DOI:
10.1039/C9NH00707E
(Communication)
Nanoscale Horiz., 2020,
5, 857-868
Defect engineering of 2D BiOCl nanosheets for photonic tumor ablation†
Received
10th November 2019
, Accepted 5th February 2020
First published on 5th February 2020
Abstract
Photothermal therapy (PTT) is an emerging technology as a noninvasive therapeutic modality for inducing photonic cancer hyperthermia. However, current photothermal conversion agents suffer from low therapeutic efficiency and single functionality. Engineering crystal defects on the surface or substrate of semiconductors can substantially enhance their optical absorption capability as well as improve their photothermal effects in theranostic nanomedicines. In this study, a specific defect engineering strategy was developed to endow two-dimensional (2D) BiOCl nanosheets with intriguing photothermal conversion performance by creating oxygen vacancies on the surface (O-BiOCl). Importantly, the photothermal performance and photoacoustic imaging capability of the 2D O-BiOCl nanosheets could be precisely controlled by modulating the amounts of oxygen vacancies. The strong Bi-based X-ray attenuation coefficient endowed these nanosheets with the contrast-enhanced computed tomography imaging capability. The high near-infrared-triggered photonic hyperthermia for tumor ablation was systematically demonstrated both in vitro at the cellular level and in vivo for tumor breast cancer mice xenograft models. Based on the demonstrated high biocompatibility of these 2D O-BiOCl nanosheets, this work not only formulates an intriguing 2D photothermal nanoagent for tumor ablation, but also provides an efficient strategy to control the photothermal performance of nanoagents by defect engineering.
New concepts
By understanding the principles from intrinsic physiochemical properties and photocatalysis chemistry, two-dimensional (2D) oxygen-vacancy-engineered BiOCl nanosheets have been constructed and developed for efficient cancer theranostics by a specific defect engineering strategy, which broadens the medical applications of advanced 2D nanosheets. This work offers a paradigm in which 2D BiOCl nanosheets can achieve specific biomedical applications by surface-means of defect engineering. After rationally tuning and optimizing the energy bandgap, near-infrared optical absorbance, and photothermal conversion performance, the designed oxygen-vacancy-engineered BiOCl nanosheets have been employed as multifunctional theranostic agents for efficient CT (by strong X-ray attenuation ability of Bi-based composition) and PA (by high photothermal conversion capability of oxygen-vacancy-engineered BiOCl nanosheets) dual-modality imaging-guided photonic cancer ablation, which has been systematically demonstrated both in vitro and in vivo.
|
Introduction
The development of nanotechnology has highly promoted and inspired researchers to engineer and design versatile nanoagents or nanodrugs with desirable properties and high performance for combating cancers; they can substantially enrich the theories and methodologies of cancer therapy.1–11 Photothermal therapy (PTT), as a promising standalone alternative that generates heat in response to exogenous light irradiation for tumor ablation, has received ever-increasing attention due to its low cost, easy location, high efficiency, and minimal side-effects.12–15 In addition, a desirable photothermal agent used in PTT necessitates high light extinction coefficient and strong photothermal transduction efficiency, which refer to the desirable light absorption and efficiency of thermal energy conversion from light, respectively. In view of this, various nanoplatforms have been explored to accumulate into tumor sites for executing efficient PTT, such as Au-based nanoagents,16–19 WS2,20,21 upconversion nanocomposites,22 carbon-based nanomaterials,23–25 and functional polymers.26,27 However, most of these explored inorganic nanoagents suffer from low photothermal conversion efficiency, while organic polymer nanoparticles (NPs) exhibit poor light extinction coefficient.28 Currently, several strategies have been proposed to enhance the PTT efficacy. For example, the modification of tumor-cells-/subcellular organelles-targeting ligands on the surface of nanoagents could be applied to enhance the recognition and internalization of nanoagents by cancer cells/subcellular organelles, potentiating the therapeutic effects of PPT.29 In addition, the surface plasmon resonance (SPR)-based photothermal effect is related to the shape and size of these nanoagents as per the Mie theory,30 which makes it an efficient strategy to tune the photothermal performance.31,32 However, PTT alone is still far from satisfactory because of its suboptimal therapeutic potency. Therefore, it is still a formidable challenge to fabricate desirable nanoagents with strong light absorption ability and high photothermal convention efficiency, which are expected to subsequently reduce the doses of agents and power density of light with minimal side-effects to normal tissues/cells.
On the basis of the intrinsic features, such as a tunable light absorption range and high biosafety, semiconducting NPs have been extensively explored as promising PTT nanoagents.33 In particular, modulating the bandgap of semiconducting NPs could be a direct approach to enhance their photothermal performance. The traditional doping of metallic or nonmetallic impurities into the original composition of semiconducting agents can generate donor or acceptor states in the bandgap, which can further result in improved light absorption characteristics.34,35 Very recently, TiO2−x nanoplatforms were established for synergistic photodynamic/photothermal cancer therapy by the creation of oxygen vacancies within the matrix by reducing the treatment time under high temperature and inert atmosphere.36 However, these nanoplatforms suffer from the requirements of complex synthesis processes and suboptimal therapeutic outcomes, as well as requiring precise control over the unique physicochemical/biochemical characteristics and smart mediation of therapeutic efficiency for further clinical translation and personalized medicine. Therefore, it is still highly urgent to discover and explore promising agents and potential approaches to achieve the maximal photothermal therapeutic effect and exercise precise control over the therapeutic potency.
Recently, two-dimensional (2D) nanomaterials have been explored in theranostic applications because of their ultrathin nanostructures, large specific surface areas, and unique physiochemical properties.37–40 Since the discovery of graphene, considerable 2D nanomaterials with outstanding properties have been discovered and successfully developed, some of which were preliminarily explored and applied in ever-restricted nanomedicine, particularly for fighting cancers. These 2D nanosystems include transition metal dichalcogenides,41,42 palladium (Pd) nanosheets,43 MXenes,44,45 and black phosphorus (BP).32,46 2D BiOCl—a p-block semiconductor—features excellent photocatalytic properties that are comparable to or even better than those of traditional anatase TiO2.47–49 In a typical 2D BiOCl crystal structure, a fluorite-like [Bi2O2] slab is sandwiched by double slabs of halogen atoms in the tetragonal structure.50 Because of the low bond energy and long bond length of the Bi–O bond, surface oxygen vacancy defects on the 2D BiOCl crystal facet are easily introduced under ultraviolet (UV)-light irradiation.50–52 These oxygen vacancies act as active sites to narrow the bandgap by the introduction of new orbitals in the bandgap; consequently, the photocatalytic activity could be substantially improved. With regard to biomedical applications, although BiOCl nanosheets have been preliminarily reported to serve as photodynamic agents for photodynamic tumor therapy,53 more studies are required for the further in vivo study and exploration of other high-efficiency medical applications. Benefiting from the excellent photonic response, the application of BiOCl nanosheets with large amounts of oxygen vacancies to act as photothermal nanoagents under near-infrared (NIR)-light irradiation is a potential strategy for efficient photonic tumor ablation.
In this work, we report an intriguing defect engineering strategy to endow semiconductor-based nanoagents with photonic hyperthermia performance, based on which 2D BiOCl nanosheets with surface oxygen vacancies were successfully fabricated for achieving photoacoustic (PA)/computed tomography (CT) dual-modal bioimaging and NIR-triggered photothermal tumor ablation. Typically, the roles of oxygen vacancies in BiOCl semiconductors were mostly explored in the process of photocatalysis for enhancing the photocatalytic activity, such as H2 production54 and CO2 photoreduction.55 Herein, oxygen vacancies on the surface of BiOCl nanosheets could be easily tuned by a facile and efficient treatment by exposure to UV-light irradiation. Theoretical simulations performed by means of density functional theory (DFT) as well as the experimental data prove that the bandgap could be efficiently tuned by introducing oxygen vacancies onto the surface of BiOCl nanosheets, which bestowed these nanosheets with strong light absorption ability and high photothermal conversion efficiency in the NIR biowindow. In addition, Bi-based BiOCl nanosheets with a high atomic number (Z = 83) and strong X-ray attenuation coefficient endowed them with superior X-ray CT contrast for CT imaging. Finally, after surface organic engineering with polyvinylpyrrolidone (PVP), the as-obtained O-BiOCl-PVP nanosheets exhibited high biosafety and biocompatibility, efficiently achieving in vivo dual-modal CT-/PA-imaging-guided photonic tumor hyperthermia.
Results and discussion
Synthesis and characterization of BiOCl nanosheets
Herein, 2D-layer-structured BiOCl nanosheets were fabricated by a facile hydrothermal method. Typically, Bi(NO3)3·5H2O and PVP were dissolved in a mannitol aqueous solution under vigorous stirring for 60 min followed by the addition of saturated KCl solution dropwise for another 60 min under agitation, which was further transferred into a Teflon-lined stainless steel autoclave and heated up to 160 °C for 4 h to obtain 2D BiOCl nanosheets. Subsequently, oxygen vacancies were induced by UV-light irradiation onto the surface of the BiOCl nanosheets (denoted as O-BiOCl), mainly attributable to the weak bond energy and long bond length of the Bi–O bond, as well as the oxygen atom density on the surface of the BiOCl nanosheets. High oxygen atom density in the {001} facets of the BiOCl nanosheets was considered to be the main cause for the generation of UV-light-triggered oxygen vacancies.56 To satisfy the biomedical requirements, a typical organic PVP molecule was chosen to modify the surface of the O-BiOCl nanosheets (denoted as O-BiOCl-PVP, Fig. 1a). After precisely regulating the oxygen vacancy generation on the surface of the O-BiOCl-PVP nanosheets by UV-light irradiation, these designed O-BiOCl-PVP nanosheets demonstrated their high photothermal convention and efficient accumulation into the tumor site via the typical enhanced permeability and retention (EPR) effect (Fig. 1b). Thereafter, the PA-/CT-imaging-guided efficient PTT ablation of tumors was achieved.
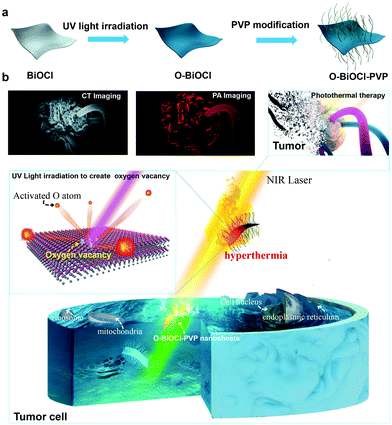 |
| Fig. 1 Schematic of the synthesis procedure and PA-/CT-imaging-guided photothermal cancer therapy as assisted by 2D O-BiOCl-PVP nanosheets. (a) Schematic of the synthesis process for 2D O-BiOCl-PVP nanosheets, including hydrothermal treatment and the generation of oxygen vacancies by UV-light irradiation. (b) Schematic illustration of the intrinsic theranostic functions of O-BiOCl-PVP nanosheets, i.e., PA-/CT-imaging-guided efficient PTT ablation of tumors. | |
The morphological features of the as-obtained 2D BiOCl nanosheets were characterized by scanning electron microscopy (SEM) (Fig. 2a). Evidently, the BiOCl nanosheets exhibited square-like nanosheet topology with sizes in the range of ∼150–250 nm. The transmission electron microscopy (TEM) images of the BiOCl nanosheets also showed a tetragonal structure, which was in accordance with the TEM characterization (Fig. S1, ESI†). After UV-light irradiation, the TEM images of the O-BiOCl nanosheets with oxygen vacancies exhibited that there were no obvious changes in the shape and size of the BiOCl and O-BiOCl nanosheets (Fig. 2b). High-resolution TEM (HRTEM) images revealed that the O-BiOCl nanosheets featured clear lattice fringes after UV-light treatment, indicating that the crystalline nature of the BiOCl nanosheets was effectively preserved. In particular, the outer layer of black O-BiOCl nanosheets became blurred and disordered at the edges, which can be attributed to the formation of oxygen vacancies, while the outer surface of the white BiOCl nanosheets was fairly intact and clear (Fig. 2c). Atomic force microscopy (AFM) was performed to further assess the thickness of the as-prepared O-BiOCl nanosheets. The obtained results showed the topographic morphology of the O-BiOCl nanosheets, yielding a height of ∼9–12 nm (Fig. 2d–f). X-ray energy-dispersive spectroscopy (EDS, Fig. S2, ESI†) further demonstrated the presence of elemental Cl, O, and Bi in the O-BiOCl nanosheets. The phase purity and crystallinity of the BiOCl nanosheets before and after UV-light irradiation were confirmed by X-ray diffraction (XRD) analysis (Fig. 2g). The XRD patterns of the BiOCl nanosheets could be effectively assigned to the tetragonal phase with the lattice parameters a = b = 0.3887 nm and c = 0.7354 nm. The presented obvious peaks at 11.6°, 23.88°, 25.94°, 32.54°, 33.48°, 34.8°, 36.95°, 41.06°, 46.7°, 48.4°, 49.46°, 53.38°, 54.18°, 54.34° and 58.62° could be indexed to the (001), (002), (101), (110), (102), (111), (003), (112), (200), (201), (113), (202), (211), (104), and (212) crystal facets of BiOCl nanosheets, respectively.57 It should be noted that the O-BiOCl nanosheets maintained their high crystallinity without any impurity peaks after UV-light irradiation treatments. The relative intensity of the XRD peaks and crystal parameters between the BiOCl and O-BiOCl nanosheets showed no obvious changes, demonstrating that the morphology, crystal size, and orientation of the O-BiOCl nanosheets were not affected by UV-light irradiation.
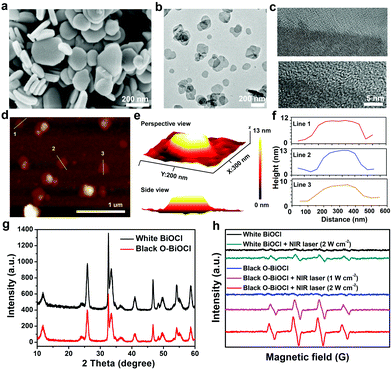 |
| Fig. 2 Characterization of BiOCl nanosheets. (a) SEM image of BiOCl nanosheets. (b) TEM images of the O-BiOCl nanosheets after exposure to UV irradiation. (c) HRTEM image of BiOCl (above) and O-BiOCl (below) nanosheets. (d) AFM image of ultrathin O-BiOCl nanosheets. (e) Three-dimensional AFM image of ultrathin O-BiOCl nanosheets. (f) AFM-measured thickness of O-BiOCl nanosheets. (g) XRD patterns of BiOCl and O-BiOCl nanosheets. (h) ESR spectra of ˙OH trapped by DMPO in the presence of BiOCl and O-BiOCl nanosheets after NIR-laser irradiation for 300 s. | |
In order to understand the influence of oxygen vacancies on the reactive oxygen species (ROS) production, the electron spin resonance (ESR) technique was employed to determine the ROS generation by the BiOCl and O-BiOCl nanosheets under 808 nm-laser irradiation. Initially, 2,2,6,6-tetramethylpiperidine (TEMP) was used as the 1O2 trapper, which selectively reacted with 1O2 to generate 2,2,6,6-tetramethylpiperidine-1-oxyl and induced the formation of the characteristic 1
:
1
:
1 triplet signal in the ESR spectrum. As shown in Fig. S3 (ESI†), no obvious 1O2 signals were observed in the presence of BiOCl nanosheets or O-BiOCl nanosheets after NIR-laser irradiation. After that, 5,5′-dimethylpyrroline-1-oxide (DMPO) was utilized as the spin trap agent to detect the generation of hydroxyl radicals (˙OH), which could introduce the characteristic ESR signal intensity of 1
:
2
:
2
:
1 (Fig. 2h). The obtained results exhibited that the ESR signals of typical ˙OH radicals were observed after 808 nm-laser irradiation in the presence of O-BiOCl nanosheets; the ESR signal intensity was higher than that of the BiOCl nanosheets at the same laser power density, mainly because of the enhanced light absorption of UV-triggered oxygen-vacancies-based BiOCl nanosheets. It is well known that ˙OH radicals are highly toxic to tumor cells. Therefore, benefiting from the semiconductor structure of the O-BiOCl nanosheets, the ˙OH-enhanced PTT could be potentially induced.
The oxygen vacancies as introduced in the lattice of the surface/subsurface of white BiOCl nanosheets are the intrinsic mechanism that results in the formation of black O-BiOCl. To further explore the photo-absorption mechanism of black O-BiOCl with oxygen vacancies, a theoretical simulation was performed by DFT. To visualize the electron distribution inside the lattice of BiOCl nanosheets, the electron density distribution, electron density difference, and electron localized function (ELF) were calculated, as shown in Fig. S4 (ESI†). The electron density difference indicated the charge depletion around the O atom and charge accumulation around the Cl atoms (Fig. S4a and b, ESI†). The ELF value (lower than 1) further revealed a strong delocalized electron character around the O atom, which was in line with the result of the ELF of BiOCl (Fig. S4c, ESI†). BiOCl supercells with different oxygen vacancy substitution ratios (0, 0.125, 0.25, and 0.5) were also studied. The band structures (Fig. S5a–d, ESI†) and density of states (DOS, Fig. S5e–h, ESI†) for the BiOCl nanosheets were further calculated. The valence band mainly stemmed from the hybrid Cl-3p and O-2p orbitals. For black BiOCl with oxygen vacancies, the nature of the VB maximum remained unchanged. The conduction bands of BiOCl were mainly composed of 6p orbitals of bismuth atoms and the intensities were essentially the same after the introduction of oxygen vacancies. However, new electronic states were produced, which resulted in the formation of the midgap states and the surface properties were changed, directly leading to the stronger light adsorption ability of BiOCl. The bandgap was effectively tuned as a result of the introduction of oxygen vacancies. Furthermore, as shown in Fig. S5a–d (ESI†), the bandgaps of BiOCl gradually decreased along with the increasing ratio of oxygen vacancies.
Oxygen vacancy, a type of point defect, can be revealed by the ESR spectra. In order to facilitate the emergence of the ESR signal from BiOCl nanosheets, all the as-obtained samples were heated in a tube furnace at 300 °C in an argon atmosphere for 2 h before testing. Fig. 3a shows the room-temperature EPR spectra of the BiOCl nanosheets before and after UV-light irradiation. The obtained results showed an obvious signal at a g-value of 2.002 in the BiOCl nanosheets after 8 h of UV-light irradiation, which was the typical characteristic of oxygen vacancies.51 However, there was no EPR signal before UV-light irradiation. XPS was performed to reveal the chemical composition and status of the BiOCl and O-BiOCl nanosheets (Fig. 3b and Fig. S6, ESI†). Binding energies centered at 164.4 and 159.1 eV were assigned to Bi 4f5/4 and Bi 4f7/4 of BiOCl, respectively. When compared with the white BiOCl nanosheets, the Bi 4f peaks of the O-BiOCl nanosheets were shifted to a higher binding energy, attributable to the formation of neighboring oxygen vacancies that could trap electrons due to their positive charge.57 At the same time, the higher binding energy of O in O-BiOCl nanosheets centered at 531 and 533 eV could be attributed to the coordination of oxygen in C–O–H, CO, and OH groups or rationally adsorbed oxygen (Fig. S6, ESI†).57
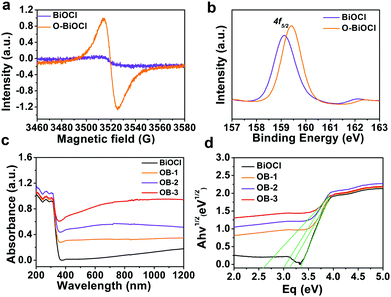 |
| Fig. 3 Characterization and bandgap calculation for O-BiOCl nanosheets. (a) ESR spectra of BiOCl and O-BiOCl nanosheets with oxygen vacancies. (b) XPS of Bi 4p region in BiOCl and O-BiOCl nanosheets. (c and d) UV-vis DRS and allowed indirect transitions of the different as-prepared BiOCl nanosheets. | |
The defect engineering of BiOCl was highly effective toward promoting the photocatalytic performance.50,58 According to earlier theoretical and experimental results, the introduction of oxygen vacancies could reduce the bandgap and provide a new electron donor level appearing in the bandgap of BiOCl nanosheets, where the absorption extended from the UV to visible and NIR regimes after introducing disorders and defects, resulting in the enhancement of light absorption. UV-visible (UV-vis) diffuse reflectance spectroscopy was performed to investigate the impact of doping on the optical properties of BiOCl and O-BiOCl nanosheets with different oxygen vacancies (Fig. 3c). Three representative branches of O-BiOCl nanosheets subjected to 2, 6, and 12 h of UV-light irradiation were designated as OB-1, OB-2, and OB-3 nanosheets, respectively. The colors of the white BiOCl and O-BiOCl nanosheets gradually changed from white to black as the amounts of oxygen vacancies increased, which provided indirect evidence that the oxygen vacancies could extend the light absorption ability of BiOCl into the visible-light and NIR regions (Fig. S7, ESI†). The absorbance of BiOCl nanosheets was enhanced with an increase in the oxygen vacancies in the BiOCl nanosheets. To further understand the origins of the change in the optical properties of BiOCl from oxygen vacancies, the plots of the transformed Kubelka–Munk function versus the energy of light (Fig. 3d) yielded bandgap energies of 3.35, 3.15, 3.0, and 2.6 eV for white BiOCl, OB-1, OB-2, and OB-3, respectively, indicating that the oxygen vacancies in the BiOCl samples had an significant impact on the band structures and therefore played a key role in enhancing the light absorption ability.
In vitro photothermal property of O-BiOCl nanosheets
The oxygen vacancies in BiOCl nanosheets could be easily introduced by UV-light irradiation (Fig. 4a). To explore how the oxygen vacancies affect the photothermal performances of the O-BiOCl nanosheets, the photothermal conversion efficiencies of OB-1, OB-2, and OB-3 were initially calculated. The UV-vis spectra of the O-BiOCl nanosheets exhibited concentration-dependent light absorption in the NIR region and the extinction coefficients of OB-1, OB-2, and OB-3 at 808 nm were calculated to be 6.02, 6.64, and 6.93 Lg−1 cm−1, respectively (Fig. S8, ESI†), whereas the extinction coefficients of the highly investigated graphene oxide was calculated to be 3.6 Lg−1 cm−1.59 The results elucidated that with an increase in the oxygen vacancies in the O-BiOCl nanosheets, their light absorption abilities got accordingly enhanced. Thereafter, we tested the in vitro photothermal performance of OB-1, OB-2, and OB-3 nanosheets for the same Bi concentration of 200 μg mL−1 after exposure to an 808 nm NIR laser at a power density of 2.0 W cm−2 (Fig. 4b–d). The maximum temperature of OB-3 could reach up to nearly 65 °C, which was 8 and 20 °C higher than those of the OB-2 and OB-1 nanosheets, respectively.
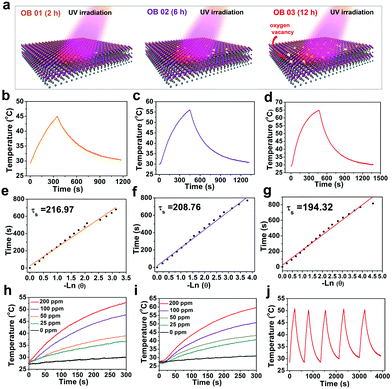 |
| Fig. 4
In vitro photothermal features of O-BiOCl treated for different time durations of UV-light irradiation. (a) 3D schematic illustration of O-BiOCl nanosheets containing different amounts of oxygen vacancies after exposure to UV-light irradiation for prolonged treatment time durations (2, 6, and 12 h). (b–d) Photothermal effect of an aqueous suspension of O-BiOCl nanosheets after exposure to UV-light irradiation for prolonged treatment time durations (2, 6, and 12 h) under 808 nm-laser irradiation (2.0 W cm−2). (e–g) Time constant of O-BiOCl nanosheets after exposure to UV-light irradiation with prolonged treatment time durations (2, 6, and 12 h) for heat transfer from the system as determined by using the linear time data from the cooling period versus the negative natural logarithm of the driving force temperature as obtained from the cooling stage. (h) Photothermal heating curves of O-BiOCl nanosheets at elevated concentrations under 808 nm-laser irradiation at a power density of 1.5 W cm−2. (i) Photothermal heating curves of O-BiOCl nanosheets at elevated concentrations under 808 nm-laser irradiation at a power density of 2.0 W cm−2. (j) Recycling heating profiles of O-BiOCl nanosheets in an aqueous solution after 808 nm-laser irradiation at a power density of 2.0 W cm−2 for 5 laser on/off cycles. | |
The photothermal conversion efficiency, η, for OB-1, OB-2, and OB-3 nanosheets were calculated to be 5.5%, 11.5%, and 13.9%, respectively (Fig. 4e–g), further confirming that the photothermal conversion performance of the O-BiOCl nanosheets was highly dependent on the oxygen vacancies. The η value of the OB-3 nanosheets was proven to be comparable to that of traditional Au nanoshells (13%).60 Subsequently, an aqueous solution containing O-BiOCl nanosheets was investigated at power densities of 1.5 and 2.0 W cm−2 after 300 s of 808 nm-laser irradiation; concentration-/laser-power-dependent photothermal features were observed (Fig. 4h and i). The photothermal stability of the O-BiOCl nanosheets (200 ppm) was also evaluated. There were insignificant variations during 5 laser on/off cycles even at a high power density of 2.0 W cm−2 for nearly 1 h (Fig. 4j).
To further guarantee the in vivo theranostic application of O-BiOCl nanosheets, the typical biocompatible organic PVP molecules were used to modify the surface of the O-BiOCl nanosheets (abbreviated as O-BiOCl-PVP). As shown in Fig. S9 (ESI†), the FTIR spectrum of the O-BiOCl-PVP nanosheets below 2000 cm−1 displayed a similar trend as that for PVP, where the characteristic peaks located at 1291, 1469, and 1685 cm−1 were indexed to C–N stretching, bending scissoring vibrations of the CH2 group, and C
O group of PVP, respectively, indicating the successful surface modification of O-BiOCl nanosheets by PVP. The colloidal stability of O-BiOCl-PVP nanosheets was significantly improved under the physiological condition, such as Dulbecco's modified Eagle's medium (DMEM), H2O, phosphate-buffered saline (PBS), and simulated body fluid (SBF) with a hydrodynamic size of about 190 nm, as measured by dynamic light scattering (DLS) measurements (Fig. 5a and b). Changes in the zeta potential of the O-BiOCl-PVP nanosheets before and after modification further evidenced the successful grafting of PVP on the surface of the O-BiOCl nanosheets (Fig. S10, ESI†).
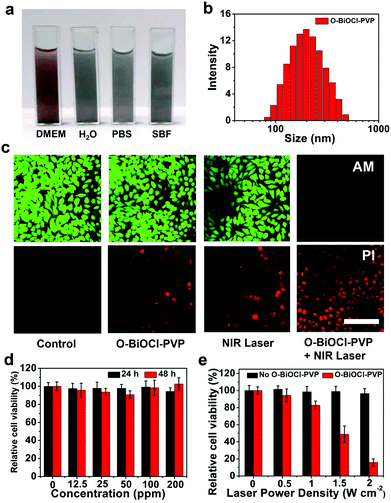 |
| Fig. 5 Dispersity characterization of O-BiOCl-PVP nanosheets after surface PVP modification and in vitro PTT against tumor cells enabled by O-BiOCl-PVP nanosheets. (a) Digital images of O-BiOCl-PVP nanosheets dispersed in different physiological solutions, such as DMEM, H2O, PBS, and SBF. (b) DLS data of O-BiOCl-PVP nanosheets in an aqueous solution. (c) CLSM images of 4T1 cancer cells stained by calcein AM and PI after different treatments, namely, untreated control, only O-BiOCl-PVP, only NIR laser, and O-BiOCl-PVP + NIR laser. All the scale bars are 50 μm. (d) Relative viabilities of 4T1 cells after incubation with O-BiOCl-PVP nanosheets at elevated Bi concentrations (0, 12.5, 25, 50, 100, and 200 μg mL−1) for 24 and 48 h. Error bars were based on the standard deviations (SD) of five parallel samples. (e) Relative viabilities of 4T1 cells after incubation with O-BiOCl-PVP nanosheets (Bi concentration: 200 μg mL−1) followed by exposure to NIR-laser irradiation for increasing laser power densities (0, 0.5, 1, 1.5, and 2.0 W cm−2). | |
In vitro photothermal ablation of tumor cells by O-BiOCl-PVP nanosheets
The in vitro cytotoxicity of O-BiOCl-PVP nanosheets serving as photothermal agents was initially determined using the 4T1 cancer cell line. After passing through incomplete blood vessels of the tumor and entering the cancer cells, O-BiOCl-PVP nanosheets with oxygen vacancies could absorb NIR-light irradiation followed by converting light into thermal energy and triggering intracellular hyperpyrexia, inducing cancer cell death. Then, the photothermal ablation of 4T1 cancer cells in the presence of O-BiOCl-PVP nanosheets after 4 h of coincubation followed by exposure to 808 nm-laser irradiation was confirmed by the fluorescence images of calcein AM (green) and PI (red) co-stained 4T1 cancer cells. After 808 nm-laser irradiation, the live and dead cells were distinguished by calcein AM and PI costaining, respectively. It should be noted that after laser irradiation, a majority of 4T1 cancer cells in the O-BiOCl-PVP + NIR laser group were killed, which was demonstrated by the presence of strong red fluorescence, while the viability of 4T1 cancer cells in the other groups, namely, the control, O-BiOCl-PVP, and NIR-laser groups, had an insignificant impact on the 4T1 cancer cell viabilities, which was confirmed by the presence of strong green fluorescence (Fig. 5c).
The in vitro toxicities of the BiOCl-PVP nanosheets on 4T1 cancer cells were evaluated by using the standard cell counting kit-8 (CCK-8) protocol. After coincubation of O-BiOCl-PVP nanosheets with 4T1 cancer cells for increasing Bi concentrations (12.5, 25, 50, 100, and 200 μg mL−1) for 24 and 48 h, the O-BiOCl-PVP nanosheets exhibited a negligible impact on the survival of 4T1 cancer cells, even when the Bi concentrations was as high as 200 μg mL−1. This demonstrated that the O-BiOCl-PVP nanosheets had excellent cytocompatibility (Fig. 5d). Then, the in vitro photothermal ablation efficiency of O-BiOCl-PVP nanosheets as photothermal agents for the ablation of 4T1 cancer cells was evaluated. After coincubation with increasing Bi concentrations from 0, 30, 60, 120, and 240 μg mL−1 for 4 h, the 4T1 cancer cells were treated by 808 nm-laser irradiation at a power density of 2.0 W cm−2 for 5 min (Fig. S11, ESI†). The inhibition rate of cancer cells (Bi concentration: 240 μg mL−1) after 808 nm-laser irradiation could reach up to nearly 84%. After that, the viabilities of 4T1 cancer cells coincubating with O-BiOCl-PVP nanosheets at a Bi concentration of 200 μg mL−1 irradiated by an 808 nm laser at increasing power densities of 0, 0.5, 1.0, 1.5, and 2.0 W cm−2 were tested. It should be noted that as the power density increased, more 4T1 cancer cells were killed (Fig. 5e), revealing the power-density-dependent feature of the O-BiOCl-PVP nanosheets. Such high in vitro photothermal ablation efficiency against 4T1 cancer cells induced by O-BiOCl-PVP nanosheets after NIR-laser irradiation guarantees their further in vivo photothermal application and translation abilities.
Dual-modal (CT and PA) bioimaging performances of O-BiOCl-PVP nanosheets
Precise spatial- and temporal-specific monitoring during the photonic hyperthermia process is highly desired because it potentially offers useful and comprehensive information regarding the entire tumor region and the status before or during the therapeutic process, including monitoring the therapeutic response and avoiding side-effects induced by external laser radiation to the surrounding healthy tissue. Due to the strong X-ray attenuation coefficient and high photothermal conversion efficiency, O-BiOCl nanosheets are expected to serve as dual-modal contrast agents for both CT and PA imaging of tumors. CT imaging is one of the most commonly used imaging modalities for clinical diagnoses because of its abilities such as deep tissue penetration and providing excellent high-resolution three-dimensional (3D) tomographic information of the anatomical structure. Bi—an element with a high atomic number—has already been explored for applications in contrast-enhanced CT imaging. Here, we initially accessed the X-ray attenuation ability of O-BiOCl-PVP nanosheets as CT contrast agents in vitro. The results exhibited that significantly enhanced Hounsfield unit (HU) values and correspondingly improved brightness of CT images were observed with elevated Bi concentrations (Fig. 6a and b), demonstrating that O-BiOCl-PVP nanosheets were promising contrast-enhanced CT imaging agents. Then, O-BiOCl-PVP nanosheets serving as in vivo contrast-enhanced CT imaging agents were evaluated on tumor-bearing BALB/c nude mice. After the intratumoral injection of O-BiOCl-PVP nanosheets, there was a pronounced CT contrast signal in the tumor region (Fig. 6c and d). The HU value induced by O-the BiOCl-PVP nanosheets after intratumoral injection increased from 71.6 to 113.3 a.u. These results demonstrated that O-BiOCl-PVP nanosheets could exhibit intriguing CT imaging capabilities.
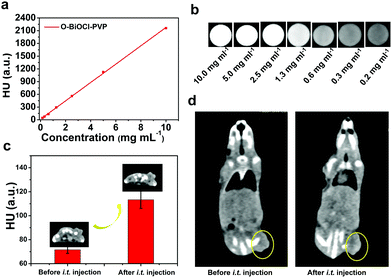 |
| Fig. 6 Contrast-enhanced CT imaging capability of O-BiOCl-PVP nanosheets both in vitro and in vivo. (a) In vitro CT contrasts and (b) CT images of O-BiOCl-PVP nanosheets solutions at increasing concentrations (0.2, 0.3, 0.6, 1.3, 2.5, 5.0, and 10.0 mg mL−1 with respect to Bi concentration). (c) In vivo CT contrasts of the tumor sites before and after intratumoral administration of O-BiOCl-PVP nanosheets. (d) In vivo 3D reconstruction of the CT images of mice before and after intratumoral administration of O-BiOCl-PVP nanosheets. | |
Furthermore, based on the high photothermal conversion efficiency, O-BiOCl-PVP nanosheets could also be used as contrast agents for PA imaging, which is an emerging imaging modality and affords deeper tissue-penetrating capabilities as compared to traditional optical imaging techniques. A combination of CT and PA imaging could offer real-time detection and whole-body imaging abilities with high spatial resolution of the whole tumor site without depth limitations. To examine their PA contrast performance in vitro and further investigate the relationship between the oxygen vacancy production and PA signal intensity, PA signals of the three representative O-BiOCl-PVP nanosheets (namely, OBP-1, OBP-2, and OBP-3) were recorded on a Vevo LAZR-X (FUJIFILM VisualSonics) instrument. The obtained results showed that the PA signals of OBP-1, OBP-2, and OBP-3 were concentration-dependent, and they were substantially enhanced with an increase in the Bi concentrations (Fig. 7a–f). It should be noted that as the amount of oxygen vacancies increased, the PA signal intensity correspondingly enhanced. The PA signals of OBP-3 were nearly 5.5 times higher than those of OBP-1 under the same concentrations (2.0 mg mL−1), indicating that the PA signals could be efficiently adjusted via precise control over oxygen vacancy generation. Then, these O-BiOCl-PVP nanosheets used as in vivo PA contrast agents were further investigated. 4T1-tumor-bearing mice were used as the in vivo animal model. After being intratumorally injected with O-BiOCl-PVP nanosheets, the mice were scanned by the PA instrument (Fig. 7g and h). The obtained images exhibited pronounced enhancement of the PA signals at the tumor sites: the PA values increased from 0.135 to 0.636 a.u. These results were in agreement with the CT results, which indicated that O-BiOCl-PVP nanosheets could act as efficient dual-modal contrast agents for tumor bioimaging.
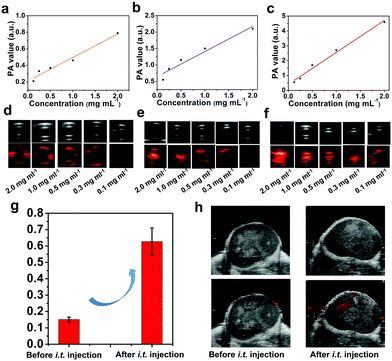 |
| Fig. 7 Contrast-enhanced PA imaging capabilities of O-BiOCl-PVP nanosheets both in vitro and in vivo. (a–c) In vitro–concentration-dependent PA signals and (d–f) corresponding PA imaging of the O-BiOCl-PVP nanosheet buffer solution for increasing concentrations (0.1, 0.3, 0.5, 1.0, and 2.0 mg mL−1). (g) In vivo PA signals and (h) PA imaging of 4T1-tumor-bearing mice after the administration of O-BiOCl-PVP nanosheets. | |
In vivo photothermal ablation of tumor by O-BiOCl-PVP nanosheets
Encouraged by the efficient in vitro photothermal ablation of cancer cells, the in vivo therapeutic efficiency of O-BiOCl-PVP nanosheets was systematically investigated. 4T1 breast tumor xenograft was established on mice. After the tumor volumes reached nearly 50 mm3, 20 4T1-tumor-bearing mice were randomly divided into 4 groups to accept different treatments (n = 5 per group): (i) control group, (ii) O-BiOCl-PVP group (intratumorally injected only with O-BiOCl-PVP nanosheets), (iii) NIR laser group, and (iv) O-BiOCl-PVP + NIR laser group (intratumorally injected with O-BiOCl-PVP nanosheets followed by 808 nm-laser irradiation). After the injection into the tumor sites, the NIR laser group and O-BiOCl-PVP + NIR laser group were subjected to 808 nm-laser irradiation at a power density of 1.5 W cm−2 for 10 min. The infrared (IR) thermal imaging instrument was utilized to monitor the surface temperature of the tumors. Fig. 8a shows the thermal images of the whole mice body at given time intervals during the treatment. Evidently, the surface temperature of the tumors in group (iv) increased up to 52 °C within 10 min, a temperature sufficient for tumor eradication, whereas the tumor surface temperature of the mice in group (iii) at the same power density only increased by 7 °C (Fig. 8b). After different instances, the mice weights and tumor volumes of the four groups were recorded and digital photos of the tumor sites were captured every 2 days for the subsequent 14 days. It should be noted that no obvious weight variation among these four groups (Fig. 8c) was observed. It was important to find that the tumor inhibition rate of the O-BiOCl-PVP-nanosheets-treated mice with 808 nm-laser irradiation could reach up to 89.3%, while the other three control groups exhibited a negligible inhibition effect on the tumor growth of mice (Fig. 8d and Fig. S12, ESI†), indicating that the O-BiOCl-PVP nanosheets with oxygen vacancies could efficiently mediate the photothermal property and induce hyperpyrexia for tumor ablation. The life span of the mice in the O-BiOCl-PVP + NIR laser group was significantly prolonged, and there were no deaths within 60 days. However, mice deaths were continuously observed in the other 3 groups after 25 days of treatments (Fig. 8e). These encouraging results demonstrated that O-BiOCl-PVP nanosheets with oxygen vacancies could efficiently achieve the photothermal inhibition of tumors.
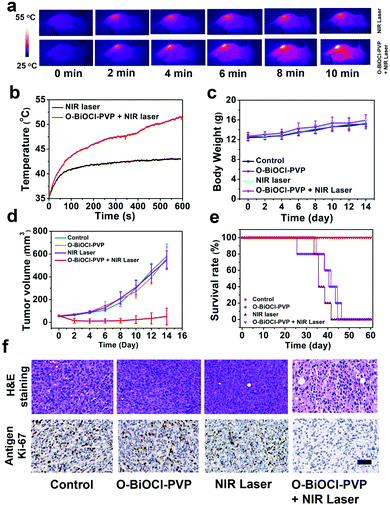 |
| Fig. 8
In vivo photothermal ablation efficiency against tumors when using O-BiOCl-PVP nanosheets. (a) IR thermal images of 4T1-tumor-bearing mice with or without the intratumoral injection of O-BiOCl-PVP nanosheets under 808 nm-laser irradiation at a power density of 1.5 W cm−2 at different time points. (b) Corresponding temperature elevations in the tumor regions of the 4T1-tumor-bearing mice under 808 nm-laser irradiation for 600 s. (c) Body weight curves of 4T1-tumor-bearing mice of four groups (control group, NIR laser group, O-BiOCl-PVP group, and O-BiOCl-PVP + NIR laser group) after receiving different treatments. (d) Tumor growth curves of the abovementioned four groups after receiving different treatments. (e) Survival rates of the 4T1-tumor-bearing mice within the tested period after different treatments (n = 5). (f) H&E staining and Ki-67 antibody immunofluorescence staining of the tumor tissues from each group after different treatments. All the scale bars are 50 μm. | |
Hematoxylin–eosin (H&E), TdT-mediated dUTP nick-end labeling (TUNEL), and Ki-67 antibody staining of the tumor slices from these four groups were conducted to obtain insights into the mechanism of the photothermal process. The tumor slices of the O-BiOCl-PVP + NIR laser group showed severe destruction of the tumor cells, including shrunk malignant cells and nucleus damage. Comparatively, the tumor specimens in the other three groups exhibited no differences regarding the size and shape, nuclear modifications, and cell necrosis. Ki-67 antibody staining was performed to study the proliferative activities of the cancer cells. The hyperthermia treatment in the O-BiOCl-PVP + NIR laser group led to markedly suppressed proliferative activities of cancer cells, whereas no appreciable change was observed in the suppression effect on cell proliferation in the other three groups, demonstrating that the O-BiOCl-PVP nanosheets with oxygen vacancies could efficiently regulate the high photothermal performance for successfully ablating the tumors (Fig. 8f and Fig. S13, ESI†).
It should be noted that the employed power density for the 808 nm laser is relatively high (2.0 W cm−2 for in vitro use and 1.5 W cm−2 for in vivo use). The employed laser power density is highly dependent on the photothermal agents’ accumulation at the tumor sites, NIR-light absorption, and photothermal convention efficiency of these agents. In this work, a relatively low dose of O-BiOCl-PVP nanosheets (10 mg kg−1, 50 μL) was used in vivo; therefore, a slightly higher laser power density was used to achieve a better tumor inhibition outcome. Both in vitro and in vivo results confirmed that the constructed O-BiOCl-PVP nanosheets with oxygen vacancies could efficiently achieve the photothermal inhibition of tumors, which also demonstrated the feasibility of this defect engineering strategy.
In vivo biocompatibility assay of O-BiOCl-PVP nanosheets
The in vivo biocompatibility of O-BiOCl-PVP nanosheets was systematically evaluated to guarantee their further clinical translation. Eighteen SBF-level healthy Kunming mice were randomly divided into 3 groups to receive different treatments (n = 6 per group), namely, (i) control, (ii) intravenously injected with O-BiOCl-PVP nanosheets at 10 mg kg−1, and (iii) intravenously injected with O-BiOCl-PVP nanosheets at 20 mg kg−1. After another 4 weeks of feeding, no obvious weight loss or abnormal behavior was observed in these 3 groups (Fig. S14, ESI†). The serum biochemistry assay and complete blood panel test were conducted. A series of blood indexes, namely, WBC, RBC, HGB, LYM, MCH, MCHC, MCV, MID, MPV, NEUT, PCT, PLT, HCT, CREA, BUN, ALT, AST, and ALP, were checked at predetermined time points post-injection. When compared with the control group, all the parameters in the other two O-BiOCl-PVP-nanosheets-treated groups appeared to be normal, indicating no significant inflammation and infection, as well as no obvious renal and hepatic toxicity in the treated mice (Fig. S15, ESI†). Then, these mice were sacrificed on the 30th day and their major organs (heart, lungs, liver, kidneys, and spleen) were dissected for further H&E histological analysis (Fig. S16, ESI†). The results of the H&E staining of the key organs from the examined tissue sections of different groups exhibited no shrunk malignant cells and/or nucleus damage on the basis of the size, shape, and nuclear modifications of the cells, indicating that no obvious lesions or histological abnormalities were induced in these main organs after the injection of O-BiOCl-PVP nanosheets at elevated doses (Fig. S16, ESI†). The preliminary in vivo data demonstrated that the constructed O-BiOCl-PVP nanosheets were biosafe and biocompatible to the mice at the tested dose within the feeding period (4 weeks).
Experimental section
Materials and reagents
D-Mannitol, Bi(NO3)3·5H2O, and potassium chloride (KCl) were obtained from Sigma-Aldrich (Shanghai, China). PVP was purchased from Shanghai Runcheng Bio-tech Co., Ltd.
Synthesis of white BiOCl nanosheets
Typically, D-mannitol (0.46 g) was dissolved in deionized water. Then, Bi(NO3)3·5H2O (0.486 g) and PVP (0.400 g) were added into the above solution. After 60 min of vigorous stirring, saturated KCl solution (5 mL) was added dropwise into the above mixture. After another 60 min of agitation, the mixture solution was transferred into a 100 mL Teflon-lined stainless steel autoclave, which was then heated up to 160 °C and maintained for 4 h. The obtained white products were collected by centrifugation and washed with deionized water for five times to remove any unwanted residual ions.
Synthesis of black O-BiOCl nanosheets
Black OB-1, OB-2, and OB-3 were produced by different UV-light irradiation durations (λ = 365 nm). In a typical procedure, pure white BiOCl nanosheets (0.125 g) were added into pure water (50 mL) under gentle stirring. When the irradiation time was 2 h, gray BiOCl nanosheets were formed (denoted as OB-1), whereas 6 h of irradiation time resulted in the formation of dark gray BiOCl nanosheets (denoted as OB-2). A further increase in the irradiation time (up to 12 h) resulted in the complete transformation into black BiOCl (denoted as OB-3).
Characterization
The TEM images and corresponding X-ray EDS data were obtained on a JEM-2100F electron microscope operated at 200 kV. The SEM images were acquired using a field-emission Magellan 400 microscope (FEI Company). AFM measurements were performed using a Veeco DI Nanoscope MultiMode V system. XPS data were recorded by ESCALAB 250 (Thermo Scientific). The UV-vis–NIR absorption spectra were recorded by means of a UV-3600 Shimadzu UV-vis–NIR spectrometer with QS-grade quartz cuvettes at room temperature. The size and zeta potential measurements were conducted on a Zetasizer Nano series instrument (Nano ZS90, Malvern Instruments Ltd). The CLSM images were measured by using an FV1000 instrument (Olympus Company, Japan). The O-BiOCl-PVP nanosheets concentration was determined by inductively coupled plasma atomic emission spectroscopy (Agilent Technologies, USA). The light was produced by a 20 W UV lamp.
ESR spectral test
TEMP (Dojindo Molecular Technologies, Inc.) was utilized to determine the generation of 1O2 induced by the BiOCl and O-BiOCl nanosheets. Typically, BiOCl nanosheets solutions and O-BiOCl nanosheets solutions (Bi: 400 μg mL−1) were exposed to 808 nm-laser irradiation at a power density of 2.0 W cm−2 for 300 s in the presence of TEMP (100 μM). Then, the 1O2 signal was immediately transferred into a quartz capillary and measured by using a Bruker EMX-8/2.7 spectrometer. In addition, an aqueous solution containing BiOCl nanosheets and O-BiOCl nanosheets (Bi: 400 μg mL−1) was mixed with DMPO (1 mM, Dojindo Molecular Technologies, Inc.), which was served as a spin trap agent for hydroxyl radicals (˙OH), followed by exposing to 808 nm-laser irradiation (2.0 W cm−2) for 300 s for ESR measurements.
In vitro photothermal performance of black O-BiOCl-PVP nanosheets
To evaluate the photothermal performance of black O-BiOCl-PVP nanosheets, 100 μL of an aqueous solution of BiOCl-PVP nanosheets with different Bi concentrations in 96-well plates was used for measurement and analysis by continuously irradiating with an 808 nm NIR laser (Shanghai Connect Fiber Optics Company) at power densities of 1.5 and 2.0 W cm−2 for 5 min. The temperatures at different time durations were recorded using the FLIRTM A325SC camera.
In vitro and in vivo CT imaging
For in vitro CT imaging, in order to detect the CT signals as a function of O-BiOCl-PVP nanosheets concentration, O-BiOCl-PVP nanosheets with different Bi concentrations (0.1, 0.3, 0.6, 1.3, 2.5, 5, and 10 mg mL−1) were scanned for in vitro CT imaging.
For in vivo CT imaging, 4T1-tumor-bearing nude mice were used as the animal model. After the tumor volume reached nearly 300 mm3, the mice were randomly separated into 2 groups (n = 3 per group), namely, the control group and O-BiOCl-PVP group. Then, the O-BiOCl-PVP nanosheets (10 mg kg−1, 30 μL) were intratumorally injected into the mice in the O-BiOCl-PVP group and the CT imaging of the tumor-bearing mice was performed before and after the intratumoral administration. The in vitro and in vivo CT images were collected using a GE discovery CT750 HD instrument (GE Healthcare). The parameters were as follows. Speed: 10.62 mm; slice thickness: 0.625 mm; beam pitch: 0.531
:
1; and gantry rotation time: 0.5 s.
In vitro and in vivo PA imaging
For in vitro PA imaging, in order to confirm the linearity of the PA signals introduced by the O-BiOCl-PVP nanosheets as a function of the O-BiOCl-PVP nanosheets concentration and further explore the relationship between the PA signal intensity and oxygen vacancies, O-BiOCl-PVP nanosheets with increasing concentrations (0.125, 0.25, 0.5, 1.0, and 2.0 mg mL−1) dispersed in deionized water were prepared for PA signal detection.
For in vivo PA imaging, 4T1-tumor-bearing nude mice were also selected as the animal model. After the tumor volume increased to nearly 150 mm3, the mice were randomly separated into 2 groups (n = 3 per group), namely, the control group and O-BiOCl-PVP group. Thereafter, the O-BiOCl-PVP nanosheets (10 mg kg−1, 50 μL) were intratumorally administered into 4T1-tumor-bearing mice of the O-BiOCl-PVP group and the PA imaging of these mice were taken before and after the injection of the O-BiOCl-PVP nanosheets using a Vevo LAZR-X PA imaging system (FUJIFILM VisualSonics).
In vitro cytotoxicity assay
Prior to the in vivo bioapplication of black O-BiOCl-PVP nanosheets, the cell viability was evaluated on murine breast cancer line (4T1 cancer cells) by the CCK-8 assay (Beyotime Institute of Biotechnology, Shanghai, China). Firstly, the 4T1 cancer cells were seeded in DMEM in 96-well plates at 37 °C for 24 h and allowed to adhere overnight. Then, the culture medium was replaced by adding fresh DMEM that contained black O-BiOCl-PVP nanosheets with different Bi concentrations ranging from 12.5 to 200 μg mL−1 in each well. After further incubation for 24 and 48 h, the cells were washed twice with PBS and the CCK-8 assay was used to evaluate the cell viabilities.
In vitro photothermal ablation of cancer cells
The in vitro cytotoxicity assay of O-BiOCl-PVP nanosheets irradiated by an 808 nm NIR laser was performed to access the in vitro PTT efficacy. 4T1 cancer cells were seeded in 96-well plates at 37 °C for 24 h. Then, the culture medium was discarded. Then, 100 μL of the O-BiOCl-PVP nanosheets solution with different Bi concentrations ranging from 0 to 240 μg mL−1 dispersed in DMEM was added into each well. After 4 h of incubation, the culture medium was replaced with fresh medium. After that, 4T1 cancer cells were irradiated by an 808 nm NIR laser at a power density of 2.0 W cm−2 for 5 min. After further incubation for 1 h, the cell viabilities were determined via the CCK-8 assay following the standard protocol.
In vivo photothermal cancer therapy by injection of O-BiOCl-PVP nanosheets
All the operations involving the animal experiments were conducted according to the guidelines authorized by the Administrative Committee of Laboratory Animals of Institute Pasteur of Shanghai, Chinese Academy of Sciences, China (approval no. A2018035). Twenty male BALB/c nude mice (4–5 weeks old) were subcutaneously inoculated with 4T1 cancer cells (1 × 107) suspended in PBS (100 μL). After the tumor volume reached ∼50 mm3, the mice were randomly separated into 4 groups (n = 5 per group), namely, (i) control group, (ii) O-BiOCl-PVP group (intratumorally injected with O-BiOCl-PVP nanosheets only), (iii) NIR laser group (exposed to 808 nm-laser irradiation only), and (iv) O-BiOCl-PVP + NIR laser group (intratumorally injected with O-BiOCl-PVP nanosheets followed by 808 nm-laser irradiation). Each mouse in the O-BiOCl-PVP group and O-BiOCl-PVP + NIR laser group was intratumorally injected with O-BiOCl-PVP nanosheets in PBS at a dose of 10 mg kg−1 (50 μL). After this intratumoral injection, the mice in the NIR laser group and O-BiOCl-PVP + NIR laser group were irradiated by the 808 nm NIR laser at a power density of 1.5 W cm−2 for 10 min. Three groups of mice (control, O-BiOCl-PVP, and NIR laser group) were set as the control groups. An IR thermal imaging instrument was utilized to measure the temperature at different time points. The tumor width (W) and length (L) were recorded every 2 days for 2 weeks and calculated as the volume according to the following formula: L × W × W/2. H&E, TUNEL, and Ki-67 antibody staining of the tumor slices from these 4 groups were collected at 24 h after different treatments for histological analysis.
In vivo toxicity assay
The in vivo toxicology of the black BiOCl-PVP nanosheets was systematically investigated. Eighteen healthy female Kunming mice (5 weeks old, ∼20 g) were randomly separated into 3 groups (n = 6), which were then subjected to the intravenous administration of black O-BiOCl-PVP nanosheets at increasing doses (0, 10, and 20 mg kg−1). The body weights of the mice were recorded every 2 days. Finally, the mice were euthanized on the 30th day after the intravenous administration, and the blood samples were collected to conduct a complete blood panel test, namely, white blood cells (WBC), red blood cells (RBC), hemoglobin (HGB), lymphocyte (LYM), mean corpuscular hemoglobin (MCH), mean corpuscular hemoglobin concentration (MCHC), mean corpuscular volume (MCV), intermediate cell absolute value (MID), mean platelet volume (MPV), neutrophil (NEUT), plateletcrit (PCT), platelets (PLT), hematocrit (HCT), creatinine (CREA), blood urea nitrogen (BUN), alanine aminotransferase (ALT), aspartate aminotransferase (AST), and alkaline phosphatase (ALP). Then, we collected the major organs of the mice (heart, lungs, liver, kidneys, and spleen), fixing them in 10% formalin and stained with H&E for further histological analysis.
Conclusions
In summary, 2D oxygen-vacancy-engineered BiOCl nanosheets were constructed for efficient dual-modal CT-/PA-imaging-guided photonic tumor ablation. In particular, a specific defect engineering strategy was developed by the application of UV-light irradiation to create oxygen vacancies on the surface of BiOCl nanosheets. Engineering crystal defects on the surface of BiOCl nanosheets substantially enhanced their optical absorption ability and photothermal performance, which could be used for both PA imaging and hyperthermia cancer ablation. Importantly, the PA imaging capability and photothermal conversion efficiency of these BiOCl nanosheets could be precisely modulated by tuning the amounts of oxygen vacancies. Furthermore, the strong Bi-based X-ray attenuation coefficient enabled them with the capability for contrast-enhanced CT imaging. After PVP surface engineering, the in vitro and in vivo biosafety of these BiOCl nanosheets was systematically evaluated, which guaranteed their further medical applications. This work not only provides a desirable theranostic agent for tumor nanomedicine, but also proposes a feasible and efficient methodology to precisely tune the PA ability and photothermal efficiency of nanoagents by defect engineering.
Conflicts of interest
The authors declare no conflicts of interest.
Acknowledgements
The authors greatly acknowledge the financial support from National Natural Science Foundation of China (Grant No. 81401428, 81571693, 81871361, 81871347 and 81670067), Shanghai East Hospital “leading talent project” (DFRC2018024), Pudong Health Bureau of Shanghai (Grant No. PWRl2018-06), Shanghai Anticancer Association EYAS PROJECT (Grant No. SACA-CY1B10) and Shanghai Sailing Program (Grant No. 19YF1410100 and 19YF1453700).
Notes and references
- M. Ferrari, Nat. Rev. Cancer, 2005, 5, 161–171 CrossRef CAS PubMed.
- Z. Shen, J. Song, B. C. Yung, Z. Zhou, A. Wu and X. Chen, Adv. Mater., 2018, 30, e1704007 CrossRef PubMed.
- Q. Miao and K. Pu, Adv. Mater., 2018, 30, 1801778 CrossRef PubMed.
- Q. Chen, L. Xu, C. Liang, C. Wang, R. Peng and Z. Liu, Nat. Commun., 2016, 7, 13193 CrossRef CAS PubMed.
- X. Ai, C. J. Ho, J. Aw, A. B. Attia, J. Mu, Y. Wang, X. Wang, Y. Wang, X. Liu, H. Chen, M. Gao, X. Chen, E. K. Yeow, G. Liu, M. Olivo and B. Xing, Nat. Commun., 2016, 7, 10432 CrossRef CAS PubMed.
- Z. Zhou, J. Song, L. Nie and X. Chen, Chem. Soc. Rev., 2016, 45, 6597–6626 RSC.
- G. Chen, H. Qiu, P. N. Prasad and X. Chen, Chem. Rev., 2014, 114, 5161–5214 CrossRef CAS PubMed.
- J. A. Barreto, W. O'Malley, M. Kubeil, B. Graham, H. Stephan and L. Spiccia, Adv. Mater., 2011, 23, H18–H40 CrossRef CAS PubMed.
- B. Pelaz, C. Alexiou, R. A. Alvarez-Puebla, F. Alves, A. M. Andrews, S. Ashraf, L. P. Balogh, L. Ballerini, A. Bestetti, C. Brendel, S. Bosi, M. Carril, W. C. Chan, C. Chen, X. Chen, X. Chen, Z. Cheng, D. Cui, J. Du, C. Dullin, A. Escudero, N. Feliu, M. Gao, M. George, Y. Gogotsi, A. Grunweller, Z. Gu, N. J. Halas, N. Hampp, R. K. Hartmann, M. C. Hersam, P. Hunziker, J. Jian, X. Jiang, P. Jungebluth, P. Kadhiresan, K. Kataoka, A. Khademhosseini, J. Kopecek, N. A. Kotov, H. F. Krug, D. S. Lee, C. M. Lehr, K. W. Leong, X. J. Liang, M. Ling Lim, L. M. Liz-Marzan, X. Ma, P. Macchiarini, H. Meng, H. Mohwald, P. Mulvaney, A. E. Nel, S. Nie, P. Nordlander, T. Okano, J. Oliveira, T. H. Park, R. M. Penner, M. Prato, V. Puntes, V. M. Rotello, A. Samarakoon, R. E. Schaak, Y. Shen, S. Sjoqvist, A. G. Skirtach, M. G. Soliman, M. M. Stevens, H. W. Sung, B. Z. Tang, R. Tietze, B. N. Udugama, J. S. VanEpps, T. Weil, P. S. Weiss, I. Willner, Y. Wu, L. Yang, Z. Yue, Q. Zhang, Q. Zhang, X. E. Zhang, Y. Zhao, X. Zhou and W. J. Parak, ACS Nano, 2017, 11, 2313–2381 CrossRef CAS PubMed.
- Z. Dong, Z. Yang, Y. Hao and L. Feng, Nanoscale, 2019, 11, 16164–16186 RSC.
- Y. Hao, Z. Dong, M. Chen, Y. Chao, Z. Liu, L. Feng, Y. Hao, Z. L. Dong, M. C. Chen, Y. Chao, Z. Liu and L. Z. Feng, Biomaterials, 2020, 228, 119568 CrossRef PubMed.
- X. Zhen, C. Xie and K. Pu, Angew. Chem., Int. Ed., 2018, 57, 3938–3942 CrossRef CAS PubMed.
- J. Li, C. Xie, J. Huang, Y. Jiang, Q. Miao and K. Pu, Angew. Chem., Int. Ed., 2018, 57, 3995–3998 CrossRef CAS PubMed.
- G. Song, C. Liang, X. Yi, Q. Zhao, L. Cheng, K. Yang and Z. Liu, Adv. Mater., 2016, 28, 2716–2723 CrossRef CAS PubMed.
- C. Dai, Y. Chen, X. Jing, L. Xiang, D. Yang, H. Lin, Z. Liu, X. Han and R. Wu, ACS Nano, 2017, 11, 12696–12712 CrossRef CAS PubMed.
- M. S. Yavuz, Y. Cheng, J. Chen, C. M. Cobley, Q. Zhang, M. Rycenga, J. Xie, C. Kim, K. H. Song, A. G. Schwartz, L. V. Wang and Y. Xia, Nat. Mater., 2009, 8, 935–939 CrossRef CAS PubMed.
- H. Liu, T. Liu, X. Wu, L. Li, L. Tan, D. Chen and F. Tang, Adv. Mater., 2012, 24, 755–761 CrossRef CAS PubMed.
- J. Kim, S. Park, J. E. Lee, S. M. Jin, J. H. Lee, I. S. Lee, I. Yang, J.-S. Kim, S. K. Kim, M.-H. Cho and T. Hyeon, Angew. Chem., Int. Ed., 2006, 118, 7918–7922 CrossRef.
- J. Conde, N. Oliva, Y. Zhang and N. Artzi, Nat. Mater., 2016, 15, 1128–1138 CrossRef CAS PubMed.
- L. Cheng, J. Liu, X. Gu, H. Gong, X. Shi, T. Liu, C. Wang, X. Wang, G. Liu, H. Xing, W. Bu, B. Sun and Z. Liu, Adv. Mater., 2014, 26, 1886–1893 CrossRef CAS PubMed.
- L. Cheng, W. Huang, Q. Gong, C. Liu, Z. Liu, Y. Li and H. Dai, Angew. Chem., Int. Ed., 2014, 53, 7860–7863 CrossRef CAS PubMed.
- X. Zhu, W. Feng, J. Chang, Y. W. Tan, J. Li, M. Chen, Y. Sun and F. Li, Nat. Commun., 2016, 7, 10437 CrossRef CAS PubMed.
- K. Yang, L. Feng, X. Shi and Z. Liu, Chem. Soc. Rev., 2013, 42, 530–547 RSC.
- K. Yang, S. Zhang, G. Zhang, X. Sun, S. T. Lee and Z. Liu, Nano Lett., 2010, 10, 3318–3323 CrossRef CAS PubMed.
- L. Lin, L. Liu, B. Zhao, R. Xie, W. Lin, H. Li, Y. Li, M. Shi, Y. G. Chen, T. A. Springer and X. Chen, Nat. Nanotechnol., 2015, 10, 465–471 CrossRef CAS PubMed.
- Z. B. Zha, X. L. Yue, Q. S. Ren and Z. F. Dai, Adv. Mater., 2013, 25, 777–782 CrossRef CAS PubMed.
- M. Y. Liu, G. J. Zeng, K. Wang, Q. Wan, L. Tao, X. Y. Zhang and Y. Wei, Nanoscale, 2016, 8, 16819–16840 RSC.
- S.-W. Liu, L. Wang, M. Lin, Y. Liu, L.-N. Zhang and H. Zhang, Chin. J. Polym. Sci., 2018, 37, 115–128 CrossRef.
- F. Gong, L. Cheng, N. Yang, Q. Jin, L. Tian, M. Wang, Y. Li and Z. Liu, Nano Lett., 2018, 18, 6037–6044 CrossRef CAS PubMed.
- P. K. Jain, K. S. Lee, I. H. El-Sayed and M. A. El-Sayed, J. Phys. Chem. B, 2006, 110, 7238–7248 CrossRef CAS PubMed.
- W. Zhou, X. Gao, D. Liu and X. Chen, Chem. Rev., 2015, 115, 10575–10636 CrossRef CAS PubMed.
- R. Vankayala, C. C. Lin, P. Kalluru, C. S. Chiang and K. C. Hwang, Biomaterials, 2014, 35, 5527–5538 CrossRef CAS PubMed.
- J. Shao, H. Xie, H. Huang, Z. Li, Z. Sun, Y. Xu, Q. Xiao, X. F. Yu, Y. Zhao, H. Zhang, H. Wang and P. K. Chu, Nat. Commun., 2016, 7, 12967 CrossRef CAS PubMed.
- X. X. Han, J. Huang, X. X. Jing, D. Y. Yang, H. Lin, Z. G. Wang, P. Li and Y. Chen, ACS Nano, 2018, 12, 4545–4555 CrossRef CAS PubMed.
- X. B. Chen, L. Liu, P. Y. Yu and S. S. Mao, Science, 2011, 331, 746–750 CrossRef CAS PubMed.
- W. Guo, F. Wang, D. Ding, C. Song, C. Guo and S. Liu, Chem. Mater., 2017, 29, 9262–9274 CrossRef CAS.
- K. S. Novoselov, Nature, 2014, 505, 291 CrossRef CAS PubMed.
- M. Yankowitz, J. Jung, E. Laksono, N. Leconte, B. L. Chittari, K. Watanabe, T. Taniguchi, S. Adam, D. Graf and C. R. Dean, Nature, 2018, 557, 404 CrossRef CAS PubMed.
- M. Naguib, V. N. Mochalin, M. W. Barsoum and Y. Gogotsi, Adv. Mater., 2014, 26, 992–1005 CrossRef CAS PubMed.
- M. R. Lukatskaya, O. Mashtalir, C. E. Ren, Y. Dall'Agnese, P. Rozier, P. L. Taberna, M. Naguib, P. Simon, M. W. Barsoum and Y. Gogotsi, Science, 2013, 341, 1502–1505 CrossRef CAS PubMed.
- S. S. Chou, B. Kaehr, J. Kim, B. M. Foley, M. De, P. E. Hopkins, J. Huang, C. J. Brinker and V. P. Dravid, Angew. Chem., Int. Ed., 2013, 52, 4160–4164 CrossRef CAS PubMed.
- S. Wang, X. Li, Y. Chen, X. Cai, H. Yao, W. Gao, Y. Zheng, X. An, J. Shi and H. Chen, Adv. Mater., 2015, 27, 2775–2782 CrossRef CAS PubMed.
- X. Huang, S. Tang, X. Mu, Y. Dai, G. Chen, Z. Zhou, F. Ruan, Z. Yang and N. Zheng, Nat. Nanotechnol., 2011, 6, 28–32 CrossRef CAS PubMed.
- H. Lin, Y. Wang, S. Gao, Y. Chen and J. Shi, Adv. Mater., 2018, 30, 1703284 CrossRef PubMed.
- H. Lin, X. Wang, L. Yu, Y. Chen and J. Shi, Nano Lett., 2017, 17, 384–391 CrossRef CAS PubMed.
- B. Yang, J. Yin, Y. Chen, S. Pan, H. Yao, Y. Gao and J. Shi, Adv. Mater., 2018, 30, 1705611 CrossRef PubMed.
- S. J. Wu, W. W. Sun, J. G. Sun, Z. D. Hood, S. Z. Yang, L. D. Sun, P. R. C. Kent and M. F. Chisholm, Chem. Mater., 2018, 30, 5128–5136 CrossRef CAS.
- W. Ouyang, L. Su and X. Fang, Small, 2018, 14, e1801611 CrossRef PubMed.
- L. Zhang, Z. Han, W. Wang, X. Li, Y. Su, D. Jiang, X. Lei and S. Sun, Chemistry, 2015, 21, 18089–18094 CrossRef CAS PubMed.
- M. Guan, C. Xiao, J. Zhang, S. Fan, R. An, Q. Cheng, J. Xie, M. Zhou, B. Ye and Y. Xie, J. Am. Chem. Soc., 2013, 135, 10411–10417 CrossRef CAS PubMed.
- L. Ye, K. Deng, F. Xu, L. Tian, T. Peng and L. Zan, Phys. Chem. Chem. Phys., 2012, 14, 82–85 RSC.
- K. Zhao, L. Zhang, J. Wang, Q. Li, W. He and J. J. Yin, J. Am. Chem. Soc., 2013, 135, 15750–15753 CrossRef CAS PubMed.
- Y. Xu, Z. Shi, L. Zhang, E. M. Brown and A. Wu, Nanoscale, 2016, 8, 12715–12722 RSC.
- L. Ye, X. Jin, Y. Leng, Y. Su, H. Xie and C. Liu, J. Power Sources, 2015, 293, 409–415 CrossRef CAS.
- L. Zhang, W. Wang, D. Jiang, E. Gao and S. Sun, Nano Res., 2014, 8, 821–831 CrossRef.
- L. Ye, L. Zan, L. Tian, T. Peng and J. Zhang, Chem. Commun., 2011, 47, 6951–6953 RSC.
- D. Cui, L. Wang, K. Xu, L. Ren, L. Wang, Y. Yu, Y. Du and W. Hao, J. Mater. Chem. A, 2018, 6, 2193–2199 RSC.
- B. Sarwan, B. Pare and A. D. Acharya, Mater. Sci. Semicond. Process, 2014, 25, 89–97 CrossRef CAS.
- J. T. Robinson, S. M. Tabakman, Y. Liang, H. Wang, H. S. Casalongue, D. Vinh and H. Dai, J. Am. Chem. Soc., 2011, 133, 6825–6831 CrossRef CAS PubMed.
- C. Ma, E. Rangasamy, C. D. Liang, J. Sakamoto, K. L. More and M. F. Chi, Angew. Chem., Int. Ed., 2015, 54, 129–133 CrossRef CAS PubMed.
Footnotes |
† Electronic supplementary information (ESI) available. See DOI: 10.1039/c9nh00707e |
‡ These authors contributed equally to this work. |
|
This journal is © The Royal Society of Chemistry 2020 |