DOI:
10.1039/C9NH00490D
(Communication)
Nanoscale Horiz., 2020,
5, 350-358
ROS self-generation and hypoxia self-enhanced biodegradable magnetic nanotheranostics for targeted tumor therapy†
Received
24th July 2019
, Accepted 2nd October 2019
First published on 3rd October 2019
Abstract
Amelioration of solid tumor hypoxia is one of the promising therapeutic strategies for malignant tumor ablation. In particular, reactive oxygen species (ROS)-induced apoptosis has been proved to be an efficient tumor therapeutic approach, relying on advanced drug delivery or rapid production of ROS in the tumor region. However, the ROS generation is severely limited by tumor hypoxia. Herein, a new nanostructure based on biodegradable mesoporous magnetic nanocubes (MMNCs) is designed for ROS self-generation and self-enhancement magnetic thermotherapy. Typically, vitamin c (Vc) locked in MMNCs with phase-change materials (PCM) could be triggered by hyperthermia under an alternating magnetic field (AMF), serving as an original source for ROS (H2O2) generation. In addition, the MMNCs possess Fenton reagent-like activity in an acidic environment for ROS self-enhancement, which causes severe damage to tumor cells. The multifunctional nanotheranostics exhibits remarkable hyperthermia and enhanced ROS synergistic therapy efficiency. Furthermore, the nanoplatform demonstrates good biodegradability under acidic tumor microenvironment conditions. This finding highlights the great potential of Vc loaded magnetic nanoparticle composites as efficient agents for tumor therapy. It opens an avenue for the design of nanoformulations with ROS self-generation and self-enhancement for overcoming tumor hypoxia.
New concepts
We report a novel ROS self-generation and ROS self-enhancement anti-tumor nanotheranostics based on Vitamin c (Vc) loaded biodegradable mesoporous magnetic nanocubes (MMNCs). Tumor hypoxia amelioration is one of the promising therapeutic strategies for tumor ablation. Vc has been proved to be a pro-oxidant for preferential formation of ROS (H2O2) in tumor cells and causes selective toxicity to tumor cells rather than normal cells. In our ingenious design, Vc locked in MMNCs with phase-change materials (PCM) could be triggered by hyperthermia under an alternating magnetic field (AMF), thus serving as an original source for ROS self-generation. The MMNCs possess Fenton reagent-like activity in the acidic environment for ROS self-enhancement, and cause more severe damage to tumor cells. Furthermore, our therapeutic nanoplatform shows good biodegradability since most iron nanomaterials interacting with cells would vanish gradually in the mild acidic tumor microenvironment in vivo. Our work presents an ingenious delivery system design strategy to fabricate hypoxia overcoming nanoagents as demonstrated herein, not only potentially useful for developing biodegradable functional nanostructures with low side effects, but also paving the way for advancing a ROS self-generation and self-enhancement cancer therapeutic approach in overcoming tumor hypoxia.
|
1. Introduction
Human solid tumors present diverse heterogeneous microenvironmental characteristics, including acidic pH, high interstitial fluid pressure, high permeability vessels, and inflammatory reactions.1–3 Hypoxia (low O2 level) is an essential pathophysiological signal for most malignant tumors.4–6 Intratumoral hypoxia generally causes phenotypic diversities like O2-sensitive pathways, histological grade, or lymph node status, which would significantly impact the responses to chemo- or radio-therapy, neoplasm metastasis, cancer recurrence and even survival.7,8 Nevertheless, hypoxia also poses an opportunity for tumor therapeutics by enhancing intralesional oxygenation so as to reverse the hypoxia-associated resistance.9 Recently, multiple strategies have been developed to overcome tumor hypoxia by delivering oxygen into tumors, generating oxygen in situ and producing lethal ROS at tumor lesions, thus causing intense oxidative damage to cells and destructing the tumor tissue.10–13 In particular, photodynamic therapy (PDT), sonodynamic therapy (SDT) and radiodynamic therapy (RDT) are the common treatments for efficient ROS tumor therapy.14–16 Unfortunately, most of these strategies have inherent limitation for ROS generation, which largely depends on external oxygen delivery.17 The low specific delivery of oxygen into hypoxic tumors or high systemic toxicity of most oxygen delivery carriers limits their further application as tumor theranostics. Meanwhile, a previous study has shown that an adequate intracellular oxygen concentration is vital for sufficient ROS generation.18 The intratumoral oxygen concentration in a tumor is at a quite low level (pO2 ≤ 8.0 mmHg), since the blood supply cannot sustain the invasive tumor growth.19 Therefore, it is essentially important to exploit ROS self-generation substances or nanoplatforms for efficient ROS tumor therapy.
Vitamin C (Vc), a well-known reducing antioxidant agent, naturally exists in biology.20 However, pharmacologic Vc has been proved to be a pro-oxidant for preferential formation of ascorbate radicals (Asc˙) and H2O2 in tumor cells and causes selective toxicity to tumor cells rather than normal cells.21–24 In normal cells, the formed H2O2 will be immediately eliminated by plasma catalase and GSH peroxidase.25 Previous studies have revealed that only high intracellular Vc concentration (>3.5 pM per cell) would cause obvious tumor inhibition.26 To this end, sufficient Vc should be transported into tumor cells by ideal drug delivery approaches for ROS therapy.
Fortunately, nanomaterials with hollow or porous structures are suitable for delivering cargoes because of their open spaces and high surface areas.27–30 Through smart design and chemical modification, the engineered nanoplatforms can be endowed with multifunctions like targeted delivery, large loading capacity, and controlled release properties.31,32 A nano drug vehicle with special characteristics also has the potential for enhanced radiotherapy or photothermal treatment to optimize the synergistic therapeutic effect.33,34 Moreover, it has been reported that the tumor hypoxia status could be significantly relieved by increasing the intratumoral blood flow via a simple mild hyperthermia in the tumor region.35–37 Consequently, nanomaterials with preferable thermogenesis ability are appropriate as ROS drug delivery systems and hypoxia specific nanotheranostics for tumor therapy.
With this in mind, a magnetic stimuli-responsive drug cargo based on Vc loaded MMNCs is designed for ROS and hyperthermia synergistic tumor therapy. Typically, Vc is locked in the MMNCs by a temperature-responsive PCM and designed as the ROS head-stream. In this study, 1-tetradecanol, a biocompatible PCM with an exquisite phase-change temperature around 38 °C approved by FDA,38–40 was utilized as the terminal switch for the hypoxia-specific temperature-responsive drug cargo (MMNCs@PCM@Vc). Interestingly, the MMNCs@PCM@Vc nanoplatform is in the solid state under physiological conditions (about 37 °C) that could achieve “zero release” behavior for leakage-free drug delivery in vivo. The packaged Vc in MMNCs@PCM@Vc would not be releases until the PCM changes into the liquid phase (>38 °C). After this, the nanoagent could be activated by an external high-frequency alternating magnetic field (AMF), since the magnetic nanomaterials could trigger thermogenesis in an operational AMF. When the temperature exceeds 38 °C, the Vc will be released from MMNCs to selectively produce H2O2 at the tumor lesion. Then the bare mesoporous MMNCs provide sufficient surface iron sites for the subsequent Fenton reaction, reducing the negative impact of surface modification for Fenton catalysis.
Meanwhile, transition metal nanoparticles have been proved to be intrinsic peroxidase-mimics,24,41–43 which not only catalyze the H2O2 to produce O2 but also mediate the oxidation of H2O2 to hydroxyl radicals (˙OH) via a typical Fenton reaction in the presence of ferrous ions.44–46 Moreover, the generated ˙OH is a superior ROS source for H2O2 in most tumor cells, leading to higher intracellular cytotoxicity. On the other hand, the magnetic nanotheranostics could be adopted as a T2 contrast agent for magnetic resonance imaging (MRI) by magnifying the longitudinal relaxation of water-proton.47–49 Simultaneously, most iron nanomaterials interacting with cells would vanish gradually, due to the combined effect of mild acidic pH (around 5.0), lysosomal enzymes, and iron regulation.50,51 It is generally assumed that the biodegradation of MMNCs could take place in vivo for potential clinical application. Thus, we hypothesize that MMNCs@PCM@Vc could release sufficient Vc under an AMF and generate H2O2in situ, and then function as an oxidase to catalytically convert H2O2 to ˙OH, enhancing the ROS level and overcoming the intracellular hypoxia.52,53 The magnetocaloric effect of the MMNCs in the AMF could not only trigger the temperature-responsive drug delivery system but also realize the anti-tumor efficiency by hyperthermia and ROS-enhanced oncotherapy. The fabrication procedure of MMNCs@PCM@Vc and its systemic hypoxia anti-tumor effect are illustrated in Scheme 1.
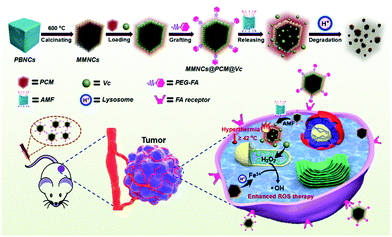 |
| Scheme 1 Diagram showing the fabrication procedure, ROS self-generation and self-enhancement process of the MMNCs@PCM@Vc nanocubes. | |
2. Results and discussion
2.1. Characterization
Firstly, Prussian Blue nanocubes (PBNCs) were synthesized according to a previous study54 and used as the precursors to prepare Fe2O3 mesoporous magnetic nanocubes (MMNCs). Then, the MMNCs were loaded with Vc via a PCM and modified with PEG-FA to prepare MMNCs@PCM@Vc (Scheme S1, ESI†). The morphologies of different as-synthesized nanocubes were revealed by scanning electron microscopy (SEM) and transmission electron microscopy (TEM). As shown in Fig. 1a–i and Fig. S1a–c (ESI†), the as-fabricated PBNCs displayed relatively high dispersity and uniform morphology with an average diameter of 147.6 nm (mean ± SD, n = 400). After calcination, the obtained MMNCs showed mesoporous structures with an average diameter of 142.3 nm. Then Vc was loaded into the MMNCs with the PCM. The MMNCs@PCM@Vc presented an increased diameter of about 154.4 nm. Fig. S1d and e (ESI†) show the HRTEM image and SAED pattern of an individual as-prepared PBNC. Typically, an interplanar spacing of about 0.506 nm was observed and attributed to the (200) plane of Prussian Blue (PB) nanoparticles, suggesting the single-crystalline structure of PBNCs. As for MMNCs, the corresponding HRTEM image (Fig. S1f, ESI†) shows crystalline characteristics with lattice spacings of 0.252 nm and 0.295 nm, which were indexed to the (311) and (220) planes of cubic γ-Fe2O3. Meanwhile, the rings of SAED patterns (Fig. S1g, ESI†) were well indexed for MMNCs. The crystallographic orientations were observed due to the crystalline structure of γ-Fe2O3. These results were also consistent with XRD analysis (Fig. S2, ESI†).
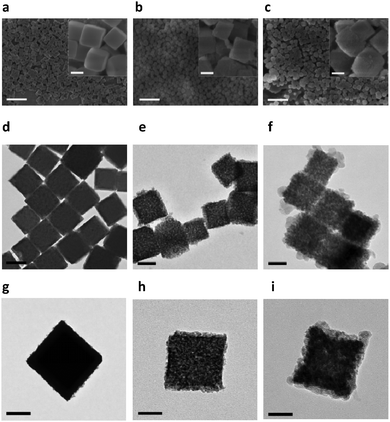 |
| Fig. 1 Characterization of the obtained nanoparticles. SEM images of (a) PBNCs, (b) MMNCs and (c) MMNCs@PCM@Vc, scale bar: 1 μm, inset scale bar: 100 nm. TEM images of (d) PBNCs, (e) MMNCs and (f) MMNCs@PCM@Vc, scale bar: 100 nm. Individual particle TEM images of (g) PBNCs, (h) MMNCs and (i) MMNCs@PCM@Vc, scale bar: 50 nm. | |
Usually, it is difficult to distinguish γ-Fe2O3 from Fe3O4 by XRD measurements because of their similar structures. The Raman technique has been proved to be valuable in verifying different iron oxide phases, especially between Fe3O4 and γ-Fe2O3, which cannot be effectively separated by the XRD spectrum.55 Typically, a strong peak at 665 cm−1 and two weak peaks at 340 and 527 cm−1 can be assigned to the A1g, T2g and Eg modes of Fe3O4, respectively. These peaks over the wavelength ranges of 200–400 and 600–800 cm−1 are characteristic peaks of γ-Fe2O3, which is consistent with pervious reports (Fig. S3, ESI†).
The magnetization values of the obtained MMNCs and MMNCs@PCM@Vc are 30.7 and 25.0 emu g−1, respectively (Fig. 2a). Such high magnetization of the MMNCs@PCM@Vc nanoagents offers great potential for magnetic targeting and hyperthermia since the nanoparticles could instantly respond to the external magnetic field. The porous nature and specific surface area of the as-prepared nanocubes were further investigated by N2 adsorption–desorption measurements. Fig. 2b and Fig. S4 (ESI†) reveal the isotherm properties and corresponding Barrett–Joyner–Halenda (BJH) pore size distribution curves. For the MMNCs, the BET specific surface area of the sample is about 86.2 m2 g−1 and the average pore diameter is 2.0–8.0 nm according to the BJH plots. As for MMNCs@PCM@Vc, the BET surface area and total pore diameter decreased significantly after loading with the PCM and Vc. The surface charge of the nanoparticles was monitored with a zeta-potentiostat (Fig. S5, ESI†). As for the positive charge of HOOC-PEG-FA, it was contributed by two factors: first, the purchased precursor molecule of HOOC-PEG-NH2 is highly positively charged; second, only a small amount of FA was conjugated to the precursor molecule, leading to only a slightly lower positive charge. The MMNCs@PCM@Vc exhibited a moderate positive charge (about 6.3 mV), which is beneficial for cellular cytophagy. X-ray photoelectron spectroscopy (XPS) analysis was further employed to reveal the purity and chemical displacement during the fabrication process. As shown in Fig. 2c, peaks at 268 and 531 eV indicate the characteristic peaks of C 1s and O 1s, respectively. For native PBNCs, the peak at 397 eV is ascribed to the characteristic peak of N 1s. Further, this N 1s peak was not observed in MMNCs and MMNCs@PCM@Vc samples, attributing to the calcination during Fe2O3 preparation. In all samples, the binding energy peaks around 711 and 724 eV were attributed to Fe 2p3/2 and Fe 2p1/2, respectively.
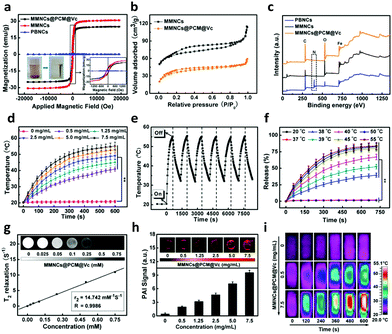 |
| Fig. 2 Characterization of the obtained nanoparticles. (a) Magnetic hysteresis loops of the as-prepared PBNCs, MMNCs and MMNCs@PCM@Vc. (b) N2 adsorption–desorption isotherms for MMNCs and MMNCs@PCM@Vc. (c) XPS spectra of the as-prepared nanoparticles. (d) The concentration-dependent thermogenesis of MMNCs@PCM@Vc in PBS subjected to an AMF (4 kW, 300 A and 200 kHz). (e) Cycle heating performance of the MMNCs@PCM@Vc (7.5 mg mL−1) over 6 on/off cycles. (f) Dug release profile of MMNCs@PCM@Vc at different temperatures. MRI (g), PAI (h) and ITI (i) performance of the obtained MMNCs@PCM@Vc in vitro. | |
To investigate the magnetic thermogenesis of MMNCs@PCM@Vc for antitumor therapy, different concentrations of MMNCs@PCM@Vc were dissolved in PBS and subjected to an AMF (4 kW, 300 A and 200 kHz) for 10 min. The temperature of the system could ultimately reach above 50 °C with a concentration of 5.0 mg mL−1 (Fig. 2d), however, in a concentration-dependent calorigenic manner. Meanwhile, the nanoagents were dispersed into different media for evaluating their stability (Fig. S6, ESI†). MMNCs@PCM@Vc exhibited high stability and slow aggregation in all kinds of media. Moreover, the thermogenic capacity of the nanoagent exhibited no significant difference in diverse solvents. In addition, the MMNCs@PCM@Vc also displayed good thermogenic stability in the AMF during the 6 “on–off” cycles (Fig. 2e), indicating that the nanocubes had good thermogenesis properties for further application. A PCM is an amphipathic molecule, having a hydrophilic head and a long hydrophobic tail, which makes it capable of encapsulating either hydrophilic or hydrophobic substances. The thermal responsive release profiles of the MMNCs@PCM system were measured as proof by using two dyes with different hydrophilicities. Herein, hydrophilic methylene blue (MB) and hydrophobic Rhodamine 6G (R6G) were adopted as model drugs and then quantified by UV-spectrophotometry. As presented in Fig. S7 (ESI†), both model drugs were loaded in the MMNCs@PCM system and achieved a “on–off” pulsing release profile by just simply switching on or off the applied AMF (300 A, 200 kHz, 4 kW). However, the MMNCs@PCM system did not release any drugs under a static magnetic field (SMF, 0.3 T).56 The pulsatile drug release behavior under the AMF also has interesting implications for other clinical applications such as diabetes treatment. Interestingly, the as synthesized system displayed a strict temperature-dependent drug release profile; over 60% of the drug was released in 10 min at 40 °C, while a negligible amount of drug was released (<5%) at 20 °C or 37 °C (Fig. 2f). It reveals that the designed nanocarriers exhibited near “zero release” to reduce the drug leakage before they reached the tumor lesion. In addition, the long-term drug release behavior was further detected for 30 days (Fig. S8, ESI†). The result manifests that there was a small amount of drug leakage, indicating their good stability below the phase transition temperature.
With the advancement of nanotheranostic technology, nanocarriers simultaneously provide great convenience for a multimodality imaging-guided cancer therapy strategy. The as-prepared iron nanocubes have the potential for magnetic resonance imaging (MRI) by reducing transverse relaxation times of protons because of the magnetic inner core. Fig. 2g shows the transverse relaxation rates (1/T2) in a linear dependence on the concentration of the obtained MMNCs@PCM@Vc. The transverse relaxivity (r2) was determined as 14.7 mM−1 S−1, indicating its potential to be an efficient T2-weighted MRI contrast agent in vivo. The as-synthesized nanocubes could serve as a photoacoustic imaging (PAI) agent due to the magnetocaloric effect. As shown in Fig. 2h, the PAI signals show concentration-dependent performance from 0 to 7.5 mg mL−1. In addition, the increasing temperature signals can be captured and reconstituted into infrared thermographic images (ITI, Fig. 2i).
2.2. Cell uptake and intracellular ROS self-generating and self-enhancing ability of MMNCs@PCM@Vc
To further explore the endocytosis of the nanocubes, the uptake of the MMNCs@PCM@Vc was detected with HeLa cells. As shown in Fig. 3a, plenty of nanoparticles (red arrows) were observed at lysosomes/endosomes (yellow circles) in the cytoplasm. Apoptosis bodies (blue circles) were observed after applying the AMF for 10 min. Surface modification on the biomaterials is profitable for enhancing the targeting property and the transfection efficiency by various bioactive compounds such as cell-penetrating peptides, galactose and folic acid, etc.57–60 The targeting capacity of the nanoagents via folic acid-receptor (FR) was further evaluated by comparing the cellular uptake of MMNCs@PCM@Vc in both FR positive (FR+) and negative (FR−) tumor cells. In this study, different types of energetic FR cells such as KB (FR+++), HeLa(FR++), C6(FR+), HepG2(FR−) and A549 (FR−−) cells were used to measure the targeting efficiency of the nanoagent. As shown in Fig. S9 (ESI†), the intracellular phagocytosis efficiency is in the order of KB > HeLa > C6 > HepG2 > A549, and the FR-mediated cell uptake could be attenuated by prior addition of free folic acid, suggesting that the MMNCs@PCM@Vc could be used as an excellent candidate for FR-mediated targeted drug delivery. Meanwhile, to further investigate the phagocytosis pathway of the MMNCs@PCM@Vc nanoparticles, HeLa cells were treated with low temperature (4 °C), NaN3/DOG and different endocytic inhibitors to determine the endocytosis pathway of the nanoagent. As presented in Fig. S10 (ESI†), the results indicate that the transport of MMNCs@PCM@Vc for cellular entry was an active transport process via energy-dependent and both clathrin and caveolae mediated endocytosis.
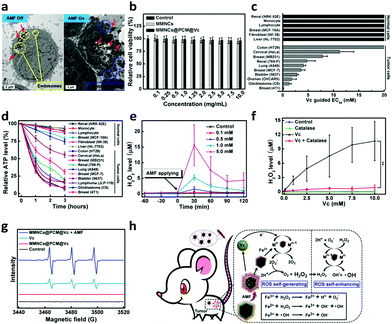 |
| Fig. 3
In vitro evaluations. (a) TEM images of HeLa cell incubation with MMNCs@PCM@Vc before and after AMF (4 kW, 300 A and 200 kHz) application. (b) Relative survival of HeLa cells under various concentrations of MMNCs@PCM@Vc for 24 h (n = 5). (c) Relative cytotoxicity of Vc (0–20 mM) on different types of normal and tumor cells. EC50 values indicate the concentration of Vc that reduced survival by 50%. (d) Relative ATP levels of normal and tumor cells at different time points after treatment with 2.0 mM VC. (e) H2O2 formation in extracellular fluid with different concentrations of Vc. (f) H2O2 levels of HeLa cells at different concentrations of Vc after treatment with 2.0 mM catalase. (g) EPR spectra showing the radical level produced by MMNCs@PCM@Vc upon AMF application. (h) Schematic illustration of MMNCs@PCM@Vc induced ROS self-generation and self-enhancement behaviour (Fenton reaction-like) in tumor cells. | |
Before MMNCs@PCM@Vc was used for in vivo tumor therapy application, we firstly evaluated its potential cytotoxicity for HeLa cells in vitro. The MTT result revealed that there was negligible cytotoxicity even at a relatively high concentration of 10.0 mg mL−1 (Fig. 3b). Next, to verify the specific killing effect of Vc on tumor cells, a panel of 6 normal cells and 10 tumor cells were exposed to Vc in vitro for 2 h and the effective concentration for 50% decreased survival (EC50) was further detected. As presented in Fig. 3c, the measured EC50 was less than 10 mM Vc for most of the tumor cells, and there was no obvious cytotoxicity in normal cells even with 20 mM Vc. Moreover, the ATP generation was mostly interdicted in tumor cells, while only slightly reduced in normal cells (Fig. 3d). It indicated that the Vc had a preferable selective killing effect against tumor cells. Next, the extracellular H2O2-producing profile by Vc was measured by using a H2O2 fluorescent probe (Fig. 3e). When the antioxidant was employed to withstand the possible generated H2O2, the H2O2 level significantly improved compared to that of no catalase addition (Fig. 3f). Furthermore, the enhanced ROS generation induced by the MMNCs was measured by an ESR detection. Herein, 5,5-dimethyl-1-pyrroline-N-oxide (DMPO) was used as an ˙OH spin-trapping agent by forming detectable DMPO-OH.61 Upon applying the AMF, the MMNCs@PCM@Vc promoted the tumor cells' production of ˙OH; in contrast, the ˙OH signal was not obvious in the Vc alone group (Fig. 3g). The results indicated that the H2O2 induced by Vc was enhanced to ˙OH with a higher oxidation ability, which was ascribed to the Fenton-like effect62 between the iron ions (released from MMNCs) and H2O2 (Fig. 3h). Hereby, this collaborative treatment presented much higher therapeutic efficiency than those of hyperthermia or Vc used alone (Fig. S11, ESI†).
The trypan blue staining assay also revealed that the synergistic magnetic hyperthermia and ROS therapy of MMNCs@PCM@Vc induced severe cell death compared to monotherapy (Fig. S12, ESI†). This result was proved by CLSM observation (Fig. S13, ESI†); the synergistic hyperthermia and ROS therapy of MMNCs@PCM@Vc led to significant cell apoptosis.
2.3.
In vivo multi-modal imaging
Meanwhile, the multimodal imaging capacity of the nanoagent was evaluated in HeLa tumor-bearing nude mice. The mice were intravenously (i.v.) injected with MMNCs@PCM@Vc (5.0 mg mL−1, 200 μL, Vc: 5.0 mM) and observed with a 3.0 T MR scanner. Accordingly, compared to the pre-injection control group, the nanocargo-injected group showed remarkably enhanced T2-weighted MRI signal at the tumor location. The PAI signals of the AMF-treated mice were recorded at 0, 1, and 6 h time points with a photoacoustic computed tomography scanner (Fig. 4c and d). The PAI signals were consistent with the conclusion of a previous study,63 indicating that the nanoplatform could be used as a PAI agent for illuminating the tumor area and delineating the margin of the tumor. Meanwhile, the temperature change of the tumor regions was recorded at specific time intervals. Mice injected with magnetic drug cargoes presented significant heat production under the AMF with a concentration-dependent performance. The temperature of the central tumor increased from 26.8 to 42.8 °C in 10 min and the surrounding tissue near the tumor site exhibited a near physiological temperature. In contrast, the control groups injected with PBS showed no significant increase (from 26.7 to 27.8 °C) under the applied AMF (Fig. 4e and f).
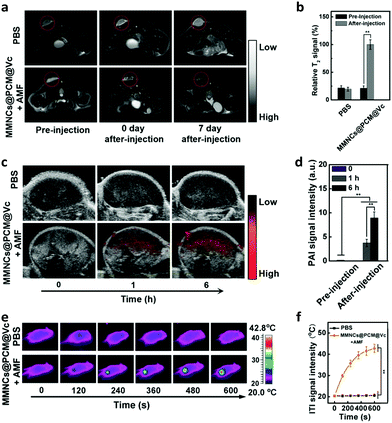 |
| Fig. 4
In vivo tri-modal imaging. (a) T2-MR images of tumors before and after injection of MMNCs@PCM@Vc and under the AMF. (b) T2-MR signal level in tumors before and after injection of MMNCs@PCM@Vc for 6 h. (c) PAI signals in the tumors before and after i.v. injection of MMNCs@PCM@Vc within 6 h. (d) PAI signal change during the injection of MMNCs@PCM@Vc and under the AMF (4 kW, 300 A and 200 kHz). (e) Infrared thermographic images (ITI) of tumor-bearing nude mice subjected to the AMF (4 kW, 300 A and 200 kHz) after injection of MMNCs@PCM@Vc (5.0 mg mL−1). (f) Temperature increase during the magnetic hyperthermia procedure. | |
2.4.
In vivo pharmacokinetics and biodistribution study
After validation of the in vivo imaging potential of the MMNCs@PCM@Vc, the pharmacokinetics (PK) and biodistribution (BioD) were quantitatively assessed for HeLa tumor bearing mice. We firstly detected the blood circulation behavior of MMNCs@PCM@Vc at different time intervals. As shown in Fig. 5a, the nanoparticles were generally cleared out of the blood stream in 12 h. Next, the BioD evaluation of MMNCs@PCM@Vc was performed to evaluate the targeting efficiency via the synergistic EPR effect and magnetic guidance. Typically, tumors and organs of mice were dissected for further Fe content measurements. The result showed that higher intratumoral nanoparticle accumulation was achieved for the magnetic guidance (+MF) groups compared with the no-magnetic guidance (−MF) groups (Fig. 5b). Meanwhile, for mice treated with MMNCs@PCM@Vc, there was significant nanoparticle accumulation in the liver both at 1 and 48 h. The accumulation of nanoparticles in the kidneys was detected as 0.84% at 1 h and 5.93% at 48 h, respectively, indicating that the bioclearance of the nanoagent occurred via a renal metabolism pathway. Furthermore, to test whether our strategy could efficiently reverse the intratumoral hypoxia or not, the in vivo PAI was performed. Four nude mice were xenografted i.v. with MMNCs@PCM@Vc. Their tumor oxygenation status showed a further increase when compared to those with PBS or MMNCs alone (Fig. 5c and Fig. S14, ESI†). Thereafter, the partial pressure of oxygen (pO2) level dramatically increased from 5.5 to 26.7 mmHg, while tumor pO2 levels of mice without the application of the AMF changed only slightly (Fig. 5d), presumably due to the supply of H2O2 by the released Vc under the AMF.
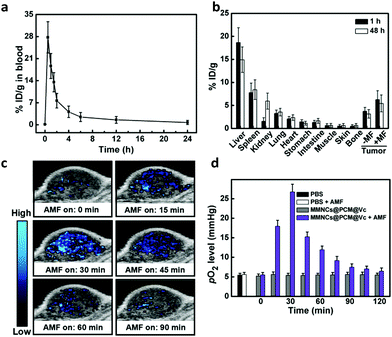 |
| Fig. 5 (a) Blood retention of MMNCs@PCM@Vc in HeLa tumor bearing mice within 24 h after i.v. injection. (b) Biodistribution of MMNCs@PCM@Vc in HeLa tumor bearing mice at 1 h and 48 h post i.v. injection. (c) Representative PA images for tumor oxygenation status by measuring the ratios of oxygenated hemoglobin in tumor-bearing nude mice at various time points post injection. (d) Corresponding pO2 level of tumor at different time periods under AMF application. | |
2.5.
In vivo anti-tumor efficacy
To further verify the therapeutic efficacy of the hypoxia specific nanoagent for tumor therapy, the HeLa tumor-bearing mice were divided into 8 groups (five mice per group): treated with PBS (Group I), PBS + AMF (Group II), Vc (Group III), Vc + AMF (Group IV), MMNCs (Group V), MMNCs + AMF (Group VI), MMNCs@PCM@Vc (Group VII) and MMNCs@PCM@Vc + AMF (Group VIII). When the tumor sizes reached about 60 mm,3 the therapeutic approaches were initiated for tumor ablation (Fig. 6a). After i.v. injection of magnetic nanoparticles for 2 h, a pint-sized magnet was placed at the tumor location for 6 h to anchor the nanoagent in a targeted manner and the AMF was applied (once every other day, 10 min per each time, two times in total). The tumor sizes of mice were measured by a caliper every two days. As presented in Fig. 6b and Fig. S15 (ESI†), Vc exhibited a certain curative effect, but failed to thoroughly inhibit the growth of tumors. Meanwhile, MMNCs and MMNCs@PCM@Vc could not cause any obvious damage to tumors without the AMF, which revealed good biocompatibility of the nanoagent. Single hyperthermia treatment caused certain tumor inhibition by moderate heating. Comparatively, MMNCs@PCM@Vc exhibited good curative outcome, in which the tumors were almost completely removed (Fig. 6c and d). These results were ascribed to the cooperation between the hyperthermia effect of MMNCs and the ROS activity of MMNCs@PCM@Vc in the tumor. Moreover, the mice in the synergistic treatment group had much higher survival rate than those in other groups (Fig. 6e). Throughout the therapeutic treatment, none of the mice showed significant change in body weight (Fig. S16, ESI†). Furthermore, histomorphological examination was conducted to examine the morphological change of tumors during the different therapeutic processes. As shown in Fig. 6f, the H&E staining revealed that the synergistic hypoxia overcoming tumor therapy strategy led to more effective tumor suppression.
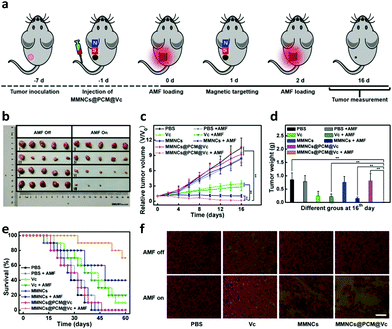 |
| Fig. 6 Anti-tumor therapeutic effect in vivo. (a) A therapeutic schedule of MMNCs@PCM@Vc for tumor suppression. (b) Photographs of the harvested tumors after different treatments (group I: PBS, group II: PBS + AMF, group III: Vc, group IV: Vc + AMF, group V: MMNCs, group VI: MMNCs + AMF, group VII: MMNCs@PCM@Vc and group VIII: MMNCs@PCM@Vc + AMF) for 16 days. (c) Relative tumor volumes after different treatments (n = 5). (d) Average tumor weights of mice at day 16 after different therapy administration. (e) Survival rates of tumor-bearing nude mice of the above different groups during the complete experimental period of 60 days (n = 10). (f) H&E examinations of the tumor slices in different therapeutic groups at day 16 (scale bar: 100 μm). | |
2.6. Biosafety evaluation
Finally, to ensure the biosecurity of the MMNCs@PCM@Vc for potential clinic application, the hemocompatibility of the as-prepared nanoparticles was further assessed by hemolytic ratio and blood coagulation time test (Table S1, ESI†). Considering the potential toxicity of the as-synthesized nanoagent, the standard blood biochemistry and hematology assays were also carried out at 1, 14 and 28 days post injection of the MMNCs@PCM@Vc (Fig. 7a–l). Accordingly, most of the hematological indexes were at normal levels and no significant statistic difference was found, demonstrating that MMNCs@PCM@Vc was a safe therapeutic regimen for animals. Simultaneously, H&E staining was performed to evaluate the histological toxicity of the nanoagent in main organs. No obvious damage was observed in MMNCs@PCM@Vc treated mice (Fig. 7m and Fig. S17, S18, ESI†), suggesting that the as-synthesized nanoagent was safe for in vivo clinic use. More remarkably, the iron mesoporous nanocubes could degrade in the acidic microenvironment. As shown in Fig. 8, the nanocubes finally degraded to infinitesimal nanoparticles in the simulation biological tumor environment with slight acidiity (pH = 5.0), indicating its good biodegradability.
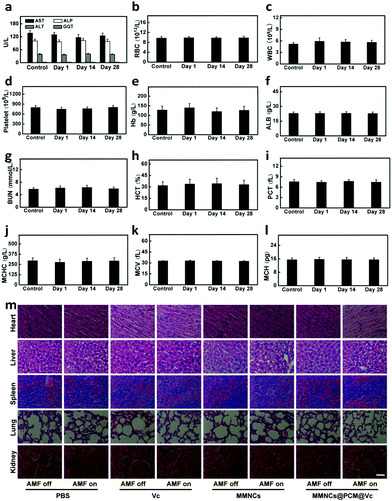 |
| Fig. 7
In vivo biocompatibility evaluation for mice injected with MMNCs@PCM@Vc. Blood biochemical indexes (n = 5) of mice determined on the 1th, 14th and 28th day, respectively. The examined items include (a) aspartate aminotransferase (AST), alanine aminotransferase (ALT), alkaline phosphatase (ALP), and glutamyltranspetidase (GGT) concentrations; (b) red blood cell (RBC) counts; (c) white blood cell (WBC) counts; (d) platelet (PLT) counts; (e) hemoglobin (HB) level; (f) albumin (ALB) content; (g) blood urea nitrogen (BUN) level; (h) hematocrit (HCT) level; (i) plateletcrit (PCT) level; (j) mean corpuscular hemoglobin concentration (MCHC) content; (k) mean corpuscular volume (MCV) level; (l) mean corpuscular haemoglobin (MCH) content. (m) Micrographs for the H&E-stained major organ tissues of mice at the 28th day after injection of MMNCs@PCM@Vc, scale bars: 100 μm. | |
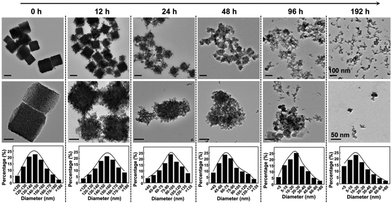 |
| Fig. 8 TEM monitoring of MMNCs in the lysosome-like medium (pH = 5.0). The MMNC nanoparticles at the initial state gradually eroded and even totally dissolved after 192 h in the lysosome-like acidic medium. | |
3. Conclusions
In summary, we developed a hypoxia specific strategy based on biocompatible and biodegradable MMNCs@PCM@Vc for tumor therapy. The thermosensitive nanoplatform could be triggered by an external AMF and thus destroy tumor cells by subsequent hyperthermia and aggregated ROS. Moreover, the system not only exhibited effective anti-tumor performance, but also showed desirable biocompatibility, hemocompatibility and biodegradability in vitro and in vivo. Taken together, the Vc mediated ROS self-generation and nanocarrier dominated ROS self-enhanced nanotheranostics is a valid approach to tumor ablation. Our work presents an ingenious delivery system design strategy to fabricate hypoxia overcoming nanoagents, as demonstrated herein, not only potentially useful for developing biodegradable functional nanostructures with low side effects, but also paving a new way for advancing ROS-enhanced cancer-therapeutic modalities.
Conflicts of interest
There are no conflicts to declare.
Acknowledgements
This work was partially supported by the National Natural Science Foundation of China (No. 31640030, 31800836, 51825302 and 21734002), the National Key R&D Program of China (Grant No. 2016YFC1100300), the Innovation Team in University of Chongqing Municipal Government (CXTDX201601002) and the Key R&D Program of Henan Province (No. 192102310194).
References
- J. J. Liu, Q. Chen, L. Z. Feng and Z. Liu, Nano Today, 2018, 21, 55 CrossRef CAS.
- L. A. Liotta and E. C. Kohn, Nature, 2001, 411(6835), 375 CrossRef CAS.
- C. Belli, D. Trapani, G. Viale, P. D'Amico, B. A. Duso, P. D. Vigna, F. Orsi. and G. Curigliano, Cancer Treat. Rev., 2018, 65, 22 CrossRef CAS.
- J. N. Liu, W. B. Bu and J. L. Shi, Chem. Rev., 2017, 117, 6160 CrossRef CAS PubMed.
- L. H. Fu, C. Qi, J. Lin and P. Huang, Chem. Soc. Rev., 2018, 47, 6454 RSC.
- W. Y. Zhen, Y. Liu, X. D. Jia, L. Wu, C. Wang and X. E. Jiang, Nanoscale Horiz., 2019, 4, 720 RSC.
- N. Niu, Z. Zhang, X. Gao, Z. C. Chen, S. J. Li and J. Li, Chem. Eng. J., 2018, 352, 818 CrossRef CAS.
- J. Yang, W. Li, L. H. Luo, M. S. Jiang, C. Q. Zhu, B. Qin, H. Yin, X. L. Yuan, X. Y. Yin, J. L. Zhang, Z. Y. Luo, Y. Z. Du and J. You, Biomaterials, 2018, 182, 145 CrossRef CAS.
- S. Rey, L. Schito, M. Koritzinsky and B. G. Wouters, Adv. Drug Delivery Rev., 2017, 109, 45 CrossRef CAS.
- N. Chen, Y. P. Han, Y. Luo, Y. F. Zhou, X. J. Hu, Y. Yu, X. D. Xie, M. Yin, J. L. Sun, W. Y. Zhong, Y. Zhao, H. Y. Song and C. H. Fan, Mater. Horiz., 2018, 5, 1204 RSC.
- K. D. Patel, R. K. Singh and H. W. Kim, Mater. Horiz., 2019, 6, 434 RSC.
- D. W. Zheng, B. Li, C. X. Li, J. X. Fan, Q. Lei, C. Li, Z. Xu and X. Z. Zhang, ACS Nano, 2016, 10(9), 8715 CrossRef CAS.
- P. E. Bickler and L. T. Buck, Annu. Rev. Physiol., 2007, 69, 145 CrossRef CAS.
- H. J. Zhu, J. C. Li, X. Y. Qi, P. Chen and K. Y. Pu, Nano Lett., 2018, 18(1), 586 CrossRef CAS.
- X. H. Lin, Y. Qiu, L. Song, S. Chen, X. F. Chen, G. M. Huang, J. B. Song, X. Y. Chen and H. G. Yang, Nanoscale Horiz., 2019, 4, 747 RSC.
- M. Z. Zou, W. L. Liu, C. X. Li, D. W. Zheng, J. Y. Zeng, F. Gao, J. J. Ye and X. Z. Zhang, Small, 2018, 14(20), 1801120 CrossRef PubMed.
- C. P. Liu, T. H. Wu, C. Y. Liu, K. C. Chen, Y. X. Chen, G. S. Chen and S. Y. Lin, Small, 2017, 13(26), 201700278 CrossRef PubMed.
- H. C. Chen, J. W. Tian, W. J. He and Z. J. Guo, J. Am. Chem. Soc., 2015, 137(4), 1539 CrossRef CAS.
- H. Kim, Q. Lin and Z. Yun, Cancer Lett., 2018, 431, 142 CrossRef CAS PubMed.
- M. W. Baek, H. S. Cho, S. H. Kim, W. J. Kim and J. Y. Jung, J. Cell. Physiol., 2017, 232(2), 417 CrossRef CAS.
- H. W. Lv, C. Z. Wang, T. Fang, T. Li, G. S. Lv, Q. Han, W. Yang and H. Y. Wang, npj Precis. Oncol., 2018, 2(1), 1 CrossRef CAS.
- M. Agathocleous, C. E. Meacham, R. J. Burgess, E. Piskounova, Z. Y. Zhao, G. M. Crane, B. L. Cowin, E. Bruner, M. M. Murphy, W. N. Chen, G. J. Spangrude, Z. P. Hu, R. J. DeBerardinis and S. J. Morrison, Nature, 2017, 549(7673), 476 CrossRef.
- J. H. Yun, E. Mullarky, C. Y. Lu, K. N. Bosch, A. Kavalier, K. Rivera, J. Roper, I. C. Chio, E. G. Giannopoulou, C. Rago, A. Muley, J. M. Asara, J. Paik, O. Elemento, Z. M. Chen, D. J. Pappin, L. E. Dow, N. Papadopoulos, S. S. Gross and L. C. Cantley, Science, 2015, 350, 1391 CrossRef CAS.
- Q. An, C. Y. Sun, D. Li, K. Xu, J. Guo and C. C. Wang, ACS Appl. Mater. Interfaces, 2013, 5(24), 13248 CrossRef CAS.
- C. M. Doskey, V. Buranasudja, B. A. Wagner, J. G. Wilkes, J. Du, J. J. Cullen and G. R. Buettner, Redox Biol., 2016, 10, 274 CrossRef CAS.
- Q. Chen, M. G. Espey, A. Y. Sun, J. Lee, M. C. Krishna, E. Shacter, P. L. Choyke, C. Pooput, K. L. Kirk, G. R. Buettner and M. Levine, Proc. Natl. Acad. Sci. U. S. A., 2007, 104(21), 8749 CrossRef CAS.
- H. Wang, B. Lv, Z. M. Tang, M. Zhang, W. Q. Ge, Y. Y. Liu, X. L. He, K. L. Zhao, X. P. Zheng, M. Y. He and W. B. Bu, Nano Lett., 2018, 18(9), 5768 CrossRef CAS.
- J. Chen, H. L. Luo, Y. Liu, W. Zhang, H. X. Li, T. Luo, K. Zhang, Y. X. Zhao and J. J. Liu, ACS Nano, 2017, 11(12), 12849 CrossRef CAS.
- G. P. Wang, G. W. Zeng, C. Wang, H. S. Wang and B. Yang, Biomed. Pap., 2015, 159(2), 227 CrossRef.
- T. Wang, J. H. Hu, H. Luo, H. Y. Li, J. H. Zhou, L. Zhou and S. H. Wei, Small, 2018, 14(38), 1802337 CrossRef.
- X. H. Wang, X. Y. Wang, S. X. Jin, M. N. Uhammad and Z. J. Guo., Chem. Rev., 2018, 8b00209 Search PubMed.
- W. B. Zhang, H. Y. Li, Y. Qin and C. Y. Gao, Mater. Horiz., 2017, 4, 1135 RSC.
- K. W. Chang, Z. H. Liu, X. F. Fang, H. B. Chen, X. J. Men, Y. Yuan, K. Sun, X. J. Zhang, Z. Yuan and C. F. Wu, Nano Lett., 2017, 17(7), 4323 CrossRef CAS.
- W. Lv, H. T. Xia, K. Y. Zhang, Z. J. Chen, S. J. Liu, W. Huang and Q. Zhao, Mater. Horiz., 2017, 4, 1185 RSC.
- X. J. Cheng, Y. Yong, Y. H. Dai, X. Song, G. Yang, Y. Pan and C. C. Ge, Theranostics, 2017, 7(17), 4087 CrossRef CAS.
- C. W. Song, H. Park and R. J. Griffin, Radiat. Res., 2001, 155, 515 CrossRef CAS.
- C. Song, A. Shakil, J. Osborn and K. Iwata, Int. J. Hyperthermia, 2009, 25, 91 CrossRef CAS.
- J. H. Li, F. S. Zhang, Z. G. Hu, W. D. Song, G. D. Li, G. F. Liang, J. Zhou, K. Li, Y. Cao, Z. Luo and K. Y. Cai, Adv. Healthcare Mater., 2017, 6(14), 201700005 Search PubMed.
- X. X. Yao, X. X. Niu, K. X. Ma, P. Huang, J. Grothe, S. Kaskel and Y. F. Zhu, Small, 2017, 13(2), 201602225 CrossRef.
- J. H. Li, Y. Hu, Y. H. Hou, X. K. Shen, G. Q. Xu, L. L. Dai, J. Zhou, Y. Liu and K. Y. Cai, Nanoscale, 2015, 7, 9004 RSC.
- J. Yao, Y. Cheng, M. Zhou, S. Zhao, S. C. Lin, X. Y. Wang, J. J. Wu, S. R. Li and H. Wei, Chem. Sci., 2018, 9(11), 2927 RSC.
- Z. Z. Wang, Y. Zhang, E. G. Ju, Z. Liu, F. F. Cao, Z. W. Chen, J. S. Ren and X. G. Qu, Nat. Commun., 2018, 9(1), 3334 CrossRef.
- T. Huang, G. M. Zhang, N. Zhang, J. Ye and G. Xian, Chem. – Eng. J., 2018, 346, 120 CrossRef CAS.
- Z. M. Tang, Y. Y. Liu, M. Y. He and W. B. Bu, Angew. Chem., Int. Ed., 2019, 58(4), 946 CrossRef CAS.
- Z. B. Cao, L. Zhang, K. Liang, S. H. Cheong, C. Boyer, J. J. Gooding, Y. Chen and Z. Gu, Adv. Sci., 2018, 5(11), 1801155 CrossRef.
- Y. Liu, W. Y. Zhen, Y. H. Wang, J. H. Liu, L. H. Jin, T. Q. Zhang, S. T. Zhang, Y. Zhao, S. Y. Song, C. Y. Li, J. J. Zhu, Y. Yang and H. J. Zhang, Angew. Chem., Int. Ed., 2019, 201813702 Search PubMed.
- G. S. Song, M. Chen, Y. R. Zhang, L. Y. Cui, H. B. Qu, X. C. Zheng, M. Wintermark, Z. Liu. and J. H. Rao, Nano Lett., 2018, 18(1), 182 CrossRef CAS.
- J. Mu, J. Lin, P. Huang and X. Y. Chen, Chem. Soc. Rev., 2018, 47, 5554 RSC.
- Y. K. Duan, Y. Xu, D. Mao, W. H. Liew, B. Guo, S. W. Wang, X. L. Cai, N. Thakor, K. Yao, C. J. Zhang and B. Liu, Small, 2018, 14(42), 1800652 CrossRef.
- L. Lartigue, D. Alloyeau, J. Kolosnjaj-Tabi, Y. Javed, P. Guardia, A. Riedinger, C. Péchoux, T. Pellegrino, C. Wilhelm and F. Gazeau, ACS Nano, 2013, 7(5), 3939 CrossRef CAS.
- P. W. Lee, S. H. Hsu, J. J. Wang, J. S. Tsai, K. J. Lin, S. P. Wey, F. R. Chen, C. H. Lai, T. C. Yen and H. W. Sung, Biomaterials, 2010, 31(6), 1316 CrossRef CAS PubMed.
- B. W. Yang, Y. Chen and J. L. Shi, Adv. Mater., 2019, 1901778 CrossRef.
- B. W. Yang, Y. Chen and J. L. Shi, Chem. Rev., 2019, 119, 4881 CrossRef CAS.
- J. Zhou, M. H. Li, Y. H. Hou, Z. Luo, Q. F. Chen, H. X. Cao, R. L. Huo, C. C. Xue, L. Sutrisno, L. Hao, Y. Cao, H. T. Ran, L. Lu, K. Li and K. Y. Cai, ACS Nano, 2018, 12(3), 2858 CrossRef CAS.
- D. L. A. de Faria, S. V. Silva and M. T. de Oliveira, J. Raman Spectrosc., 1997, 28, 873–878 CrossRef CAS.
- Y. Zhang, Y. J. Shen, X. Y. Teng, M. Q. Yan, H. Bi and P. C. Morais, ACS Appl. Mater. Interfaces, 2015, 7, 10201 CrossRef CAS.
- X. R. Deng, K. Li, X. C. Cai, B. Liu, Y. Wei, K. R. Deng, Z. X. Xie, Z. J. Wu, P. A. Ma, Z. Y. Hou, Z. Y. Cheng and J. Lin, Adv. Mater., 2017, 29(36), 1701266 CrossRef.
- J. M. Wolfe, C. M. Fadzen, Z. N. Choo, R. L. Holden, M. Yao, G. J. Hanson and B. L. Bradley, ACS Cent. Sci., 2018, 4(4), 512 CrossRef CAS.
- X. Zhang, Z. Q. Xi, J. O. Machuki, J. J. Luo, D. Z. Yang, J. J. Li, W. B. Cai, Y. Yang, L. J. Zhang, J. W. Tian, K. J. Guo, Y. Y. Yu and F. L. Gao, ACS Nano, 2019, 13(5), 5306 CrossRef CAS.
- J. H. Park, D. Dehaini, J. R. Zhou, M. Holay, R. H. Fang and L. F. Zhang, Nanoscale Horiz., 2019 10.1039/C9NH00291J.
- W. D. Ke, J. J. Li, F. Mohammed, Y. H. Wang, K. Tou, X. Y. Liu, P. Y. Wen, H. Kinoh, Y. Anraku, H. B. Chen, K. Kataoka and Z. S. Ge, ACS Nano, 2019, 13(2), 2357 CAS.
- W. Feng, X. G. Han, R. Y. Wang, X. Gao, P. Hu, W. W. Yue, Y. Chen and J. L. Shi, Adv. Mater., 2018, 1805919 CrossRef.
- X. M. Guo, Z. Wu, W. Li, Z. H. Wang, Q. P. Li, F. F. Kong, H. B. Zhang, X. L. Zhu, Y. P. Du, Y. Jin, Y. Z. Du and J. You, ACS Appl. Mater. Interfaces, 2016, 8(5), 3092 CrossRef CAS.
Footnotes |
† Electronic supplementary information (ESI) available: Experimental details, materials characterization and histological staining. See DOI: 10.1039/c9nh00490d |
‡ These authors contributed equally to this work. |
|
This journal is © The Royal Society of Chemistry 2020 |