, Y. Du, J.-J. Li, X. Zhang, N. L. Leung, Z. Zhao and G. Niu, Phage-Guided Targeting, Discriminative Imaging, and Synergistic Killing of Bacteria by AIE Bioconjugates, J. Am. Chem. Soc., 2020, 142, 3959–3969 CrossRef CAS PubMed.
- A. Panigrahi, V. N. Are, S. Jain, D. Nayak, S. Giri and T. K. Sarma, Cationic Organic Nanoaggregates as AIE Luminogens for Wash-Free Imaging of Bacteria and Broad-Spectrum Antimicrobial Application, ACS Appl. Mater. Interfaces, 2020, 12, 5389–5402 CrossRef CAS PubMed.
- M. Kang, R. T. Kwok, J. Wang, H. Zhang, J. W. Lam, Y. Li, P. Zhang, H. Zou, X. Gu and F. Li, A multifunctional luminogen with aggregation-induced emission characteristics for selective imaging and photodynamic killing of both cancer cells and Gram-positive bacteria, J. Mater. Chem. B, 2018, 6, 3894–3903 RSC.
- M. Wu, G. Qi, X. Liu, Y. Duan, J. Liu and B. Liu, Bio-Orthogonal AIEgen for Specific Discrimination and Elimination of Bacterial Pathogens via Metabolic Engineering, Chem. Mater., 2019, 32, 858–865 CrossRef.
- T. Zhou, R. Hu, L. Wang, Y. Qiu, G. Zhang, Q. Deng, H. Zhang, P. Yin, B. Situ and C. Zhan, AIE conjugated polymer with ultra-strong ROS generation ability and great biosafety for efficient therapy of bacterial infection, Angew. Chem., Int. Ed., 2020, 55, 16704–16710 Search PubMed.
- V. Müller, J. M. Sousa, H. C. Koydemir, M. Veli, D. Tseng, L. Cerqueira, A. Ozcan, N. F. Azevedo and F. Westerlund, Identification of pathogenic bacteria in complex samples using a smartphone based fluorescence microscope, RSC Adv., 2018, 8, 36493–36502 RSC.
- R. Guo, C. McGoverin, S. Swift and F. Vanholsbeeck, A rapid and low-cost estimation of bacteria counts in solution using fluorescence spectroscopy, Anal. Bioanal. Chem., 2017, 409, 3959–3967 CrossRef CAS PubMed.
- X. Huang, Y. Yang, J. Shi, H. T. Ngo, C. Shen, W. Du and Y. Wang, High-Internal-Phase Emulsion Tailoring Polymer Amphiphilicity towards an Efficient NIR-Sensitive Bacteria Filter, Small, 2015, 11, 4876–4883 CrossRef CAS PubMed.
- S. Deshmukh, S. Patil, S. Mullani and S. Delekar, Silver nanoparticles as an effective disinfectant: a review, Mater. Sci. Eng., C, 2019, 97, 954–965 CrossRef CAS PubMed.
- F. Huang, Y. Gao, Y. Zhang, T. Cheng, H. Ou, L. Yang, J. Liu, L. Shi and J. Liu, Silver-decorated polymeric micelles combined with curcumin for enhanced antibacterial activity, ACS Appl. Mater. Interfaces, 2017, 9, 16880–16889 CrossRef CAS PubMed.
- A. Scalbert, Antimicrobial properties of tannins, Phytochemistry, 1991, 30, 3875–3883 CrossRef CAS.
- W. F. Broekaert, B. P. Cammue, M. F. De Bolle, K. Thevissen, G. W. De Samblanx, R. W. Osborn and K. Nielson, Antimicrobial peptides from plants, Crit. Rev. Plant Sci., 1997, 16, 297–323 CrossRef CAS.
- G. Qing, X. Zhao, N. Gong, J. Chen, X. Li, Y. Gan, Y. Wang, Z. Zhang, Y. Zhang and W. Guo, Thermo-responsive triple-function nanotransporter for efficient chemo-photothermal therapy of multidrug-resistant bacterial infection, Nat. Commun., 2019, 10, 1–12 Search PubMed.
- H. Zheng, Z. Ji, K. R. Roy, M. Gao, Y. Pan, X. Cai, L. Wang, W. Li, C. H. Chang and C. Kaweeteerawat, Engineered Graphene Oxide Nanocomposite Capable of Preventing the Evolution of Antimicrobial Resistance, ACS Nano, 2019, 13, 11488–11499 CrossRef CAS PubMed.
- M. L. Ranney, V. Griffeth and A. K. Jha, Critical supply shortages—the need for ventilators and personal protective equipment during the Covid-19 pandemic, N. Engl. J. Med., 2020, 382, 41–44 Search PubMed.
- W. H. Organization, Rational use of personal protective equipment for coronavirus disease (COVID-19) and considerations during severe shortages: interim guidance, 6 April 2020, World Health Organization, 2020 Search PubMed.
- H. Zhong, Z. Zhu, J. Lin, C. F. Cheung, V. L. Lu, F. Yan, C.-Y. Chan and G. Li, Reusable and Recyclable Graphene Masks with Outstanding Superhydrophobic and Photothermal Performances, ACS Nano, 2020 DOI:10.1021/acsnano.0c02250.
- A. Konda, A. Prakash, G. A. Moss, M. Schmoldt, G. D. Grant and S. Guha, Aerosol filtration efficiency of common fabrics used in respiratory cloth masks, ACS Nano, 2020 DOI:10.1021/acsnano.0c03252.
- P. Li, J. Li, X. Feng, J. Li, Y. Hao, J. Zhang, H. Wang, A. Yin, J. Zhou and X. Ma, Metal-organic frameworks with photocatalytic bactericidal activity for integrated air cleaning, Nat. Commun., 2019, 10, 1–10 CrossRef PubMed.
- G. Liu, J. Nie, C. Han, T. Jiang, Z. Yang, Y. Pang, L. Xu, T. Guo, T. Bu and C. Zhang, Self-powered electrostatic adsorption face mask based on a triboelectric nanogenerator, ACS Appl. Mater. Interfaces, 2018, 10, 7126–7133 CrossRef CAS PubMed.
- V. Parthasarathi and G. Thilagavathi, Development of plasma enhanced antiviral surgical gown for healthcare workers, Fashion Text., 2015, 2, 4–16 CrossRef.
- A. Nel, Air pollution-related illness: effects of particles, Science, 2005, 308, 804–806 CrossRef CAS PubMed.
- B. Brunekreef and S. T. Holgate, Air pollution and health, Lancet, 2002, 360, 1233–1242 CrossRef CAS.
- M. S. Shafeeyan, W. M. A. W. Daud, A. Houshmand and A. Shamiri, A review on surface modification of activated carbon for carbon dioxide adsorption, J. Anal. Appl. Pyrolysis, 2010, 89, 143–151 CrossRef CAS.
- Z. Feng, Z. Long and T. Yu, Filtration characteristics of fibrous filter following an electrostatic precipitator, J. Electrost., 2016, 83, 52–62 Search PubMed.
-
D. Provenzano, Y. J. Rao, K. Mitic, S. N. Obaid, J. Berger, S. Goyal and M. H. Loew, Alternative Qualitative Fit Testing Method for N95 Equivalent Respirators in the Setting of Resource Scarcity at the George Washington University, 2020, medRxiv, DOI:10.1101/2020.04.06.20055368.
- S. Au, C. Gomersall, P. Leung and P. Li, A randomised controlled pilot study to compare filtration factor of a novel non-fit-tested high-efficiency particulate air (HEPA) filtering facemask with a fit-tested N95 mask, J. Hosp. Infect., 2010, 76, 23–25 CrossRef CAS PubMed.
- M. Cai, H. Li, S. Shen, Y. Wang and Q. Yang, Customized design and 3D printing of face seal for an N95 filtering facepiece respirator, J. Occup. Environ. Hyg., 2018, 15, 226–234 CrossRef PubMed.
- A. Deplazes-Zemp, ‘Genetic resources’, an analysis of a multifaceted concept, Biol. Conserv., 2018, 222, 86–94 CrossRef.
- T. Abramishvili and N. Chkhaidze, The role of bioagents in protection of wheat genetic resources, Commun. Agric. Appl. Biol. Sci., 2009, 74, 401–406 Search PubMed.
-
R. Scholl, L. Rivero-Lepickas and D. Crist, Arabidopsis Protocols, Springer, 1998, pp. 1–12 Search PubMed.
- C. Walters, P. Berjak, N. Pammenter, K. Kennedy and P. Raven, PLANT SCIENCE: Preservation of Recalcitrant Seeds, Science, 2013, 339, 915–916 CrossRef CAS PubMed.
-
K. Semagn, Molecular Plant Taxonomy, Springer, 2014, pp. 53–67 Search PubMed.
- F. S. Chiou, C. Y. Pai, Y. P. P. Hsu, C. W. Tsai and C. H. Yang, Extraction of human DNA for PCR from chewed residues of betel quid using a novel “PVP/CTAB” method, J. Forensic Sci., 2001, 46, 1174–1179 CAS.
- M. Guamán-Balcázar, A. Montes, C. Pereyra and E. M. de la Ossa, Production of submicron particles of the antioxidants of mango leaves/PVP by supercritical antisolvent extraction process, J. Supercrit. Fluids, 2019, 143, 294–304 CrossRef.
- L. Dennany, R. J. Förster, B. White, M. Smyth and J. F. Rusling, Direct electrochemiluminescence detection of oxidized DNA in ultrathin films containing [Os(bpy)2(PVP)10]2+, J. Am. Chem. Soc., 2004, 126, 8835–8841 CrossRef CAS PubMed.
- I.-K. Park, J.-E. Ihm, Y. Park, Y. Choi, S. Kim, W. Kim, T. Akaike and C. Cho, Galactosylated chitosan (GC)-graft-poly(vinyl pyrrolidone) (PVP) as hepatocyte-targeting DNA carrier: preparation and physicochemical characterization of GC-graft-PVP/DNA complex (1), J. Controlled Release, 2003, 86, 349–359 CrossRef CAS.
- X. Yu, Q. Luo, K. Huang, G. Yang and G. He, Prospecting for microelement function and biosafety assessment of transgenic cereal plants, Frontiers in plant, Science, 2018, 9, 326 Search PubMed.
- G. N. Y. Lemgo, S. Sabbadini, T. Pandolfini and B. Mezzetti, Biosafety considerations of RNAi-mediated virus resistance in fruit-tree cultivars and in rootstock, Transgenic Res., 2013, 22, 1073–1088 CrossRef CAS PubMed.
- A. T. Zvinavashe, E. Lim, H. Sun and B. Marelli, A bioinspired approach to engineer seed microenvironment to boost germination and mitigate soil salinity, Proc. Natl. Acad. Sci. U. S. A., 2019, 116, 25555–25561 CrossRef CAS PubMed.
- P. Tseng, B. Napier, S. Zhao, A. N. Mitropoulos, M. B. Applegate, B. Marelli, D. L. Kaplan and F. G. Omenetto, Directed assembly of bio-inspired hierarchical materials with controlled nanofibrillar architectures, Nat. Nanotechnol., 2017, 12, 474 CrossRef CAS PubMed.
- A. Matsumoto, A. Lindsay, B. Abedian and D. L. Kaplan, Silk fibroin solution properties related to assembly and structure, Macromol. Biosci., 2008, 8, 1006–1018 CrossRef CAS PubMed.
- B. Marelli, M. Brenckle, D. L. Kaplan and F. G. Omenetto, Silk fibroin as edible coating for perishable food preservation, Sci. Rep., 2016, 6, 25263 CrossRef CAS PubMed.
- H.-J. Jin and D. L. Kaplan, Mechanism of silk processing in insects and spiders, Nature, 2003, 424, 1057–1061 CrossRef CAS PubMed.
- E. Porcu, R. Fabbri, R. Seracchioli, P. M. Ciotti, O. Magrini and C. Flamigni, Birth of a healthy female after intracytoplasmic sperm injection of cryopreserved human oocytes, Fertil. Steril., 1997, 68, 724–726 CrossRef CAS PubMed.
- Y. Wang, X. Yao, S. Wu, Q. Li, J. Lv, J. Wang and L. Jiang, Bioinspired solid organogel materials with a regenerable sacrificial alkane surface layer, Adv. Mater., 2017, 29, 1700865 CrossRef PubMed.
- I. Jarić, T. Heger, F. C. Monzon, J. M. Jeschke, I. Kowarik, K. R. McConkey, P. Pyšek, A. Sagouis and F. Essl, Crypticity in biological invasions, Trends Eol. Evol., 2019, 34, 291–302 CrossRef PubMed.
- T. M. Blackburn, P. Pyšek, S. Bacher, J. T. Carlton, R. P. Duncan, V. C. Jarošík, J. R. Wilson and D. M. Richardson, A proposed unified framework for biological invasions, Trends Ecol. Evol., 2011, 26, 333–339 CrossRef PubMed.
- A. Tayeh, R. A. Hufbauer, A. Estoup, V. Ravigné, L. A. Frachon and B. Facon, Biological invasion and biological control select for different life histories, Nat. Commun., 2015, 6, 1–5 Search PubMed.
- C. L. Bellard, J.-F. O. Rysman, B. Leroy, C. Claud and G. M. Mace, A global picture of biological invasion threat on islands, Nat. Ecol. Evol., 2017, 1, 1862–1869 CrossRef.
- D. Simberloff, J.-L. Martin, P. Genovesi, V. Maris, D. A. Wardle, J. Aronson, F. Courchamp, B. Galil, E. García-Berthou and M. Pascal, Impacts of biological invasions: what's what and the way forward, Trends Ecol. Evol., 2013, 28, 58–66 CrossRef PubMed.
- L. P. Xu, Y. Chen, G. Yang, W. Shi, B. Dai, G. Li, Y. Cao, Y. Wen, X. Zhang and S. Wang, Ultratrace DNA detection based on the condensing-enrichment effect of superwettable microchips, Adv. Mater., 2015, 27, 6878–6884 CrossRef CAS PubMed.
- H. Y. El-Kassas and M. G. Ghobrial, Biosynthesis of metal nanoparticles using three marine plant species: anti-algal efficiencies against “Oscillatoria simplicíssima”, Environ. Sci. Pollut. Res., 2017, 24, 7837–7849 CrossRef CAS PubMed.
- G. Rajakumar and A. A. Rahuman, Larvicidal activity of synthesized silver nanoparticles using Eclipta prostrata leaf extract against filariasis and malaria vectors, Acta Trop., 2011, 118, 196–203 CrossRef CAS PubMed.
- Y. Y. Song, J. J. Zhou, J. B. Fan, W. Z. Zhai, J. X. Meng and S. T. Wang, Hydrophilic/Oleophilic Magnetic Janus Particles for the Rapid and Efficient Oil-Water
Separation, Adv. Funct. Mater., 2018, 28, 1802493 CrossRef.
- C. Ren, J. Shen, F. Chen and H. Zeng, Rapid Room-Temperature Gelation of Crude Oils by a Wetted Powder Gelator, Angew. Chem., Int. Ed., 2017, 56, 3847–3851 Search PubMed.
- X.-J. Ju, S.-B. Zhang, M.-Y. Zhou, R. Xie, L. Yang and L.-Y. Chu, Novel heavy-metal adsorption material: ion-recognition P(NIPAM-co-BCAm) hydrogels for removal of lead(II) ions, J. Hazard. Mater., 2009, 167, 114–118 Search PubMed.
- M. Lian, Q. Feng, L. Wang, L. Niu, Z. Zhao, X. Li and Z. Zhang, Highly effective immobilization of Pb and Cd in severely contaminated soils by environment-compatible, mercapto-functionalized reactive nanosilica, J. Cleaner Prod., 2019, 235, 583–589 CrossRef CAS.
- L. R. R. Souza, L. C. Pomarolli and M. A. M. S. da Veiga, From classic methodologies to application of nanomaterials for soil remediation: an integrated view of methods for decontamination of toxic metal(oid)s, Environ. Sci. Pollut. Res., 2020, 27, 10205–10227 CrossRef PubMed.
- Z. Peng, X. Liu, W. Zhang, Z. Zeng, Z. Liu, C. Zhang, Y. Liu, B. Shao, Q. Liang and W. Tang, Advances in the application, toxicity and degradation of carbon nanomaterials in environment: a review, Environ. Int., 2020, 134, 105298 CrossRef CAS PubMed.
- K. Kerwat, S. Becker, H. Wulf and D. Densow, Biological weapons, Dtsch. Med. Wochenschr., 2010, 135, 1612–1616 CrossRef CAS PubMed.
- A. Akçali, Viruses as biological weapons, Mikrobiyol. Bul., 2005, 39, 383–397 Search PubMed.
- G. Pappas, P. Panagopoulou, L. Christou and N. Akritidis, Biological weapons, Cell. Mol. Life Sci., 2006, 63, 2229–2236 CrossRef CAS PubMed.
- K. Luan, R. Meng, C. Shan, J. Cao, J. Jia, W. Liu and Y. Tang, Terbium Functionalized Micelle Nanoprobe for Ratiometric Fluorescence Detection of Anthrax Spore Biomarker, Anal. Chem., 2018, 90, 3600–3607 Search PubMed.
- D. J. Bower, R. J. Hart, P. A. Matthews and M. E. Howden, Nonprotein neurotoxins, Clin. Toxicol., 1981, 18, 813–863 CrossRef CAS PubMed.
- J. Segura-Aguilar and R. M. Kostrzewa, Neurotoxins and neurotoxicity mechanisms. An overview, Neurotoxic. Res., 2006, 10, 263–285 CrossRef CAS PubMed.
- B. Poulain, La neurotoxine botulinique, Rev. Neurol., 2010, 166, 7–20 CrossRef CAS PubMed.
- P. Zhang, E. J. Liu, C. Tsao, S. A. Kasten, M. V. Boeri, T. L. Dao, S. J. DeBus, C. L. Cadieux, C. A. Baker and T. C. Otto, Nanoscavenger provides long-term prophylactic protection against nerve agents in rodents, Sci. Transl. Med., 2019, 11, eaau7091 Search PubMed.
- S. Y. Kwak, T. T. S. Lew, C. J. Sweeney, V. B. Koman, M. H. Wong, K. Bohmert-Tatarev, K. D. Snell, J. S. Seo, N. H. Chua and M. S. Strano, Chloroplast-selective gene delivery and expression in planta using chitosan-complexed single-walled carbon nanotube carriers, Nat. Nanotechnol., 2019, 14, 447–455 CrossRef CAS PubMed.
- P. Wang, F.-J. Zhao and P. M. Kopittke, Engineering crops without genome integration using nanotechnology, Trends Plant Sci., 2019, 24, 574–577 Search PubMed.
- M. S. Brewer, G. K. Sprouls and C. Russon, Consumer attitudes toward food safety issues, J. Food Saf., 1994, 14, 63–76 CrossRef.
- S. Miles, M. Brennan, S. Kuznesof, M. Ness, C. Ritson and L. J. Frewer, Public worry about specific food safety issues, Br. Food J., 2004, 92, 9–22 CrossRef.
- A. R. Shalaby, Significance of biogenic amines to food safety and human health, Food Res. Int., 1996, 29, 675–690 CrossRef CAS.
- A. Röhr, K. Lüddecke, S. Drusch, M. J. Müller and R. Alvensleben, Food quality and safety–consumer perception and public health concern, Food Control, 2005, 16, 649–655 CrossRef.
- S. P. Oliver, B. M. Jayarao and R. A. Almeida, Foodborne pathogens in milk and the dairy farm environment: food safety and public health implications, Foodbourne Pathog. Dis., 2005, 2, 115–129 CrossRef CAS PubMed.
- J.-C. Ogier and P. Serror, Safety assessment of dairy microorganisms: the Enterococcus genus, Int. J. Food Microbiol., 2008, 126, 291–301 CrossRef CAS PubMed.
- D. Rodríguez-Lázaro, B. Lombard, H. Smith, A. Rzezutka, M. D'Agostino, R. Helmuth, A. Schroeter, B. Malorny, A. Miko and B. Guerra, Trends in analytical methodology in food safety and quality: monitoring microorganisms and genetically modified organisms, Trends Food Sci. Technol., 2007, 18, 306–319 CrossRef.
- S. He, W. Xie, W. Zhang, L. Zhang, Y. Wang, X. Liu, Y. Liu and C. Du, Multivariate qualitative analysis of banned additives in food safety using surface enhanced Raman scattering spectroscopy, Spectrochim. Acta, Part A, 2015, 137, 1092–1099 Search PubMed.
- L. Tollefson, Monitoring adverse reactions to food additives in the US Food and Drug Administration, Regul. Toxicol. Pharmacol., 1988, 8, 438–446 CrossRef CAS PubMed.
- F. Arduini, S. Cinti, V. Scognamiglio and D. Moscone, Nanomaterials in electrochemical biosensors for pesticide detection: advances and challenges in food analysis, Microchim. Acta, 2016, 183, 2063–2083 CrossRef CAS.
- C. K. Winter and E. A. Jara, Pesticide food safety standards as companions to tolerances and maximum residue limits, J. Integr. Agric., 2015, 14, 2358–2364 Search PubMed.
- K. Weidemaier, E. Carruthers, A. Curry, M. Kuroda, E. Fallows, J. Thomas, D. Sherman and M. Muldoon, Real-time pathogen monitoring during enrichment: a novel nanotechnology-based approach to food safety testing, Int. J. Food Microbiol., 2015, 198, 19–27 CrossRef CAS PubMed.
- A. Mousavi, M. Sarhadi, S. Fawcett, S. Bowles and M. York, Tracking and traceability solution using a novel material handling system, Innovative Food Sci. Emerging Technol., 2005, 6, 91–105 CrossRef.
- T. McHugh and E. Senesi, Apple wraps: A novel method to improve the quality and extend the shelf life of fresh-cut apples, J. Food Sci., 2000, 65, 480–485 CrossRef CAS.
- D. Liu and N. Gu, Nanomaterials for fresh-keeping and sterilization in food preservation, Recent Pat. Food, Nutr. Agric., 2009, 1, 149–154 Search PubMed.
- S. Tang, T. Qi, D. Xia, M. Xu, M. Xu, A. Zhu, W. Shen and H. K. Lee, Smartphone Nanocolorimetric Determination of Hydrogen Sulfide in Biosamples after Silver-Gold Core-Shell Nanoprism-Based Headspace Single-Drop Microextraction, Anal. Chem., 2019, 91, 5888–5895 CrossRef CAS PubMed.
- K. A. Doherty, J. G. Carton, A. Norman, T. McCaul, B. Twomey and K. T. Stanton, A thermal control surface for the Solar Orbiter, Acta Astronaut., 2015, 117, 430–439 Search PubMed.
- T. Ghidini, Materials for space exploration and settlement, Nat. Mater., 2018, 17, 846–850 CrossRef CAS PubMed.
- S. R. Zavada, N. R. McHardy, K. L. Gordon and T. F. Scott, Rapid, Puncture-Initiated Healing via Oxygen-Mediated Polymerization, ACS Macro Lett., 2015, 4, 819–824 CrossRef CAS.
Footnote |
† These authors contributed equally to this work. |
|
This journal is © the Partner Organisations 2020 |