DOI:
10.1039/C8NP00027A
(Highlight)
Nat. Prod. Rep., 2020,
37, 6-16
Harnessing diverse transcriptional regulators for natural product discovery in fungi
Received
27th March 2018
First published on 29th April 2019
Abstract
Covering: Up to March 2019
Secondary metabolites (SMs) are chemical entities produced by organisms in response to environmental stimuli and as a defense against biological warfare. The production of SMs is controlled by a hierarchical regulatory network involving core factors that orchestrate transcriptional activation of SM gene clusters. In the past few years, significant achievements have been made in the discovery of novel fungal natural products by genetic manipulations of various types of transcriptional regulators. In this review, we summarized the representative regulators for the activation of fungal secondary metabolism and focused on the strategies for the exploitation of these regulators and their application in finding novel structures.
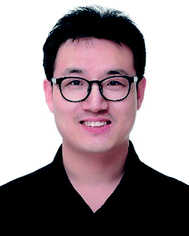 Hai-Ning Lyu | Hai-Ning Lyu obtained his Bachelor's degree (2011) in Pharmaceutical Engineering from Beijing University of Chinese Medicine. He received his Master's (2014) and PhD degree (2017) in Natural Medicine at Peking University, under the supervision of Prof. Peng-Fei Tu and Prof. Yong Jiang. His doctoral research focused on the discovery of bioactive natural products from plants of the genera Murraya and Sinomenium. Currently, he works as a staff scientist in the Institute of Microbiology, Chinese Academy of Sciences, with Professor Wen-Bing Yin, where his research interests include the discovery, biosynthesis and metabolic engineering of natural products. |
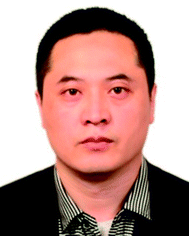 Hong-Wei Liu | Hong-Wei Liu is currently a Professor in the Institute of Microbiology, Chinese Academy of Sciences (CAS), Beijing, China. He obtained his PhD in medicinal chemistry in 2003 from Shenyang Pharmaceutical University with Professor Xin-Sheng Yao where he investigated the bioactive natural products from traditional Chinese medicine. Then he worked with Professor Guo-Ping Cai as a post-doctoral fellow at the Tsinghua University to study marine natural products. After that, he moved to Capital Medical University where he worked as an associated professor in the School of Pharmaceutical Sciences. In 2009, he joined the Institute of Microbiology and led a research team in the field of fungal natural products. His research interests mostly focus on the identification of novel bioactive fungal secondary metabolites, elucidation of biosynthetic pathway of fungal products, and generation of bioactive pharmaceutical agents by synthetic biology methods. |
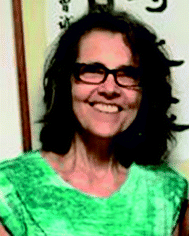 Nancy P. Keller | Nancy Keller obtained her PhD degree in the Plant Pathology Department at Cornell University and is currently a Professor in the Department of Medical Microbiology and Immunology and Bacteriology at the University of Wisconsin. For her entire professional career, she has explored the genetics underlying fungal development with an emphasis on natural product synthesis in fungi. |
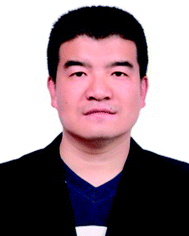 Wen-Bing Yin | Wen-Bing Yin is currently a Professor in the Institute of Microbiology, Chinese Academy of Sciences (CAS), Beijing, China. He obtained his PhD in Pharmaceutical Biology in 2009 from Philipps-University Marburg with Professor Shu-Ming Li where he studied the chemo-enzymatic biosynthesis of fungal natural products. Then he worked with Professor Nancy Keller as a post-doctoral fellow at the University of Wisconsin–Madison to study gene regulation on fungal secondary metabolism. After that, he moved to Los Angeles and worked with Professor Yi Tang at the University of California where he studied the discovery of natural products by a synthetic biological approach. In 2014, he returned to China to join the Institute of Microbiology and was supported by “the 100 Talents Project” of CAS. His research interests mostly focus on the activation, biosynthesis of silent fungal biosynthetic gene cluster and synthetic biological reconstruction of pharmaceutical molecules. |
1. Introduction
Secondary metabolites (SMs), usually called natural products (NPs), play a highly significant role in the discovery of drug leads for the treatment of human diseases. For example, more than half of the clinically approved medicines are NPs or their derivatives.1 Despite the significant advances made via the study of natural resources, industrial investments in NP research have been reduced as traditional NP discovery methods (growth of microbes on different media) cannot meet the ever-growing demands for new bioactive molecules in drug research and development; therefore, the development of innovative approaches for accurate targeting and activation of undiscovered NPs is urgent for drug discovery.
Filamentous fungi are well known for their abilities to produce an enormous amount of SMs, many of which, including antibiotics, cholesterol-lowering drugs, immunosuppressants, and anti-osteoporosis agents, have benefitted human health.2,3 Recent advances made in sequencing technologies and bioinformatics analysis have revealed a large amount of fungal SM encoding genes, which are usually clustered in a chromosome and called biosynthetic gene clusters (BGCs).4–6 However, to date, only a few BGCs have been functionally annotated and correctly assigned to the relative metabolites;4–9 one of the major reasons hindering the identification of BGCs is the poor understanding of how these BGCs are regulated. Most BGCs are not expressed or only lowly expressed under laboratory culture conditions; this suggests that a huge fungal NP treasure trove is yet to be exploited. Indeed, it has been estimated that several million fungal BGCs are yet to be characterized.10
How to activate these BGCs in the laboratory? In recent years, genome mining strategies, including transcriptional regulator modulation, promoter engineering, as well as heterologous expression, have been successfully developed and introduced to awake the silent/lowly expressed BGCs for new fungal NP discovery.10,11 Among them, genetic manipulations of regulators have made significant progress, ranging from overexpression of pathway-specific regulators to deletion of epigenetic blocks of BGC expression to gain access to new fungal NPs. In this review, we presented the recent achievements made in mining novel NPs in fungi via genetic manipulations of various types of regulators. The inherent advantages and disadvantages of these approaches have also been discussed. Moreover, a promising combinational strategy for the discovery of novel fungal SMs has been outlined in Section 4.
2. Transcriptional regulators play critical roles in fungal secondary metabolism
Fungi produce SMs to adapt to variable environmental stresses, and thus, the production of SMs is controlled by complex regulatory networks. The current understanding of fungal secondary metabolism regulation has been reported in many excellent reviews,7–9,11–14 indicating that regulators are central players in the fungal secondary metabolism. In this section, we present the critical roles of regulators involved in SM biosynthesis by outlining the fungal SM regulatory network at different levels including pathway-specific regulation, global regulation, and epigenetic regulation.
More than half of the fungal BGCs contain a putative regulatory gene.8 These transcription factors act as pathway-specific regulators that connect the fungal BGCs to one specific metabolite (as shown in Scheme 1). The most common type of these regulators is the Zn(II)2Cys6 domain protein that has to date only been found in fungi. The archetype is undoubtedly AflR, which binds to the palindromic sequence 5′-TCG(N5)CGA-3′ in the promoter regions of several aflatoxin and sterigmatocystin cluster genes in Aspergillus.15 In addition to Zn(II)2Cys6 proteins, Cys2His2 zinc-finger proteins are commonly found in fungi such as Tri6 for the regulation of mycotoxin deoxynivalenol (DON) with Tri10 by cAMP signalling in F. graminearum.16 The less frequently found regulators in fungi are ankyrin repeat proteins (ToxE for HC-toxin production)17 and winged helix proteins (CPCR1 for cephalosporin C production).18 However, all these transcription factors were first identified in laboratory-expressed BGCs; moreover, they were also present in laboratory-silent BGCs and successfully used to activate new metabolites, as has been described in the next section.
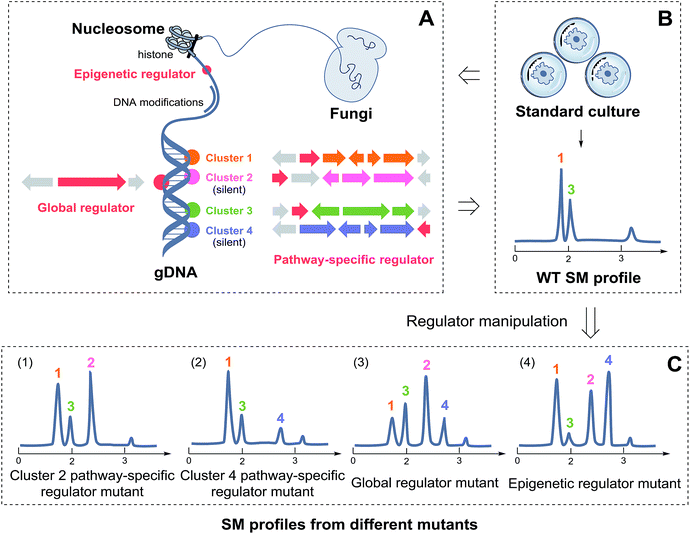 |
| Scheme 1 The strategy for fungal NP discovery via genetic manipulation of regulators. (A) Shows the possible locations of three types of regulators (pathway-specific regulator, global regulator, and epigenetic regulator) within the fungal genome, marked in red. (B) Shows the generation of SMs 1 and 3 under standard culture condition for demonstration. (C) Displays four hypothetical SM profiles of mutants. Manipulations of pathway-specific regulators led to the production of the corresponding SMs 2 and 4. Manipulations of global and epigenetic regulators could change the whole SM metabolic profiling, which activated the silent SMs 2 and 4 randomly. | |
Pathway-specific regulation delineates the transcription of single BGCs in quite a direct pattern. However, fungal SM biosynthesis is generally affected by various environmental factors including pH, temperature, nutrition supply, interspecific interactions, and other unknown factors. Global regulators often relay these environmental signals through higher-level regulation of the fungal secondary metabolism (as shown in Scheme 1). For example, aflatoxin and sterigmatocystin production is decreased with the increasing environmental pH.19 The overexpression of pacC, a global regulator for pH regulation in fungi, could mimic alkaline conditions for reducing the productions of aflatoxin and sterigmatocystin in A. nidulans.19 PacC acts as a key transcription mediator in the pH regulation of various BGCs via repressing the transcription of acid-expressed genes. To date, a number of global regulators, such as CreA (carbon catabolite regulation and regulation of patulin biosynthesis in Penicillium expansum),20,21 AreA (nitrogen regulation and regulation of up to 1/2 of the BGCs in Fusarium fujikuroi),22,23 and the HAP-like CCAAT-binding complex regulating penicillin production and iron supply,24,25 responding to environmental signals and involved in the regulations of specific BGCs have been identified. Genetic manipulation of these regulators influences the production of SMs that are susceptible to the corresponding environmental stimuli. However, note that global regulators usually participate in the huge and complex regulatory network of fungal development; thus, genetic operations on these types of regulators may cause abnormal growth and/or dramatic changes in the metabolomic profile of fungi.
Although the co-regulation of SM biosynthesis by pathway-specific and global regulators depicts a simple multiple level model system at first glance, fungal SM regulation does not follow a strict hierarchical regime in most cases. For example, the discovery of LaeA—a global regulator positively regulating up to half of the BGCs in filamentous fungi26–29—has led to the finding that SMs are intimately connected to fungal development. A seminal study reported in 2008 demonstrated that LaeA partnered with two other proteins to form the ‘Velvet Complex’ composed of VelB, VeA, and LaeA, coordinating fungal development and secondary metabolism in response to light.30 Unlike the other global regulators that are bonafide transcription factors, LaeA is a nuclear protein with the closest identity to arginine and histone methyltransferases; this suggests that LaeA may affect the SM production via epigenetic modification of the chromatin. This hypothesis was further supported by comparison of the heterochromatic marks of the sterigmatocystin BGC in the wild-type (high sterigmatocystin) versus the ΔlaeA mutant (low sterigmatocystin) in A. nidulans; this revealed increased repressive histone 3 lysine 9 (H3K9) trimethylation in the ΔlaeA mutant.31 Therefore, the discovery of LaeA demonstrated a chromatin-mediated mechanism for secondary metabolism regulation and opened a new strategy by epigenetic manipulation of chromatin modifying proteins to genetically control the expressions of fungal BGCs.
3. Regulators as tools to shed light on the new fungal chemistry
Among the regulators abovementioned in Section 2, the most useful tools for fungal NP discovery are pathway-specific regulators and chromatin modifying proteins. To date, a number of silent fungal SMs, including both truly new compounds and known metabolites (which have been isolated from other fungi before), have been obtained upon BGC activation. The structures of the truly new compounds are shown in Fig. 1, and their regulators-in-charge are listed in Table 1.
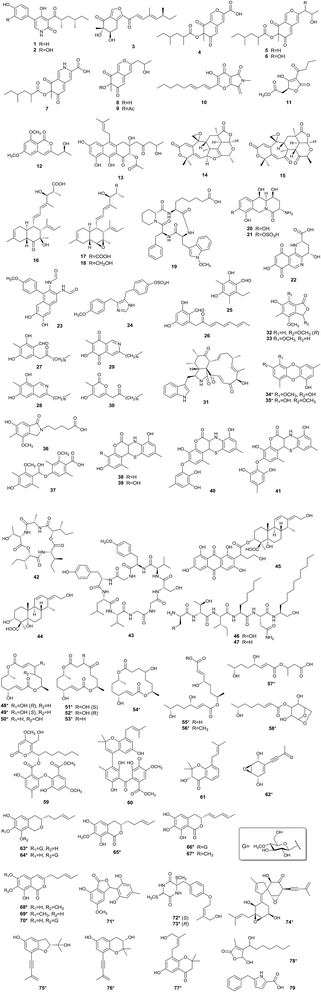 |
| Fig. 1 Novel fungal NPs produced by genetic manipulation of pathway-specific (1–30), global (31–37), and epigenetic (38–79) regulators. *: BGC not identified. | |
Table 1 Regulators used for discovering new fungal NPs
Regulatorsa |
Accession no. |
Domain/description |
New metabolites discovered |
Species |
References |
Alphabetically arranged.
Underdetermined.
BGC not identified.
|
Pathway-specific regulators
|
AfoA |
Q5BEK1 |
Zn(II)2Cys6 |
Asperfuranone 3 |
Aspergillus nidulans
|
33
|
AoiH |
BAE65966 |
Zn(II)2Cys6 |
12
|
Aspergillus oryzae
|
37
|
ApdR |
Q5ATG6 |
Zn(II)2Cys6 |
Aspyridones A 1 and B 2 |
Aspergillus nidulans
|
32
|
APS2 |
S0EBQ6 |
Zn(II)2Cys6 |
Apicidin F 19 |
Fusarium fujikuroi
|
41
|
AzaR |
G3XMC5 |
Zn(II)2Cys6 |
Azanigerones A–F 4–9 |
Aspergillus niger
|
34
|
BerA |
—b |
Zn(II)2Cys6 |
Berkeleyacetal D 14, 11-epiberkeleyacetal C 15 |
Neosartorya glabra
|
39
|
CaaR |
CAK45414 |
Zn(II)2Cys6 |
Carlosic acid methyl ether 11 |
Aspergillus niger
|
36
|
FSL7 |
ESU17175 |
Zn(II)2Cys6 |
Fusarielins F–H 16–18 |
Fusarium graminearum
|
40
|
NscR |
A1D8J1 |
Zn(II)2Cys6 |
Neosartoricin 13 |
Aspergillus fumigatus
|
38
|
PynR |
CAK48262 |
Zn(II)2Cys6 |
Pyranonigrin E 10 |
Aspergillus niger
|
35
|
FsqA |
XP_747734 |
Zn(II)2Cys6 |
Fumisoquins A–C 20–22 |
Aspergillus fumigatus
|
42
|
XanC |
A4DA05 |
bZIP |
Melanocins E 23 and F 24 |
Aspergillus fumigatus
|
43
|
— |
EAA66632 |
Zn(II)2Cys6 |
25
|
Aspergillus nidulans
|
44
|
— |
EAA64857 |
Zn(II)2Cys6 |
26
|
Aspergillus nidulans
|
44
|
— |
EAA63353 |
Zn(II)2Cys6 |
27–30
|
Aspergillus nidulans
|
44
|
![[thin space (1/6-em)]](https://www.rsc.org/images/entities/char_2009.gif) |
Global regulators
|
LaeA |
EAQ93455 |
— |
Chaetoglobosin Z 31 |
Chaetomium globosum
|
55
|
LaeB |
EAA60741 |
— |
3-Methoxyporriolide 32, 7-methoxyporriolide 33, gibellulin C 34c and D 35c |
Aspergillus nidulans
|
56
|
McrA |
EAA60243 |
Zn(II)2Cys6 |
36, 37 |
Aspergillus nidulans
|
52
|
![[thin space (1/6-em)]](https://www.rsc.org/images/entities/char_2009.gif) |
Epigenetic regulators
|
Hat1 |
EFZ02700 |
Histone acetyltransferase |
Meromusides A–I 63–71c, meromutides A 72c and B 73c |
Metarhizium robertsii
|
72
|
HdaA |
— |
Histone deacetylase |
Arbumycin 42, arbumelin 43, arbusculic acids A 44 and B 45 |
Calcarisporium arbuscular
|
70
|
NnaB |
CBF84101 |
GNAT-type acetyltransferase |
Pheofungins A–D 38–41 |
Aspergillus nidulans
|
61
|
PfcclA |
ETS83251 |
Histone methyltransferase |
Pestaloficiols T–V 59–61c |
Pestalotiopsis fici
|
71
|
PfhdaA |
ETS79135 |
Histone deacetylase |
Ficiolides A–K 48–58c, pestaloficiol W 62c |
Pestalotiopsis fici
|
71
|
RpdA |
EAA60836 |
Histone deacetylase |
Aspercryptins A1 46 and A2 47 |
Aspergillus nidulans
|
65
|
![[thin space (1/6-em)]](https://www.rsc.org/images/entities/char_2009.gif) |
Other regulators
|
PfcsnE |
ETS75687 |
COP9 signalosome |
Pestaloficins A–E 74–78c |
Pestalotiopsis fici
|
75
|
PkaC1 |
CAC82611 |
Protein kinase A |
Fumipyrrole 79 |
Aspergillus fumigatus
|
76
|
3.1 Pathway-specific transcription factors activate the dedicated BGCs
Manipulation of the pathway-specific regulators is one of the most convenient and successful approaches to discover novel NPs. These transcription factors are encoded within the BGC they regulate and thus easily recognized bioinformatically. Several cryptic BGCs have been activated, and new metabolites have been identified simply by overexpressing the BGC regulator. For example, the novel pyridone alkaloids aspyridones A and B (1 and 2) were identified when the in-cluster putative pathway-specific regulator apdR (a Zn(II)2Cys6 protein) was overexpressed and activated the entire apd BGC in A. nidulans (Fig. 1).32 In the same fungus, the overexpression of the transcriptional regulatory gene afoA in a BGC including two PKS genes resulted in the production of a new polyketide, asperfuranone 3 (Fig. 1).33 In A. niger, the overexpression of azaR, a Zn(II)2Cys6 zinc finger protein within a dual PKS gene cluster, afforded six novel azaphilone compounds, azanigerones A–F (4–9) (Fig. 1).34 Similarly, by applying the same method, two PKS/NRPS hybrid metabolites (pyranonigrin E 10 and carlosic acid methyl ether 11) (Fig. 1) were characterized in the same species.35,36 To date, ca. 30 new compounds have been discovered via overexpression of pathway-specific regulators. These include polyketide 12 from A. oryzae,37 anthracenone 13 from A. fumigatus,38 the berkeleyacetal derivatives 14 and 15 from Neosartorya glabra,39 cytotoxic fusarielins 16–18 from F. graminearum,40 apicidin congener 19 from F. fujikuroi,41 fumisoquins A–C 20–22 from A. fumigatus,42 the melanocins E 23 and F 24 from A. fumigatus,43 and the polyketides 25–30 from A. nidulans44 (Fig. 1).
Indeed, the manipulation of pathway-specific regulators is a straightforward method to activate a specific BGC in fungi and offers unique advantages by accurately targeting novel structures even from chemically well-studied species. However, this approach has limitations if a BGC doesn't possess a pathway-specific regulator or does not activate for some unknown reason. In these cases, combinational methods with other genetic manipulation techniques can be used to activate a BGC. For example, replacement of the promoters of BGC genes led to the identifications of eight polyketide compounds, including the novel structures 25–30 in A. nidulans.44 An exciting advance was achieved with the activation of the asperlin BGC in A. nidulans through fusion of the DNA-binding domain of the silent asperlin cluster with the asperfuranone transcription factor AfoA.45
3.2 Global regulators can enhance the overall fungal NP diversity
Genetic mutagenesis has been successful in identifying several global regulators of fungal secondary metabolism. For instance, laeA and laeB were identified from A. nidulans by examining 23 mutants that displayed a phenotype of loss of sterigmatocystin production.26,46McrA was disclosed through screening the mutants that up-regulated the transcription of the BGC for nidulanin A biosynthesis. In general, the global regulators are conserved across fungal genera. By identifying the sequence of each protein, such as LaeA,26,47 LaeB,46 CreA,20 AreA,22 PacC,48 MeaB,49 HapX,50 RsmA,51 and McrA,52 we can find a homolog in most ascomycetes and thus use that gene to up-regulate the BGCs in the selected fungi. In particular, LaeA has been identified and manipulated in dozens of fungal species; this has led to the studies of a magnitude of BGCs.53
Basically, the manipulation of global regulators for new NP discovery could be regarded as a “genetic” form of the OSMAC54 (One Strain Many Compounds) approach. Note that the studies concerning global regulators mainly focus on their influences on fungal development or the production changes of known SMs such as the stimulation of antibiotic yield,7,8 rather than the discovery of new NPs. However, some studies have showed new metabolites using these proteins. For example, the overexpression of laeA up-regulated the expression of the chaetoglobosin gene cluster in Chaetomium globosum and led to the isolation of a new structure, chaetoglobosin Z 31, together with six known analogues, chaetoglobosins A, B, D, E, O, and V.55 Recently, eight silent SMs have been activated and obtained from A. nidulans by disruption of LaeB.56 Among the isolates, two phthalides (3-methoxyporriolide 32 and 7-methoxyporriolide 33) and two dibenzo[1,4]dioxins (gibellulin C 34 and D 35) possessed new structures. This was the first study on dibenzo[1,4]dioxin derivatives obtained from an Aspergillus species, enhancing the chemical diversity of the genus. Deletion of the global regulator mcrA in A. nidulans led to the dramatic upregulation of nidulanin derivatives and prenyl xanthones, along with two new cichorine analogues 36 and 37 (Fig. 1).52
3.3 Epigenetic regulators in the fungal natural product discovery
Similar to the case of global regulators, the genetic manipulation of epigenetic regulators can activate a multitude of BGCs that are often laboratory-silent BGCs. The reader is referred to a recent review for extensive details on this approach.57 The success of this method is due to chromatin remodelling arising from the deletion or overexpression of histone modifying genes that lead to the activation of the BGCs located in the heterochromatic regions on the chromatin. For example, Bre2 is a critical member of the COMPASS complex, which is a conserved eukaryotic transcriptional effector bidirectionally regulating chromatin-mediated processes through methylation of H3K4.58 Deletion of cclA in A. nidulans, the ortholog of bre2, activates the production of emodin analogues as well as two polyketides F9775A and B, whose encoding BGCs are located at the right telomere of chromosome VIII and the left telomere of chromosome II, respectively.2
By knowing that histone proteins function as a scaffold for nucleosome formation, modifications of histone decorations, including methylation, acetylation, phosphorylation, ubiquitylation, and sumoylation,59 have been proven to be dependable approaches for remodelling of the chromatin landscape and, in the case of fungi, activating BGCs.57 The most common strategy is to delete or overexpress histone methyltransferases, acetylases, demethylases or deacetylases including GcnE,60 NnaB,61 EsaA,62 HdaA,63 SirA,64 RpdA,65 HosA,66 HstD,67 KdmA,68 and RmtA.69 Some of these manipulations have been successfully employed to access fungal chemical diversity and new NP discovery. Deletion of the putative GNAT-type acetyltransferase NnaB in A. nidulans resulted in a slow-growing, yellow to red/orange pigmented strain that resulted in a significantly increased production of SMs. HPLC-HRESIMS analysis of the ΔnnaB strain yielded a series of heterocyclic products, which were further identified as pheofungins A–D (38–41) (Fig. 1).61 Inactivation of the histone deacetylase hdaA resulted in aberrant growth and physiology in Calcarisporium arbuscular as well as production of novel metabolites, two new peptides (arbumycin 42 and arbumelin 43) and two new diterpenes (arbusculic acids A 44 and B 45) (Fig. 1).70 Similarly, down regulation of the histone deacetylase rpdA in A. nidulans led to the selective up-regulation of two new lipopeptides, aspercryptin A1 46 and A2 47 (Fig. 1).65 Chemical analysis of the HdaA and CclA deletion mutants in P. fici revealed polyketide productions of 11 macrodiolide ficiolides A–K (48–58) as well as pestaloficiols T–W (59–62) (Fig. 1).71 Ficiolide K 58 was found to contain a very rare 1,6-anhydropyranose moiety. Moreover, deletion of the putative histone acetyltransferase Hat1 in Metarhizium robertsii resulted in the characterizations of 11 new polyketides, meromusides A–I (63–71) and meromutides A 72 and B 73 (Fig. 1).72
All the abovementioned studies involved the genetic manipulation of the genes encoding enzymes that add or remove chemical moieties on histone tails. Most recently, another class of histone modifying proteins, the histone reader SntB, has been found to modify the BGC expression.46 By deleting sntB in A. flavus, seven known NPs and many uncharacterized secondary metabolites were aberrantly regulated.73 Moreover, in addition to genetically modifying epigenetic regulators, treatment with chemicals, such as DNA methyltransferase and histone deacetylase inhibitors, can control the native expression of fungal biosynthetic pathways and generate new bioactive SMs. Readers that are interested in chemical epigenetic methodologies for exploring novel NPs are referred to the reported literature.74
3.4 Other regulators as tools in accessing the structural diversity
In addition to the abovementioned regulators, several alternative regulator-based approaches can be used to discover new NPs. The COP9 signalosome (CSN) is a highly conserved protein complex implicated in diverse biological functions that involve ubiquitin-mediated proteolysis. By disrupting the fifth subunit of CSN in P. fici, the resultant strain ΔPfcsnE lost the ability to produce conidia along with remarkable changes in the SM output. In addition, five new structures, pestaloficins A–E (74–78), were thus isolated and characterized (Fig. 1).75 Pestaloficin A 74 represents a new type of dimeric cyclohexanone derivative linked through an unprecedented pentacyclic spiral ring. Cyclic adenosine monophosphate (cAMP)/protein kinase A (PKA) is one of the most studied signal transduction pathways that regulate gene expression and activation of secondary metabolism in fungi. Overexpression of the gene coding for the PKA catalytic subunit (PkaC1) increased the expressions of the fmp cluster genes in A. fumigatus and led to the isolation of a new NP, fumipyrrole 79.76
4. Combinational regulatory strategy to elucidate new fungal chemistry
As abovementioned, different regulator classes exhibit unique mechanisms by which new fungal chemistry can be accessed; for example, while the manipulation of both global and epigenetic regulators result in the alteration of the expression of multiple BGCs in a fungus, the mechanisms are quite different: one largely occurs through alteration of transcriptional networks and the other occurs through remodelling of chromatin; targeting of pathway-specific regulators results in the accurate activation of a specific BGC. Moreover, SM production is a complicated process because these metabolites are dedicated to the ecological or physiological functions of the fungus. Thus, fungal NP production is also controlled by various spatial and temporal inputs.14 Therefore, a universal system that integrates multiple regulators into a single model fungal host would be a powerful route to uncover novel fungal NP treasures. Herein, we proposed a combinational strategy for the effective and accurate discovery of new NPs in fungi.
As shown in Scheme 2, this strategy combines the integration of global/epigenetic regulators and pathway-specific regulators at different levels. As a demonstration, a BGC with no in-cluster transcriptional factor is selected. In Aspergillus spp., the expression of lovastatin is directly controlled by the pathway-specific regulator LovE, whereas the penicillin production is regulated by a higher regulatory network. However, both SMs are dramatically up-regulated in an OE::laeA mutant in Aspergillus spp.26 In this case, the LaeA-lovastatin/penicillin regulation system could be selected as a synthetic DNA gene-expressing UNIT. Using gene switching techniques such as via yeast assembly, the lovastatin and penicillin biosynthetic ORFs would be replaced by target genes of the desired cryptic cluster; this would leave the native promotors in the gene-expressing UNIT. This would allow the upregulation of cryptic genes by LaeA and additionally LovE in the case of using lovastatin promoters. This strategy would enable the BGC expression in the model host regardless of the BGC from any fungal strain. We can anticipate that with the characterization of more key regulators and mechanisms involved in the SM production, more efficient combinational strategies will be developed for mining novel fungal chemical diversity.
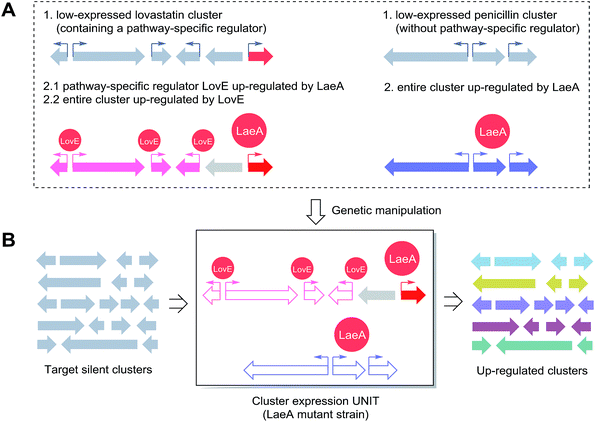 |
| Scheme 2 A proposed combinational strategy for activating targeted silent BGCs, using LaeA and LovE as a case study. (A) The expressions of the lovastatin (also containing a pathway specific transcription factor LovE) and penicillin (no specific transcription factor) gene clusters are dramatically up-regulated by overexpression of the global regulator LaeA in Aspergillus spp.26 (B) The hypothetical heterologous universal cluster expression UNIT. The LaeA/LovE regulation system can be utilized to substitute genes from BGCs of interest for penicillin or lovastatin ORFs in a tunable LaeA host strain. | |
5. Conclusions and prospects
With the advent of cheap sequencing and bioinformatics technologies, enabling the identification of a huge number of BGCs in fungal genomes, there is now an unprecedented potential for discovering specific SMs in fungi.4,5,10 Among the diverse genome-based strategies for exploring novel NPs, regulator manipulation exhibits a unique advantage: only one gene needs to be handled, avoiding the complicated genetic manipulation required for whole BGC pathway reconstruction. In this review, we report a total of 79 completely new compounds, including the biologically interesting compounds aspyridones A and B (1 and 2),32 the bioactive molecules fusarielins (16–18)40 and apicidin F 19,41 and the new skeleton structure pestaloficin A 74,75 discovered by applying these strategies. However, as in the case of any technology, there is still a need for improvement. For example, these achievements have been limited to fungi that are genetically accessible. In this regard, one future goal is to improve the efficiency of genetic manipulations in all fungi; possibly with the advent of the CRISPR technology, there is foreseeable improvement in this area.77 Moreover, a better understanding of the regulatory networks controlled by global or epigenetic regulators would result in new methods to harness this network to accurately activate silent NPs through alternative means. Keeping these challenging goals in mind, it is clear that regulator manipulation will continue to be a powerful and efficient tool for new NP discovery in fungi.
6. Conflicts of interest
We declare no conflicts of interest.
7. Acknowledgements
We thank Professor Shu-Ming Li (Philipps University of Marburg) for critical reading of the manuscript. This project was financially supported by the National Key Research and Development Program (2016YFD0400105), National Natural Science Foundation of China (31861133004), the Construction of the Registry and Database of Bioparts for Synthetic Biology of the Chinese Academy of Sciences (ZSYS-016) and the State Key Laboratory of Bioactive Substance and Function of Natural Medicines, Institute of Materia Medica, Chinese Academy of Medical Sciences and Peking Union Medical College (Grant GTZK201802). Wen-Bing Yin is a scholar of “the 100 Talents Project” of CAS.
8. References
- D. J. Newman and G. M. Cragg, J. Nat. Prod., 2012, 75, 311–335 CrossRef CAS PubMed.
- J. W. Bok, Y. M. Chiang, E. Szewczyk, Y. Reyes-Dominguez, A. D. Davidson, J. F. Sanchez, H. C. Lo, K. Watanabe, J. Strauss, B. R. Oakley, C. C. Wang and N. P. Keller, Nat. Chem. Biol., 2009, 5, 462–464 CrossRef CAS PubMed.
- Z. Liu, J. Y. Zhao, S. F. Sun, Y. Li and Y. B. Liu, J. Asian Nat. Prod. Res., 2018, 1–22, DOI:10.1080/10286020.2018.1488833.
- E. Choque, C. Klopp, S. Valiere, J. Raynal and F. Mathieu, BMC Genomics, 2018, 19, 200 CrossRef PubMed.
- I. Kjaerbolling, T. C. Vesth, J. C. Frisvad, J. L. Nybo, S. Theobald, A. Kuo, P. Bowyer, Y. Matsuda, S. Mondo, E. K. Lyhne, M. E. Kogle, A. Clum, A. Lipzen, A. Salamov, C. Y. Ngan, C. Daum, J. Chiniquy, K. Barry, K. LaButti, S. Haridas, B. A. Simmons, J. K. Magnuson, U. H. Mortensen, T. O. Larsen, I. V. Grigoriev, S. E. Baker and M. R. Andersen, Proc. Natl. Acad. Sci. U. S. A., 2018, 115, E753–E761 CrossRef PubMed.
- M. Machida, K. Asai, M. Sano, T. Tanaka, T. Kumagai, G. Terai, K. I. Kusumoto, T. Arima, O. Akita, Y. Kashiwagi, K. Abe, K. Gomi, H. Horiuchi, K. Kitamoto, T. Kobayashi, M. Takeuchi, D. W. Denning, J. E. Galagan, W. C. Nierman, J. J. Yu, D. B. Archer, J. W. Bennett, D. Bhatnagar, T. E. Cleveland, N. D. Fedorova, O. Gotoh, H. Horikawa, A. Hosoyama, M. Ichinomiya, R. Igarashi, K. Iwashita, P. R. Juvvadi, M. Kato, Y. Kato, T. Kin, A. Kokubun, H. Maeda, N. Maeyama, J. Maruyama, H. Nagasaki, T. Nakajima, K. Oda, K. Okada, I. Paulsen, K. Sakamoto, T. Sawano, M. Takahashi, K. Takase, Y. Terabayashi, J. R. Wortman, O. Yamada, Y. Yamagata, H. Anazawa, Y. Hata, Y. Koide, T. Komori, Y. Koyama, T. Minetoki, S. Suharnan, A. Tanaka, K. Isono, S. Kuhara, N. Ogasawara and H. Kikuchi, Nature, 2005, 438, 1157–1161 CrossRef PubMed.
- J. Macheleidt, D. J. Mattern, J. Fischer, T. Netzker, J. Weber, V. Schroeckh, V. Valiante and A. A. Brakhage, Annu. Rev. Genet., 2016, 50, 371–392 CrossRef CAS PubMed.
- A. A. Brakhage, Nat. Rev. Microbiol., 2013, 11, 21–32 CrossRef CAS PubMed.
- N. P. Keller, G. Turner and J. W. Bennett, Nat. Rev. Microbiol., 2005, 3, 937–947 CrossRef CAS PubMed.
- N. P. Keller, Nat. Rev. Microbiol., 2019, 17, 167–180 CrossRef CAS PubMed.
- T. Hautbergue, E. L. Jamin, L. Debrauwer, O. Puel and I. P. Oswald, Nat. Prod. Rep., 2018, 35, 147–173 RSC.
- E. M. Fox and B. J. Howlett, Curr. Opin. Microbiol., 2008, 11, 481–487 CrossRef CAS PubMed.
- N. P. Keller, Nat. Chem. Biol., 2015, 11, 671–677 CrossRef CAS PubMed.
- F. Y. Lim and N. P. Keller, Nat. Prod. Rep., 2014, 31, 1277–1286 RSC.
- M. Fernandes, N. P. Keller and T. H. Adams, Mol. Microbiol., 1998, 28, 1355–1365 CrossRef CAS PubMed.
- C. Jiang, C. Zhang, C. Wu, P. Sun, R. Hou, H. Liu, C. Wang and J. R. Xu, Environ. Microbiol., 2016, 18, 3689–3701 CrossRef CAS PubMed.
- K. F. Pedley and J. D. Walton, Proc. Natl. Acad. Sci. U. S. A., 2001, 98, 14174–14179 CrossRef CAS PubMed.
- E. K. Schmitt and U. Kuck, J. Biol. Chem., 2000, 275, 9348–9357 CrossRef CAS PubMed.
- N. P. Keller, C. Nesbitt, B. Sarr, T. D. Phillips and G. B. Burow, Phytopathology, 1997, 87, 643–648 CrossRef CAS PubMed.
- C. E. Dowzer and J. M. Kelly, Curr. Genet., 1989, 15, 457–459 CrossRef CAS PubMed.
- J. Tannous, D. Kumar, N. Sela, E. Sionov, D. Prusky and N. P. Keller, Mol. Plant Pathol., 2018, 19, 2635–2650 CrossRef CAS PubMed.
- M. J. Hynes, Aust. J. Biol. Sci., 1975, 28, 301–313 CrossRef CAS PubMed.
- A. Pfannmuller, J. Leufken, L. Studt, C. B. Michielse, C. M. K. Sieber, U. Guldener, S. Hawat, M. Hippler, C. Fufezan and B. Tudzynski, PLoS One, 2017, 12, e0176194 CrossRef PubMed.
- P. Hortschansky, M. Eisendle, Q. Al-Abdallah, A. D. Schmidt, S. Bergmann, M. Thon, O. Kniemeyer, B. Abt, B. Seeber, E. R. Werner, M. Kato, A. A. Brakhage and H. Haas, EMBO J., 2007, 26, 3157–3168 CrossRef CAS PubMed.
- O. Litzka, K. Then Bergh and A. A. Brakhage, Eur. J. Biochem., 1996, 238, 675–682 CrossRef CAS PubMed.
- J. W. Bok and N. P. Keller, Eukaryot. Cell, 2004, 3, 527–535 CrossRef CAS PubMed.
- D. Kumar, S. Barad, Y. Chen, X. Luo, J. Tannous, A. Dubey, N. Glam Matana, S. Tian, B. Li, N. Keller and D. Prusky, Mol. Plant Pathol., 2017, 18, 1150–1163 CrossRef CAS PubMed.
- M. G. Amare and N. P. Keller, Fungal Genet. Biol., 2014, 66, 11–18 CrossRef CAS PubMed.
- R. A. Butchko, D. W. Brown, M. Busman, B. Tudzynski and P. Wiemann, Fungal Genet. Biol., 2012, 49, 602–612 CrossRef CAS PubMed.
- O. Bayram, S. Krappmann, M. Ni, J. W. Bok, K. Helmstaedt, O. Valerius, S. Braus-Stromeyer, N. J. Kwon, N. P. Keller, J. H. Yu and G. H. Braus, Science, 2008, 320, 1504–1506 CrossRef CAS PubMed.
- Y. Reyes-Dominguez, J. W. Bok, H. Berger, E. K. Shwab, A. Basheer, A. Gallmetzer, C. Scazzocchio, N. Keller and J. Strauss, Mol. Microbiol., 2010, 76, 1376–1386 CrossRef CAS PubMed.
- S. Bergmann, J. Schumann, K. Scherlach, C. Lange, A. A. Brakhage and C. Hertweck, Nat. Chem. Biol., 2007, 3, 213–217 CrossRef CAS PubMed.
- Y. M. Chiang, E. Szewczyk, A. D. Davidson, N. Keller, B. R. Oakley and C. C. C. Wang, J. Am. Chem. Soc., 2009, 131, 2965–2970 CrossRef CAS PubMed.
- A. O. Zabala, W. Xu, Y. H. Chooi and Y. Tang, Chem. Biol., 2012, 19, 1049–1059 CrossRef CAS PubMed.
- T. Awakawa, X. L. Yang, T. Wakimoto and I. Abe, ChemBioChem, 2013, 14, 2095–2099 CrossRef CAS PubMed.
- X. L. Yang, T. Awakawa, T. Wakimoto and I. Abe, ChemBioChem, 2014, 15, 1578–1583 CrossRef CAS PubMed.
- T. Nakazawa, K. Ishiuchi, A. Praseuth, H. Noguchi, K. Hotta and K. Watanabe, ChemBioChem, 2012, 13, 855–861 CrossRef CAS PubMed.
- Y. H. Chooi, J. Fang, H. Liu, S. G. Filler, P. Wang and Y. Tang, Org. Lett., 2013, 15, 780–783 CrossRef CAS PubMed.
- T. Zhang, J. Wan, Z. Zhan, J. Bai, B. Liu and Y. Hu, Acta Pharm. Sin. B, 2018, 8, 478–487 CrossRef PubMed.
- J. L. Sorensen, F. T. Hansen, T. E. Sondergaard, D. Staerk, T. V. Lee, R. Wimmer, L. G. Klitgaard, S. Purup, H. Giese and R. J. Frandsen, Environ. Microbiol., 2012, 14, 1159–1170 CrossRef PubMed.
- K. W. von Bargen, E. M. Niehaus, K. Bergander, R. Brun, B. Tudzynski and H. U. Humpf, J. Nat. Prod., 2013, 76, 2136–2140 CrossRef CAS PubMed.
- J. A. Baccile, J. E. Spraker, H. H. Le, E. Brandenburger, C. Gomez, J. W. Bok, J. Macheleidt, A. A. Brakhage, D. Hoffmeister, N. P. Keller and F. C. Schroeder, Nat. Chem. Biol., 2016, 12, 419–424 CrossRef CAS PubMed.
- F. Y. Lim, T. H. Won, N. Raffa, J. A. Baccile, J. Wisecaver, A. Rokas, F. C. Schroeder and N. P. Keller, mBio, 2018, 9, e00785-18 CrossRef PubMed.
- M. Ahuja, Y. M. Chiang, S. L. Chang, M. B. Praseuth, R. Entwistle, J. F. Sanchez, H. C. Lo, H. H. Yeh, B. R. Oakley and C. C. Wang, J. Am. Chem. Soc., 2012, 134, 8212–8221 CrossRef CAS PubMed.
- M. F. Grau, R. Entwistle, Y. M. Chiang, M. Ahuja, C. E. Oakley, T. Akashi, C. C. C. Wang, R. B. Todd and B. R. Oakley, ACS Chem. Biol., 2018, 13, 3193–3205 CrossRef CAS PubMed.
- B. T. Pfannenstiel, X. Zhao, J. Wortman, P. Wiemann, K. Throckmorton, J. E. Spraker, A. A. Soukup, X. Luo, D. L. Lindner, F. Y. Lim, B. P. Knox, B. Haas, G. J. Fischer, T. Choera, R. A. E. Butchko, J. W. Bok, K. J. Affeldt, N. P. Keller and J. M. Palmer, mBio, 2017, 8, e01246-17 CrossRef PubMed.
- J. W. Bok, D. Noordermeer, S. P. Kale and N. P. Keller, Mol. Microbiol., 2006, 61, 1636–1645 CrossRef CAS PubMed.
- J. Tilburn, S. Sarkar, D. A. Widdick, E. A. Espeso, M. Orejas, J. Mungroo, M. A. Penalva and H. N. Arst Jr, EMBO J., 1995, 14, 779–790 CrossRef CAS PubMed.
- S. D. Polley and M. X. Caddick, FEBS Lett., 1996, 388, 200–205 CrossRef CAS PubMed.
- A. Tanaka, M. Kato, T. Nagase, T. Kobayashi and N. Tsukagoshi, Biochim. Biophys. Acta, 2002, 1576, 176–182 CrossRef CAS.
- Y. Cui, A. Chatterjee, Y. Liu, C. K. Dumenyo and A. K. Chatterjee, J. Bacteriol., 1995, 177, 5108–5115 CrossRef CAS PubMed.
- C. E. Oakley, M. Ahuja, W. W. Sun, R. Entwistle, T. Akashi, J. Yaegashi, C. J. Guo, G. C. Cerqueira, J. Russo Wortman, C. C. Wang, Y. M. Chiang and B. R. Oakley, Mol. Microbiol., 2017, 103, 347–365 CrossRef CAS PubMed.
-
J. W. B. N. P. Keller, in Biochemistry and Molecular Biology, ed. H. Dirk, Springer International Publishing, 2016, pp. 21–29 Search PubMed.
- H. B. Bode, B. Bethe, R. Hofs and A. Zeeck, ChemBioChem, 2002, 3, 619–627 CrossRef CAS PubMed.
- T. Jiang, M. Wang, L. Li, J. Si, B. Song, C. Zhou, M. Yu, X. Wang, Y. Zhang, G. Ding and Z. Zou, J. Nat. Prod., 2016, 79, 2487–2494 CrossRef CAS PubMed.
- H. Lin, H. Lyu, S. Zhou, J. Yu, N. P. Keller, L. Chen and W. B. Yin, Org. Biomol. Chem., 2018, 16, 4973–4976 RSC.
- B. T. Pfannenstiel and N. P. Keller, Biotechnol. Adv., 2019 DOI:10.1016/j.biotechadv.2019.02.001.
- M. Gandhi, B. L. Goode and C. S. Chan, Genetics, 2006, 174, 665–678 CrossRef CAS PubMed.
- A. Shilatifard, Annu. Rev. Biochem., 2006, 75, 243–269 CrossRef CAS PubMed.
- H. W. Nutzmann, Y. Reyes-Dominguez, K. Scherlach, V. Schroeckh, F. Horn, A. Gacek, J. Schumann, C. Hertweck, J. Strauss and A. A. Brakhage, Proc. Natl. Acad. Sci. U. S. A., 2011, 108, 14282–14287 CrossRef CAS PubMed.
- K. Scherlach, H. W. Nutzmann, V. Schroeckh, H. M. Dahse, A. A. Brakhage and C. Hertweck, Angew. Chem., Int. Ed. Engl., 2011, 50, 9843–9847 CrossRef CAS PubMed.
- A. A. Soukup, Y. M. Chiang, J. W. Bok, Y. Reyes-Dominguez, B. R. Oakley, C. C. Wang, J. Strauss and N. P. Keller, Mol. Microbiol., 2012, 86, 314–330 CrossRef CAS PubMed.
- E. K. Shwab, J. W. Bok, M. Tribus, J. Galehr, S. Graessle and N. P. Keller, Eukaryot. Cell, 2007, 6, 1656–1664 CrossRef CAS PubMed.
- M. Shimizu, S. Masuo, T. Fujita, Y. Doi, Y. Kamimura and N. Takaya, Mol. Cell. Biol., 2012, 32, 3743–3755 CrossRef CAS PubMed.
- M. T. Henke, A. A. Soukup, A. W. Goering, R. A. McClure, R. J. Thomson, N. P. Keller and N. L. Kelleher, ACS Chem. Biol., 2016, 11, 2117–2123 CrossRef CAS PubMed.
- S. Graessle, M. Dangl, H. Haas, K. Mair, P. Trojer, E. M. Brandtner, J. D. Walton, P. Loidl and G. Brosch, Biochim. Biophys. Acta, 2000, 1492, 120–126 CrossRef CAS.
- M. Kawauchi, M. Nishiura and K. Iwashita, Eukaryot. Cell, 2013, 12, 1087–1096 CrossRef CAS PubMed.
- A. Gacek-Matthews, L. M. Noble, C. Gruber, H. Berger, M. Sulyok, A. T. Marcos, J. Strauss and A. Andrianopoulos, Mol. Microbiol., 2015, 96, 839–860 CrossRef CAS PubMed.
- T. Satterlee, J. W. Cary and A. M. Calvo, PLoS One, 2016, 11, e0155575 CrossRef PubMed.
- X. M. Mao, W. Xu, D. Li, W. B. Yin, Y. H. Chooi, Y. Q. Li, Y. Tang and Y. Hu, Angew. Chem., Int. Ed. Engl., 2015, 54, 7592–7596 CrossRef CAS PubMed.
- G. Wu, H. Zhou, P. Zhang, X. Wang, W. Li, W. Zhang, X. Liu, H. W. Liu, N. P. Keller, Z. An and W. B. Yin, Org. Lett., 2016, 18, 1832–1835 CrossRef CAS PubMed.
- A. Fan, W. Mi, Z. Liu, G. Zeng, P. Zhang, Y. Hu, W. Fang and W. B. Yin, Org. Lett., 2017, 19, 1686–1689 CrossRef CAS PubMed.
- B. T. Pfannenstiel, C. Greco, A. T. Sukowaty and N. P. Keller, Fungal Genet. Biol., 2018, 120, 9–18 CrossRef CAS PubMed.
- R. H. Cichewicz, Nat. Prod. Rep., 2010, 27, 11–22 RSC.
- Y. Zheng, K. Ma, H. Lyu, Y. Huang, H. Liu, L. Liu, Y. Che, X. Liu, H. Zou and W. B. Yin, Org. Lett., 2017, 19, 4700–4703 CrossRef CAS PubMed.
- J. Macheleidt, K. Scherlach, T. Neuwirth, W. Schmidt-Heck, M. Strassburger, J. Spraker, J. A. Baccile, F. C. Schroeder, N. P. Keller, C. Hertweck, T. Heinekamp and A. A. Brakhage, Mol. Microbiol., 2015, 96, 148–162 CrossRef CAS PubMed.
- A. Idnurm and V. Meyer, Fungal Biol. Biotechnol., 2018, 5, 19 CrossRef PubMed.
|
This journal is © The Royal Society of Chemistry 2020 |