A nopinone based multi-functional probe for colorimetric detection of Cu2+ and ratiometric detection of Ag+†
Received
13th July 2019
, Accepted 18th November 2019
First published on 19th November 2019
Abstract
A dual-signal probe PPN based on the natural β-pinene derivative nopinone was synthesized for the colorimetric detection of Cu2+ and ratiometric detection of Ag+. Upon the addition of Ag+, a significant fluorescence change from blue to green was observed with a low detection limit (0.86 μM). However, upon the addition of Cu2+, a significant color change from colorless to yellow was observed with a low detection limit (0.56 μM). The novel probe PPN was applied as a probe for the colorimetric detection of Cu2+ and ratiometric detection of Ag+ with a high selectivity, good sensitivity and fast response time. The detection mechanisms of probe PPN for Cu2+/Ag+ were confirmed by 1H NMR and HRMS-ESI. Besides, probe PPN could sense Cu2+/Ag+ on test strips. Additionally, probe PPN could be applied to quantitatively detect the concentration of Ag+ in water samples and image Ag+ in living cells.
Introduction
Due to their unique physical and chemical properties, silver-containing compounds are widely used in many industries, such as photographic, pharmaceutical, electronic and imaging industries.1 Excessive usage of silver-containing compounds lead to the enhancement of Ag+ concentration in environmental water, which can affect agriculture and food safety.2,3 Meanwhile, excessive levels of Ag+ in the body can cause cardiac enlargement, skin damage and growth retardation.4–6 The United States Environmental Protection Agency (USEPA) has prescribed a safe limit value for Ag+ to be 100 mg L−1 in drinking water.7 As the third abundant transition metal element (after iron and zinc) in living organism,8 Cu2+ plays a crucial role in living organisms, such as signal transduction, energy generation, and free radical detoxification.9–11 However, excessive levels of Cu2+ in human bodies can also cause several neurodegenerative diseases, such as Wilson's disease, Parkinson's disease, and Alzheimer's disease.12,13 In addition, the irregular levels of Cu2+ can lead to irreversible damage to entire ecosystem. The U.S. Environmental Protection Agency (EPA) has prescribed a safe limit value of Cu2+ to be 20 μM in drinking water.14 To develop a dual-signal probe for Cu2+/Ag+ is very important to human health and environment protection.
Compared with traditional analytical methods,15–17 fluorescence is a simple, rapid, highly selective and sensitive method.18–23 Thus, many probes for separate detection of Cu2+ or Ag+ have been reported; these reported probes exhibit good sensing performances, such as an excellent sensitivity, high selectivity and real-time detection.24–37 However, a dual-signal probe can enhance the detection efficiency and save a lot of time and money spent on the synthesis of probe. Up to now, there are many reports on dual-signal probes.38–43 However, reported dual-site probes for the simultaneous detection of Ag+ and Cu2+ are still rare. So, developing a dual-site probe for Ag+ and Cu2+ is particularly urgent.
Nopinone is obtained by the oxidation of β-pinene, which is the main ingredient of natural turpentine. Nopinone has been applied to prepare many products, such as anesthetics, shift reagents, antitumor agents and catalysts.44–46 However, there are few reported fluorescence probes based on nopinone.47,48 Fluorescent probes based on nopinone have a good biological compatibility and low cytotoxicity.49 Thus, fluorescent probes based on nopinone have good application prospects.
Considering the aforesaid facts, we aimed to develop a nopinone-based probe for the colorimetric detection of Cu2+ and ratiometric detection of Ag+. The phenyl group was selected as the electron-donating group, and pyridine and imidazole were selected as the receptors. As expected, the probe PPN toward Cu2+ exhibited obviously visible color change from colorless to yellow. At the same time, probe PPN toward Ag+ exhibited a distinct fluorescent color change from blue to green. Moreover, probe PPN could determine the concentration of Cu2+ on test strips. Additionally, probe PPN was applied not only to determine the concentration of Ag+ in water samples, but also to image Ag+ in living cells.
Experimental
Materials and measurements
All the solvents were purchased from commercial suppliers and used without further purification. The reaction process was monitored with TLC using silica plates. All pH measurements were tested by a Sartorius basic pH-Meter PB-20. The purity of the synthesized compounds was determined on an America Agilent 1260 Infinity liquid chromatograph. Mass spectra were carried out on an America Agilent 5975c mass spectrometer. The 1H NMR and 13C NMR spectra were recorded on a Bruker AV 400 spectrometer. UV-vis absorption measurements were measured on a PerkinElmer Lambda 950. PL emission measurements were measured on a PerkinElmer LS55. The absolute photoluminescence quantum yields (ΦPL) were measured with a Hamamatsu absolute PL quantum yield analysis system (Quantaurus-QY, C11347).
Preparation of stock solutions
A stock solution of probe PPN (1.0 mM) was prepared in DMF and diluted with DMF/PBS buffer to the desired concentrations for spectroscopic determination. All the metal ion (K+, Na+, Mg2+, Fe2+, Fe3+, Mn2+, Ca2+, Al3+, Pb2+, Cr2+, Co2+, Zn2+, Bi3+, Ni2+, Pb2+, Sn2+, Ba2+, Ag+, Cu2+) stock solutions (10 μM) were prepared in aqueous solution from their nitrate, chloride or sulfate, and stored at room temperature. All the UV-vis absorption and fluorescence measurements were taken at room temperature.
Synthesis of compound 3-(2′-pyridinecarbonyl)nopinone (PCN)
A solution of nopinone (276 mg, 2 mmol) dissolved in 1,2-dimethoxyethane (6 mL) was added to a suspension of NaH (144 mg, 6 mmol) in 1,2-dimethoxyethane (6 mL). The mixture was refluxed for 0.5 h, whereupon ethyl pyridine-2-carboxylate (453 mg, 3 mmol) dissolved in 1,2-dimethoxyethane (8 mL) was added to the reaction mixture. The reaction was quenched by the addition of EtOH (95%) after 7 h, and extracted with ethyl acetate. After removing solvent, the crude product was purified by column chromatography (100–200 mesh silica gel, eluent: PE/EA, 10/1, v/v) to obtain the yellow grease compound PCN (346.5 mg), yield: 71.3%. 1H NMR (400 MHz, CDCl3) δ: 15.58 (s, 1H), 8.68 (dd, J = 4.9, 1.7 Hz, 1H), 8.00 (d, J = 8.0 Hz, 1H), 7.88–7.72 (m, 1H), 7.35–7.30 (m, 1H), 3.28–2.95 (m, 2H), 2.59 (dt, J = 22.5, 5.4 Hz, 2H), 2.32 (qt, J = 5.9, 2.6 Hz, 1H), 1.49 (d, J = 9.9 Hz, 1H), 1.35 (s, 3H), 0.96 (s, 3H). 13C NMR (100 MHz, CDCl3) δ: 209.55, 169.51, 150.69, 149.01, 135.67, 131.29, 123.24, 104.89, 54.84, 39.77, 39.61, 28.07, 27.66, 25.79, 21.54. HRMS (m/z): [M + H]+ calculated for C15H17NO2 + H+, 244.1299; found, 244.1335.
Synthesis of probe 6,6-dimethyl-2-phenyl-3-(pyridin-2-yl)-4,5,6,7-tetrahydro-2H-5,7-methanoindazole (PPN)
A mixture of compound PCN (486 mg, 2 mmol) and phenyl hydrazine (324 mg, 3 mmol) was added into a round bottom flask with 10 mL methanol, and 10% HAc (1 mL). The reaction mixture was stirred for 5 h at 72 °C. After removal of the solvent, the crude product was purified by column chromatography (100–200 mesh silica gel, eluent: PE/EA, 6/1, v/v) to obtain the yellow grease compound PPN (495.5 mg), yield: 78.4%. 1H NMR (400 MHz, DMSO) δ: 8.57–8.50 (m, 1H), 7.81 (ddt, J = 7.8, 5.6, 1.9 Hz, 1H), 7.38–7.32 (m, 2H), 7.27–7.23 (m, 1H), 7.19–7.15 (m, 4H), 3.51–3.11 (m, 1H), 2.87–2.76 (m, 2H), 2.53–2.50 (m, 1H), 2.29 (dd, J = 5.8, 3.0 Hz, 1H), 1.43–1.34 (m, 4H), 0.74 (d, J = 3.3 Hz, 3H). 13C NMR (100 MHz, CDCl3) δ: 153.67, 150.00, 149.37, 148.36, 139.50, 136.13, 129.08, 127.16, 124.01, 121.86, 120.74, 113.46, 41.79, 41.37, 41.08, 32.28, 27.02, 26.32, 21.69. HRMS (m/z): [M + H]+ calculated for C21H21N3 + H+, 316.1785; found, 316.1811.
Cell imaging using the probe PPN
HeLa cells were incubated with probe PPN (1 μM) in Dulbecco's Modified Eagle's Medium (DMEM) culture medium for 24 h at 37 °C. Then, the cells were divided into two parts. For the control test, cells were washed with phosphate-buffered saline (PBS) three times for the cell images. For the experiment tests, the cells were further incubated with Ag+ (10 μM) for 1 h, then also washed with PBS three times for the cell images. The cell images were obtained via a confocal microscope from Ti-E-A1R (Nikon).
Results and discussion
Synthesis
The synthetic routes of probe PPN are shown in Scheme 1. Compound PCN was synthesized by a Claisen condensation reaction between nopinone and ethyl pyridine-2-carboxylate in the presence of NaH. Finally, compound PPN was obtained by the reaction of compound PCN with phenyl hydrazine in methanol. The structure of the target compounds were characterized by 1H NMR, 13C NMR and HRMS.
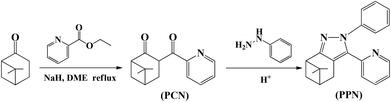 |
| Scheme 1 Synthesis of probe PPN. | |
Colorimetric responses of PPN toward Cu2+
The sensing behaviors of PPN for Cu2+ were studied by UV-vis absorption experiments. UV–vis absorption spectra were carried out in DMF/PBS solution (DMF/PBS buffer, v/v = 5/5, pH = 7.4). As shown in Fig. S1,† upon the addition of metal ions (K+, Na+, Mg2+, Fe2+, Fe3+, Mn2+, Ca2+, Al3+, Pb2+, Cr2+, Co2+, Zn2+, Bi3+, Ni2+, Pb2+, Sn2+, Ba2+, Ag+, Cu2+) to a PPN solution (1.0 μM), only Cu2+ caused an obvious red-shift (from 300 nm to 360 nm), along with a noticeable color change from colorless to yellow. As shown in Fig. 1A, upon the addition of increasing concentrations of Cu2+, the absorption peak at 300 nm decreased gradually and the absorption peak at 360 nm increased regularly, which indicated that a new product between PPN and Cu2+ was formed. The absorption intensity at 360 nm exhibited a good linear increase; when in the Cu2+ concentration range of 10–45 μM, y = 0.0271x − 0.19066 (R2 = 0.99227) (Fig. 1B). The detection limit of probe PPN for Cu2+ was calculated to be 0.56 μM (LOD = 3σ/s). As shown in Fig. 2, other competitive analytes did not interfere with the detection process.
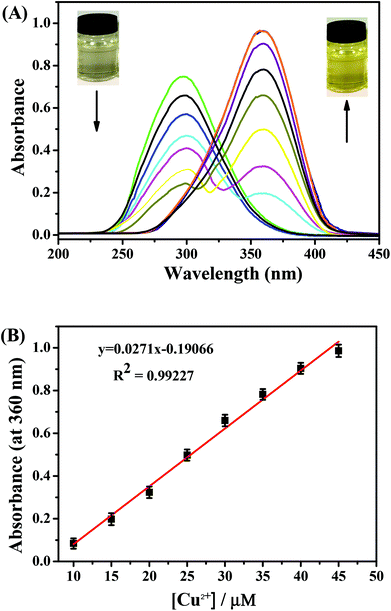 |
| Fig. 1 (A) Absorbance spectra of PPN (10 μM) in the presence of various concentrations of Cu2+ (0–50 μM) in DMF/PBS solution (v/v = 5/5, pH = 7.4). (B) Absorbance intensity (at 360 nm) of PPN against various concentrations of Cu2+. | |
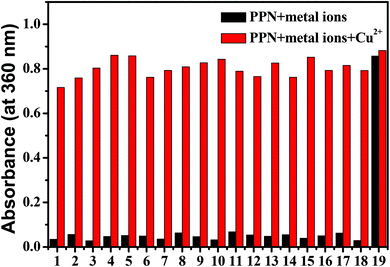 |
| Fig. 2 The interference of other metal ions to probe PPN (10 μM) toward Cu2+ in DMF/PBS solution (v/v = 5/5, pH = 7.4). λex = 340 nm. 1. K+, 2. Na+, 3. Mg2+, 4. Fe2+, 5. Fe3+, 6. Mn2+, 7. Ca2+, 8. Al3+, 9. Pb2+, 10. Cr2+, 11. Co2+, 12. Zn2+, 13. Bi3+, 14. Ni2+, 15. Pb2+, 16. Sn2+, 17. Ba2+, 18. Ag+, 19. Cu2+. | |
As shown in Fig. S2,† after the addition of Cu2+, the absorption intensity of probe PPN remained constant in the pH range of 4.0–10.0. At the same time, with the addition of Cu2+, the absorbance at 360 nm reached the maximum within 60 s. These results indicated that the probe could serve as a colorimetric probe for Cu2+ with a high sensitivity, good selectivity and strong anti-interference ability.
Fluorescence response of PNN toward Ag+
Firstly, we investigated the optimal detection conditions, such as pH range and response time. As shown in Fig. S3,† the fluorescence ratio (F515/F432) reached the maximum after 40 s. As shown in Fig. 3, in the presence of Ag+ (10 μM), the fluorescence ratio (F515/F432) of PPN was enhanced in the range pH 4–10 and reached the maximum at pH = 7. These results indicated that probe PPN can be applied to detect Ag+ in environmental and biological conditions.
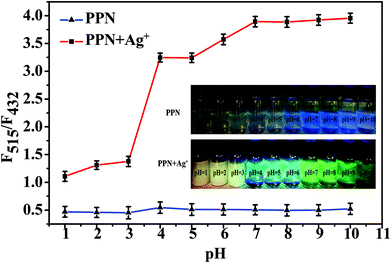 |
| Fig. 3 Fluorescence intensity ratios (F515/F432) of probe PPN (10 μM) in the absence and presence of Ag+ (100 μM) in DMF/PBS solution (v/v = 5/5, pH = 7.4) with different pH values. λex = 340 nm. | |
As shown in Fig. S4,† upon the addition of common metal ions (K+, Na+, Mg2+, Fe2+, Fe3+, Mn2+, Ca2+, Al3+, Pb2+, Cr2+, Co2+, Zn2+, Bi3+, Ni2+, Pb2+, Sn2+, Ba2+, Ag+, Cu2+) to the PPN solution (1.0 μM), only Ag+ caused a noticeable fluorescence color change from blue to green. By contrast, there are no remarkable changes after the addition of other competitive analytes. As shown in Fig. 4, other competitive analytes did not interfere with the detection process.
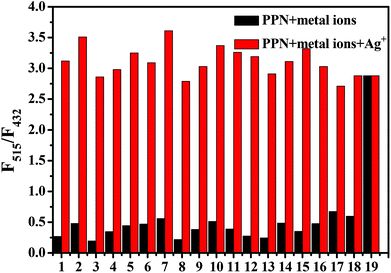 |
| Fig. 4 The interference of other metal ions to probe PPN (10 μM) toward Ag+ in DMF/PBS solution (v/v = 5/5, pH = 7.4). λex = 340 nm. 1. K+, 2. Na+, 3. Mg2+, 4. Fe2+, 5. Fe3+, 6. Mn2+, 7. Ca2+, 8. Al3+, 9. Pb2+, 10. Cr2+, 11. Co2+, 12. Zn2+, 13. Bi3+, 14. Ni2+, 15. Pb2+, 16. Sn2+, 17. Ba2+, 18. Cu2+, 19. Ag+. | |
Next, we studied the sensitivity of probe PPN for Ag+. As shown in Fig. 5, probe PPN displayed a blue fluorescence at 432 nm (Φf = 0.39) in DMF/PBS buffer (v/v = 5/5, pH = 7.4). With the addition of various concentrations of Ag+, an obvious green fluorescence at 515 nm (Φf = 0.43) was observed. The fluorescence lifetime (τ) of probe PPN increased from 2.893 to 3.325 ns after the addition of Ag+. As shown in Fig. 6, the ratio of the fluorescence intensities (F515/F432) was linear with the concentrations of Ag+ in the range 1–80 μM, y = 0.02515x + 0.92678 (R2 = 0.9927). The detection limit of probe PPN for Ag+ was calculated to be 0.86 μM (LOD = 3σ/s).50–53 These results suggested that probe PPN could serve as a ratiometric probe for Ag+ with a high sensitivity, good selectivity and strong anti-interference ability.
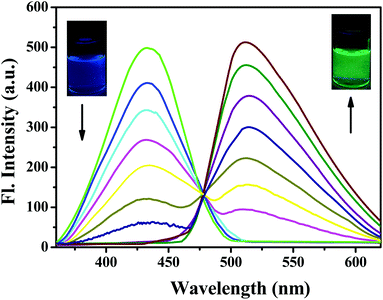 |
| Fig. 5 Fluorescence spectra of PPN (10 μM) in the presence of various concentrations of Ag+ (0–120 μM) in DMF/PBS solution (v/v = 5/5, pH = 7.4). λex = 340 nm. | |
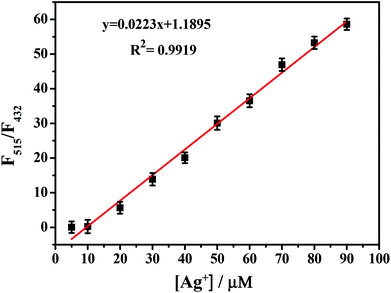 |
| Fig. 6 Fluorescence intensity ratios of PPN (F515/F432) against various concentrations of Ag+. λex = 340 nm. | |
Table S1† summarizes the performance of reported Ag+ fluorescent probes and highlights their applications in water samples and living cells.54–57 These results indicate that PPN can be applied for the detection of Ag+ with a high sensitivity and selectivity.
After continuous illumination for 60 h, the fluorescence intensity of the PPN-Ag+ complex did not show any obvious fluorescence change (Fig. S5†). The good photo-stability of the PPN-Ag+ complex suggests that probe PPN could be used as a novel method for Ag+ detection.
Mechanism study
In order to study the mechanism for the detection of Ag+ and Cu2+, Job plot experiments were first performed. As shown in Fig. S6A,† the ratio of fluorescence intensities (F515/F432) reached the maximum at a molar ratio of about 1
:
1, which manifested a 1
:
1 stoichiometry between Ag+ and PPN. The absorption intensity at 360 nm reached the maximum at a molar ratio of about 0.5, which manifested a 1
:
1 stoichiometry between Cu2+ and PPN (Fig. S6B†). 1H NMR spectroscopy of PPN was performed in DMSO before and after the addition of Ag+ and Cu2+. Compared to the 1H NMR spectrum of the free PPN (Fig. S7†), the proton on the pyridine moiety (H3) shifted downfield and the protons on the phenyl moiety (H1, H2) shifted upfield. The probe PPN results for Ag+ and Cu2+ were further identified by HRMS-ESI experiments; a signal peak at m/z 423.1291 was assigned to [PPN + Ag+ + H]+ (Fig. S8A†). As shown in Fig. S8B,† a new peak at m/z = 451.1091 was consistent with [PPN + Cu2 + 2Cl− + H+]. The proposed coordination mechanism is shown in Scheme 2.
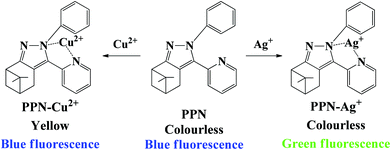 |
| Scheme 2 Proposed coordination mechanism of PPN with Ag+/Cu2+. | |
With the addition of Ag+, the fluorescence emission band at 432 nm red-shifted to 515 nm, which was ascribed to a typical intramolecular charge transfer (ICT) effect. In order to prove the deduction, time-dependent density functional theory (TDDFT) calculations were performed. HOMO–LUMO distributions of PPN and PPN-Ag+ are observed in Fig. S9.† After the addition of Ag+, the lowest occupied molecular orbitals (LUMO) were mainly distributed on pyridine, and the highest occupied molecular orbitals (HOMO) were mainly situated on phenylimidazole. Meanwhile, the band gap energy of PPN-Ag+ (2.667 eV) was smaller than that of PPN (3.948 eV), which proved that the ICT process between the electron-donating and the electron-accepting groups in complex PPN-Ag+ was stronger than those in compound PPN.58–60 The ICT process is due to π–π* transition from pyridine to the phenylimidazole in complex PPN-Ag+, which led to the red-shift in the fluorescence spectra.
Application studies
PPN-based filter papers were prepared by dipping filter papers in PPN solution (10 μM), and drying them in air. As shown in Fig. 7, of the drops on common metal ion solutions on PPN-based filter paper, only Ag+ caused the color the of PPN-based filter paper to change from blue to green under UV light. However, only Cu2+ caused the color change of PPN-based filter paper from colorless to yellow under daylight. Second, PPN-based filter papers were prepared for the detection of the concentration of Cu2+. Different concentrations of Cu2+ solution (0, 2, 6, 10, 40, 80, 100, 120 μM) were dropped on PPN-based filter paper; the color changes of the test strips are shown in Fig. 8. The results demonstrated that the detection limit of PPN for the Cu2+ on the PPN-based filter paper is 6 μM. Therefore, the PPN-based test strips have promising application for the detection of Cu2+/Ag+ in aqueous solution.
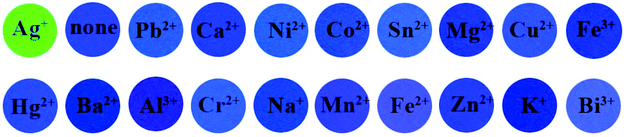 |
| Fig. 7 The images of filter papers containing spots of probe PPN (20 μM, 8 μL) after exposure to other metal ion solutions (100 μM, 8 μL). | |
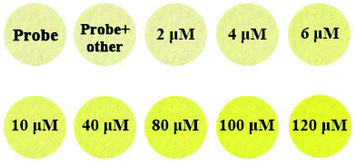 |
| Fig. 8 The images of filter papers containing spots of probe PPN (20 μM, 8 μL) after exposure to various concentrations of Cu2+ (8 μL) (0, 2, 6, 10, 40, 80, 100, 120 μM) under daylight. | |
To evaluate the potential of probe PPN toward Ag+ in water samples, probe PPN was applied to detect the Ag+ concentration in lake water, tap water and distilled water. All the water samples were simply filtered and applied for detection experiments. Different concentrations of Ag+ (1, 5, 10, 15, 20, 30 μM) were added to water samples. As shown in Fig. S10,† there was a linearly relationship between the ratios of fluorescence intensities (F515/F432) and the concentration of Ag+ in different water samples. As shown in Table 1, probe PPN could determine the Ag+ concentration in different water samples with a good recovery.
Table 1 Applicability of PPN for determining Ag+ in different water samples
Samples |
Added (μM) |
Detected (μM) |
Recovery (%) |
Lake water |
1 |
1.02 ± 0.016 |
100.2 |
5 |
4.85 ± 0.035 |
97.5 |
10 |
10.07 ± 0.021 |
100.7 |
15 |
14.92 ± 0.032 |
99.5 |
20 |
20.33 ± 0.019 |
101.2 |
30 |
29.89 ± 0.02 |
99.63 |
Tap water |
1 |
1.05 ± 0.031 |
100.5 |
5 |
4.91 ± 0.012 |
98.2 |
10 |
9.83 ± 0.017 |
98.3 |
15 |
9.49 ± 0.018 |
94.9 |
20 |
20.03 ± 0.013 |
103.1 |
30 |
29.96 ± 0.02 |
99.86 |
Distilled water |
1 |
1.01 ± 0.025 |
100.1 |
5 |
5.13 ± 0.032 |
102.6 |
10 |
9.89 ± 0.016 |
98.9 |
15 |
15.27 ± 0.022 |
100.1 |
20 |
20.03 ± 0.013 |
100.3 |
30 |
29.89 ± 0.013 |
99.6 |
Cell imaging
To further demonstrate the potential of probe PPN in biological samples, cell imaging was evaluated in HeLa cells. The cytotoxicity of probe PPN was firstly measured. The results showed that the cell viability was more than 85%, which indicated that probe PPN had a low cytotoxicity (Fig. S11†). HeLa cells were incubated with only probe PPN (1 μM) for 24 h at 37 °C, and exhibited a blue fluorescence (Fig. 9). However, HeLa cells were incubated with probe PPN for 24 h and then incubated with Ag+ for 1 h, and a bright green fluorescence was observed. These results demonstrated that probe PPN could track Ag+ in living cells.
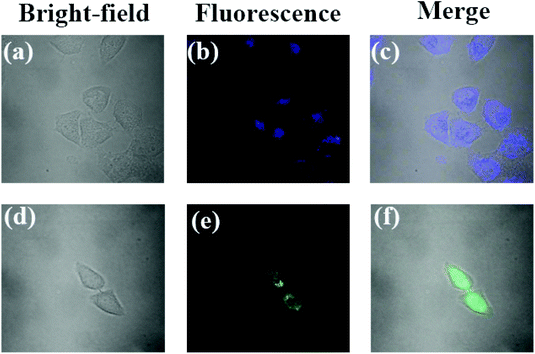 |
| Fig. 9 Fluorescent images of HeLa cells. Top row: Cells incubated with probe PPN (10 μM) for 24 h at 37 °C. Bottom row: The cells are treated with AgNO3 (100 μM) for 60 min after incubation with probe PPN. | |
Conclusions
In conclusion, a novel probe PPN based on the natural β-pinene derivative nopinone for the colorimetric detection of Cu2+ and ratiometric detection of Ag+ was designed and synthesized. Not only could it be used as a ratiometric fluorescent probe for Ag+ by the significant change of fluorescent color, but also it could act as a colorimetric probe for Cu2+ by the change of solution color. The probe PPN could determine the concentration of Ag+/Cu2+ with a high selectivity, low detection limit (for Ag+, LOD = 0.86 μM; for Cu2+, LOD = 0.56 μM) and fast response. A 1
:
1 stoichiometry between PPN and Ag+/Cu2+ was proved by Job-plot analysis, 1H NMR spectra and HRMS-ESI spectra. Moreover, PPN-based filter papers were prepared for the detection of Ag+/Cu2+. In addition, probe PPN was employed for visualizing Ag+ in water samples and living cells.
Conflicts of interest
There are no conflicts to declare.
Acknowledgements
The research was supported by the National Natural Science Foundation of China (no. 31470592), Jiangsu Provincial Key Lab for the Chemistry and Utilization of Agro-Forest Biomass, the open fund of Jiangsu Key Laboratory for Biomass Energy and Material (JSBEM201702), a Priority Academic Program Development of Jiangsu Higher Education Institutions (PAPD), and a project funded by the National First-Class Disciplines (PNFD).
Notes and references
- X. C. Yin, J. Long, Y. Xi and X. B. Luo, ACS Sustainable Chem. Eng., 2017, 5, 2090–2097 CrossRef CAS.
- J. L. Barriada, A. D. Tappin and E. H. Evans, TrAC, Trends Anal. Chem., 2007, 26, 809–817 CrossRef CAS.
- J. Liu, W. Qu and M. B. Kadiiska, Toxicol. Appl. Pharmacol., 2009, 238, 209–214 CrossRef CAS PubMed.
- Z. W. Dong, L. Wang, J. P. Xu, Y. L. Li, Y. Zhang, S. L. Zhang and J. Y. Miao, Toxicol. In Vitro, 2009, 23, 105–110 CrossRef CAS PubMed.
- I. Takashima, A. Kanegae and M. Sugimoto, Inorg. Chem., 2014, 53, 7080–7082 CrossRef CAS.
- S. Singha, D. Kim, H. Seo, S. W. Cho and K. H. Ahn, Chem. Soc. Rev., 2015, 44, 4367–4399 RSC.
- L. J. Bian, X. Ji and W. Hu, J. Agric. Food Chem., 2014, 62, 4870–4877 CrossRef CAS.
- Y. H. Zhao, Y. Y. Luo, H. Wang, L. Yuan and X. B. Zhang, Anal. Chim. Acta, 2019, 1065, 134–141 CrossRef CAS PubMed.
- R. L. Chao, Y. Y. Zheng and L. L. Si, J. Lumin., 2018, 198, 327–336 CrossRef.
- H. Z. Ni, Q. M. Wang, L. Jin, W. L. Wang, L. H. Dai and C. Zhao, J. Lumin., 2019, 206, 125–131 CrossRef CAS.
- Z. Y. Zhang, Y. P. Liu and E. J. Wang, Dyes Pigm., 2019, 163, 533–537 CrossRef CAS.
- Q. M. Wang, S. S. Wu, Y. Z. Tan, Y. L. Yan and X. H. Tang, J. Photochem. Photobiol., A, 2018, 357, 149–155 CrossRef CAS.
- Y. S. Yang, S. S. Ma, Y. P. Zhang, J. X. Ru, X. Y. Liu and H. C. Guo, Spectrochim. Acta, Part A, 2018, 199, 202–208 CrossRef CAS PubMed.
- Y. Fu, X. X. Pang, Z. Q. Wang, Q. Chai and F. Ye, Spectrochim. Acta, Part A, 2019, 208, 198–205 CrossRef CAS PubMed.
- L. L. Wang, Y. Qu, Y. X. Yang, J. Cao and L. Wang, Sens. Actuators, B, 2018, 269, 70–78 CrossRef CAS.
- M. C. Yeung and V. W. Yam, Chem. Soc. Rev., 2015, 44, 4192–4202 RSC.
- C. J. Chen, A. J. Zhang, J. B. Hu, Y. H. Miao and X. Liu, Inorg. Chim. Acta, 2015, 435, 137–141 CrossRef CAS.
- B. Daly, J. Ling and A. P. Silva, Chem. Soc. Rev., 2015, 44, 4203–4211 RSC.
- P. S. Yao, Z. Liu, J. Z. Ge, Y. Chen and Q. Y. Cao, Dalton Trans., 2015, 44, 7470–7476 RSC.
- R. D. Hancock, Chem. Soc. Rev., 2013, 42, 1500–1524 RSC.
- Q. Q. Tan, W. S. Kong and F. L. Qu, Microchim. Acta, 2019, 186, 282–291 CrossRef PubMed.
- Q. Q. Tan, R. R. Zhang and F. L. Qu, ACS Appl. Bio Mater., 2018, 1, 777–782 CrossRef CAS.
- F. L. Qu, H. M. Pei and L. Xia, Talanta, 2017, 165, 136–142 CrossRef CAS PubMed.
- K. P. Carter, A. M. Young and A. E. Palmer, Chem. Rev., 2014, 114, 4564–4601 CrossRef CAS PubMed.
- L. L. Wang, Y. Qu, Y. X. Yang, J. Cao and L. Wang, Sens. Actuators, B, 2018, 269, 70–78 CrossRef CAS.
- K. S. Wang, K. Y. Park, D. B. Kim and S. K. Chang, Dyes Pigm., 2017, 147, 413–419 CrossRef.
- X. Yang, E. Wang and A. Chen, Anal. Chem., 2011, 83, 5005–5011 CrossRef CAS PubMed.
- Y. T. Wu, J. L. Zhao, L. Mu, X. Zeng, G. Wei, C. Redshaw and Z. W. Jin, Sens. Actuators, B, 2017, 252, 1089–1097 CrossRef CAS.
- A. Coskun and E. U. Akkaya, J. Am. Chem. Soc., 2005, 127, 10464–10465 CrossRef CAS PubMed.
- C. R. Li, Z. Y. Yang and S. L. Li, J. Lumin., 2018, 198, 327–336 CrossRef CAS.
- X. M. Wu, H. Wang, S. X. Yang, H. Y. Tian and B. G. Sun, Food Chem., 2019, 284, 23–27 CrossRef CAS PubMed.
- G. Y. Duan, G. X. Zhang, S. Q. Yuan and Y. Q. Ge, Spectrochim. Acta, Part A, 2019, 219, 173–178 CrossRef CAS PubMed.
- H. L. Mu, R. Gong, L. Ren, C. Zhong and E. Q. Fu, Spectrochim. Acta, Part A, 2008, 70, 923–928 CrossRef PubMed.
- B. H. Kang, Z. F. Gao, N. Li, Y. Shi, N. B. Li and H. Q. Luo, Talanta, 2016, 156, 141–146 CrossRef.
- Y. Fu, L. Mu, X. Zeng, J. L. Zhao, X. L. Ni and T. Yamato, Dalton Trans., 2013, 42, 3552–3560 RSC.
- Y. L. Zhang, W. H. Chen, X. T. Dong, X. H. Wang and L. J. Bian, Sens. Actuators, B, 2018, 261, 58–65 CrossRef CAS.
- H. J. Wu, J. H. Jia, Y. F. Xu, X. H. Qian and W. P. Zhu, Sens. Actuators, B, 2018, 265, 59–66 CrossRef CAS.
- G. N. Lin, H. Xu, Y. J. Cui, Z. Y. Wang, Y. Yang and G. D. Qian, Mater. Chem. Phys., 2013, 141, 591–595 CrossRef CAS.
- Y. Huang, C. F. Li, W. J. Shi, F. G. Liu and J. W. Yan, Talanta, 2019, 198, 390–397 CrossRef CAS.
- Y. Feng, Y. Yang, Y. Z. Wang, F. Z. Qiu, G. L. Zhang and W. S. Liu, Sens. Actuators, B, 2019, 288, 27–37 CrossRef CAS.
- C. C. Wang, C. H. Yang, S. M. Tseng, Y. M. Cheng and P. T. Chou, Inorg. Chem., 2004, 43, 4781–4783 CrossRef CAS PubMed.
- H. L. Liu, S. Q. Cui, F. Shi and S. Z. Pu, Dyes Pigm., 2019, 161, 34–43 CrossRef CAS.
- S. Erdemir and S. Malkondu, Dyes Pigm., 2019, 163, 330–336 CrossRef CAS.
- R. Flamholc, A. Wrona-Piotrowicz, A. Makal and J. Zakrzewski, Dyes Pigm., 2018, 154, 52–61 CrossRef CAS.
- L. Liu, Y. Le, M. G. Teng, Z. X. Zhou, C. S. Zhao and J. X. Cao, Dyes Pigm., 2018, 151, 1–6 CrossRef CAS.
- S. Swami, A. Agarwala, D. Behera and R. Shrivastava, Sens. Actuators, B, 2018, 260, 1012–1017 CrossRef CAS.
- J. L. Yang, X. Xu, J. Rui and S. F. Wang, Spectrochim. Acta, Part A, 2017, 183, 60–67 CrossRef CAS PubMed.
- Z. L. Wang, Y. Zhang, J. Song, H. J. Xu and S. F. Wang, Anal. Chim. Acta, 2019, 65, 23–29 Search PubMed.
- J. L. Yang, H. J. Xu, X. Xu, X. Q. Cao and S. F. Wang, Dyes Pigm., 2016, 128, 75–83 CrossRef CAS.
- H. Liang, Z. G. Li, D. Q. Wu, M. H. Yan and X. G. Tuo, Sens. Actuators, B, 2018, 269, 62–69 CrossRef CAS.
- L. Kang, Y. T. Liu, N. N. Li, J. L. Li and Y. Zhang, J. Lumin., 2017, 186, 48–52 CrossRef CAS.
- N. Zhang, X. H. Tian, J. Zheng, Q. Y. Zhu and H. P. Zhou, Dyes Pigm., 2016, 124, 174–179 CrossRef CAS.
- M. G. Choi, J. L. Yu, I. J. Chang, S. W. Yoon and S. K. Chang, Sens. Actuators, B, 2018, 268, 22–28 CrossRef CAS.
- N. Bhuvanesh, S. Suresh, J. Prabhu, K. Kannan, V. Rajesh Kannan and R. Nandhakumar, Opt. Mater., 2018, 82, 123–129 CrossRef CAS.
- Y. Zhang, D. H. Wang, C. L. Sun, D. Z. Zhao and Y. F. Bi, Dyes Pigm., 2017, 141, 202–208 CrossRef CAS.
- Y. Li, H. Yu and G. Shao, J. Photochem. Photobiol., A, 2015, 301, 14–19 CrossRef CAS.
- H. Wu, J. Jia and Y. Xu, Sens. Actuators, B, 2018, 241, 59–66 CrossRef.
- T. T. Zhang, X. P. Chen and J. T. Liu, RSC Adv., 2014, 4, 16973–16978 RSC.
- Y. Wang, L. J. Wang and E. Jiang, Dyes Pigm., 2018, 156, 338–347 CrossRef CAS.
- T. G. Jo, K. H. Bok and J. Han, Dyes Pigm., 2017, 139, 136–147 CrossRef CAS.
Footnote |
† Electronic supplementary information (ESI) available. See DOI: 10.1039/c9pp00297a |
|
This journal is © The Royal Society of Chemistry and Owner Societies 2020 |