DOI:
10.1039/D0NA00257G
(Review Article)
Nanoscale Adv., 2020,
2, 2220-2233
Recent advances in the template-confined synthesis of two-dimensional materials for aqueous energy storage devices
Received
1st April 2020
, Accepted 28th April 2020
First published on 28th April 2020
Abstract
The template-confined synthesis strategy is a simple and effective methodology to prepare two-dimensional nanomaterials. It has multiple advantages including green process, controllable morphology and adjustable crystal structure, and therefore, it is promising in the energy storage realm to synthesize high-performance electrode materials. In this review, we summarize the recent advances in the template-confined synthesis of two-dimensional nanostructures for aqueous energy storage applications. The material design is discussed in detail to accommodate target usage in aqueous supercapacitors and zinc metal batteries. The remaining challenges and future prospective are also covered.
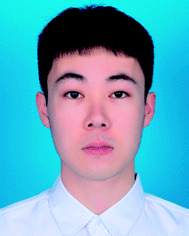 Zhengnan Tian | Zhengnan Tian received his BS degree from Soochow University in 2017. He is currently an MS candidate in College of Energy, Soochow Institute for Energy and Materials InnovationS (SIEMIS), Soochow University under the supervision of Prof. Jingyu Sun. His current research relates to aqueous energy storage devices and multi-field integrated systems. |
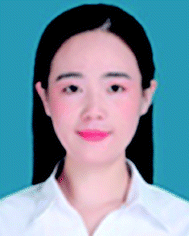 Chaohui Wei | Chaohui Wei received her bachelor's degree from Xi'an Jiaotong University in 2014 and her PhD from the University of Nottingham in 2019. She is currently a postdoctoral research fellow in College of Energy, Soochow University under the supervision of Prof. Jingyu Sun and Prof. Ruizhi Yang. Her current research interests focus on flexible and wearable energy storage devices, including Li–S and Zn–air batteries. |
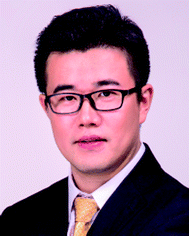 Jingyu Sun | Jingyu Sun earned his bachelor's degree (2008) from Zhejiang University and obtained his PhD (2013) from the University of Oxford. After two-year postdoc training with Prof. Zhongfan Liu at Peking University, he joined the University of Cambridge as a postdoctoral research fellow in 2015. Since March 2017 he became a full professor in College of Energy, Soochow University. His research interests center on CVD design of graphene materials and printing methodology for wearable energy systems. |
1. Introduction
The growing demand for the miniaturization and multi-functionalization of electronic devices has stimulated the development of flexible and portable electrochemical energy storage (EES) devices.1 Traditional alkali metal batteries, such as commercial lithium ion batteries, sodium ion batteries and potassium ion batteries, have been extensively attempted to aim for micro-EES devices. These systems normally adopt organic electrolytes to harvest favourable energy density and long durability. Nevertheless, their safety concerns due to the toxicity, flammability and corrosion of organic electrolytes pose a daunting threat.2 In this respect, aqueous electrolyte-based EES devices such as aqueous supercapacitors,3 aqueous zinc batteries (zinc ion batteries, zinc alkaline batteries and zinc air batteries) and aqueous alkali metal ion batteries have emerged as a research hotspot.4–7 These aqueous systems are featured by high safety, excellent rate performance and ease-of-assembly, making it an ideal candidate for future portable EES.
The electrode material is an essential component in all EES devices; hence, strenuous efforts have been devoted to tailor its innovative structural and compositional design. In an aqueous electrolyte, the cation typically exhibits as hydrated forms such as [Na(H2O)6]+, [K(H2O)8]+, [Mg(H2O)6]2+ and [Al(H2O)6]3+, leading to a sizeable hydrated ionic radius in comparison with those in the organic electrolytes.8,9 In line with this, exploring advanced materials with expanded layer spacings for rapid intercalation of ions is highly desirable, specifically for the insertion reaction-based zinc ion batteries. With regard to aqueous supercapacitors, the key lies in the design of electrode components with either a large specific surface area to boost ion adsorption/desorption, or sufficient active sites to harness redox reactions. Accordingly, two-dimensional (2D) materials have come onto the stage, owing to their favourable properties toward electrochemical process. For instance, graphene and its derivatives (graphene oxide (GO) and reduced GO (rGO)) have served as the fundamental building blocks for various EES devices, rendering high electronic conductivity and special 2D morphology.10 Other 2D materials, encompassing transition metal dichalcogenides (TMDs), metal–organic frameworks (MOFs), layered metal oxides (LMOs), layered double hydroxides (LDHs), MXene, etc.,11 have also become promising candidates in emerging energy systems. Specifically, their unique physical and chemical features including the large interlayer distance, interconnected pores and sufficient active sites enable the effective promotion of electrochemical reaction kinetics.
To date, great attention has been garnered to the deployment of reliable synthesis strategies for target 2D materials with desired structures and properties. In general, there are two main synthetic streams for producing 2D materials: bottom-up and top-down routes. Bottom-up approach involves the liquid phase reaction (e.g., organic ligand-assisted growth) and vapor phase reaction (e.g., chemical vapor deposition). The top-down method mainly includes mechanical exfoliation of bulk materials in various media.12 Of note, the bottom-up method is more widely adopted due to the versatile morphologies and the precisely controllable properties of final products. Indeed, there have been several review articles dealing with the introduction of different synthetic methods for 2D materials.13 Amongst them, the template-confined strategy has been considered as a simple, effective and versatile bottom-up approach for synthesizing 2D nanostructures, which has advanced capability to well define the size, shape and configuration of target materials and holds a great implication for applications as compared with the other synthetic strategies. Nevertheless, recent reviews focusing on the template-confined growth strategy have rarely been reported.
Herein, we first summarize the synthetic methods of 2D materials via template-confined strategies, covering the employment of dimensional templates (Scheme 1). We then provide an overview of recent advances in the target applications such as aqueous supercapacitors, aqueous zinc ion batteries and zinc air batteries. Finally, we analyse the pros and cons of the synthetic methods and propose the future directions of the template-confined strategy for aqueous energy storage devices.
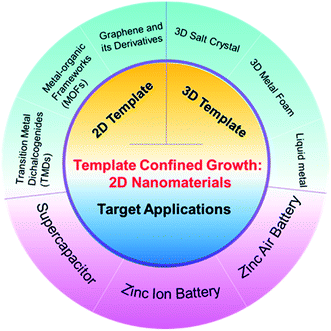 |
| Scheme 1 Outline of the template-confined growth strategy of 2D materials and their related applications in aqueous energy storage devices. | |
2. Synthesis strategy
The template-confined growth methodology is effective, feasible and universal amongst various synthetic strategies.14 In general, the morphological templates induce liquid phase ions or gaseous molecules to grow along desired directions thanks to the distinct surface activities in different dimensions. Key parameters such as the adsorption energy barrier, catalytic activity and hydrophilic property could dictate the material growth. Usually, the growth along the z-direction is limited so as to form the 2D structure. Furthermore, the employed templates are stable to not participate in chemical reactions upon synthesis.15 To fabricate the target materials, the template is required to have higher dimensions. Therefore, the dimension of such guiding agents for synthesizing 2D materials should be greater than or equal to two. In other words, templates possessing lower dimensions such as zero-dimensional quantum dots and one-dimensional nanowires are unlikely to form 2D productions. Accordingly, templates for the confined preparation of 2D materials are typically of 2D or three-dimensional (3D) forms, as demonstrated vide infra.
2.1. 2D templates
Various 2D materials themselves could act as excellent confined templates for the growth of designed 2D configurations, as the localized area restricts the growth along the z-direction. This section summarizes the commonly used (graphene and its derivatives, TMDs, MOFs, LDHs, hydroxides and biomass metal oxides) and emerging (MXenes, stanenes and graphdiynes) 2D templates as confined growth agents.
2.1.1. Graphene and its derivatives.
Graphene and its derivatives (GO and rGO), as the “star material”, have been extensively exploited in the realm of energy storage due to their high conductivity, unique 2D morphology and good mechanical robustness.16 In addition, their satisfactory tolerance to extreme environment and tailorable surface properties has by far ignited the interest in using them as hard templates to synthesize various 2D materials. In 2015, Sun et al. reported a synthetic strategy of GO/TiO2 hybrid laminates using the monolayer GO as a universal template.17 The hybrid exhibited excellent water desalination properties. Subsequently, Saito et al. proposed that the confined space between two GO layers were ideal for constructing various types of oxide layers. In this view, polycrystalline TiO2, ZnO, Fe2O3 and amorphous SiO2 were successfully deposited on the GO layers with a thickness of several nanometers.18 Chen et al. also reported that graphene with various textures can be employed as versatile templates for preparing 2D metal oxide crystalline films with similar textures, including crumpled, wrinkled and hierarchical texture (Fig. 1). The intercalated metal ions were confined between van der Waals gaps of GO, followed by in situ conversion and annealing, wherein the ultrathin 2D metal oxide nanoplates (ZnO, Al2O3, Mn2O3 and CuO) were obtained.19 The formation mechanism here is pertaining to two respects: (1) electrostatic attraction, where exists strong electrostatic adsorption between the intercalated metal ions and GO sheets (with a net negative potential: −50 mV) and (2) chemical complexation, in which carboxyl groups on the surface of GO have a large binding energy with metal ions, which is in favour of forming chemically specific ion-carboxylate complexation. Along with the fabrication of binary metal oxides, Abdelhamid et al. extended the same GO-templated method to produce ternary oxide nanosheets (TixNbyOz, NixCoyOz and MnxCoyOz).20 Moreover, complicated oxide species, such as YBa2Cu3O7−δ, can also be synthesized via the GO-templated strategy by Boston and his colleagues.21
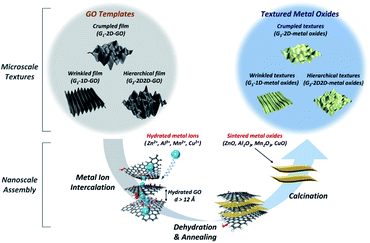 |
| Fig. 1 Creation of hierarchical metal oxide architectures using textured GO as a versatile ion intercalation template. Reproduced with permission.19 Copyright 2016, American Chemical Society. | |
It is worth noting that LDHs fabricated by such a GO-template method harvested large specific surface areas and enriched active sites.22 In addition to the production of a wide variety of compounds, graphene and its derivatives can be employed as the space-confined templates to synthesize noble metals (e.g., Au, Pt and Pd). Marangoni et al. developed a unique strategy of controllable growth of 2D gold with asymmetric nanostructures in aqueous media, using GO as a template (Fig. 2). Based on the microscopic investigations, gold nanoparticles underwent adsorption, nucleation and growth, readily forming a continuous 2D sheet on the GO template (with a ∼5 nm thickness).23
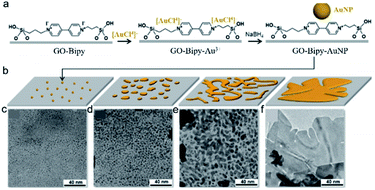 |
| Fig. 2 (a) GO functionalization and the synthesis of gold nanoparticles (seeds). (b) Growing steps of the asymmetric 2D gold nanostructures. (c–f) Corresponding TEM images. Reproduced with permission.23 Copyright 2018, Royal Society of Chemistry. | |
Although graphene and its derivatives have been widely utilized as templates, stacking issues between graphene layers often affect the growth of 2D nanosheets. Moreover, aiming at removing the template, the additional calcination process might be detrimental to the surface properties of obtained products.
2.1.2. Transition metal dichalcogenides (TMDs).
TMDs are typical 2D layered compounds with the chemical formula of MX2, where M represents the transition metal (Fe, Mo, W, Mn, Re, V, etc.) and X denotes the chalcogen element (S, Se and Te). Similar to graphene, the van der Waals force between monolayers can offer suitable 2D confined spaces as the reaction platform to grow 2D materials. However, it is difficult to eliminate the TMD template after synthesis due to the non-volatility of metal elements upon annealing. In line with this, MoS2 species has normally been employed as a hard template for forming 2D heterojunctions in situ.
Such 2D MoS2 heterojunctions demonstrate widespread application potentials, e.g., in the realm of electrocatalysis governed by interfacial defect engineering. Moreover, a MoS2/metal/MoS2 heterostructure has been employed as a high-frequency metal-base transistor. Moreover, several intriguing properties have been witnessed at the epitaxially grown metals on MoS2. In 2013, Huang et al. reported an effective solution-phase growth, where Pd, Pt and Ag nanostructures could epitaxially grow on the MoS2 (001) planes mainly with the (111) and (101) orientations.24 Furthermore, a wide variety of thin metal films including Al, Ag, Cu, Mn, Mo, Ni, Pd, Ru, Re, and Zn can be synthesized on monolayer MoS2 due to the suitable lattice parameters.25 To the best of our knowledge, several possible scenarios occur when MoS2 and metal come into contact, such that new compounds could be formed, Mo or S lattices could be substituted by metal atoms, intercalation would be gained in between the MoS2 layers, and/or the two materials could form an atomically abrupt interface. On the basis of this, metal/MoS2 heterojunctions can be grown on MoS2 throughout synergetic manipulation of thermodynamics and kinetics.
Different hybrid nanomaterials can be synthesized via choosing different metal precursors (K2PtCl4, K2PdCl4, HAuCl4 or AgNO3) and reduction routes (chemical reduction or photochemical reduction). Wu et al. constructed MoxC–graphene (MoxC–G) heterojunctions in situ via a MoS2 atomic layer template.26Fig. 3a illustrates the growth procedures of MoxC–G. With the temperature increasing to 750 °C, the MoS2 atomic layer was reduced to Mo, where the sulfur was volatilized. The metal Mo can subsequently act as the catalyst for graphene growth. Simultaneously, some Mo atoms were carburized to form MoxC supported on graphene. Viewing from the thermodynamics analysis, the direct reaction between bcc-Mo (hcp-Mo) structures and C2H4 tended to occur due to the energetic favourability compared with the starting reactants of MoS2 and C2H4. In addition, the formation enthalpy of β-Mo2C was much lower than those of other potentially formed compounds during the synthetic process of MoxC–G hybrids. Fig. 3b and c show the SEM images of MoxC–graphene composites, still maintaining the triangular template morphology. Fig. 3d and e verify the uniform distribution of MoxC nanoparticles on the surface of triangles. Nevertheless, it was noted that other TMDs have rarely been applied in the synthesis of 2D materials as the confined templates. In this regard, further efforts are required to develop simple and ubiquitous templating strategies to fabricate 2D materials and/or their heterojunctions.
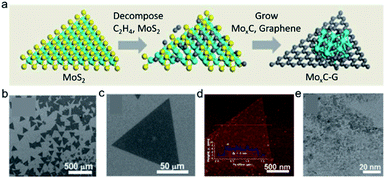 |
| Fig. 3 Microscopic characterization of MoxC–G hybrids. (a) Illustration of the synthesis procedure of MoxC–G hybrids. The cyan, yellow, and gray spheres represent molybdenum, sulfur, and carbon atoms, respectively. (b and c) SEM images of MoxC–G. (d) AFM image of the MoxC–G flake on a SiO2/Si substrate. The inset shows the height profile of the location as indicated by the solid white line. (e) TEM image of the MoxC–G hybrid. Reproduced with permission.26 Copyright 2017, Wiley-VCH. | |
In short, utilizing the TMDs as templates affords a feasible avenue to prepare 2D materials. However, in comparison with graphene templates, the removal of TMD templates remains a tough task.
2.1.3. Metal–organic frameworks (MOFs).
2D MOFs, generally formed by the coordination bonds between organic ligands and metal ion, have attracted growing attention because of the periodic structural unit.27–30 For example, they have highly ordered pore structures, large specific surface areas, regulable metal components, and ample active sites.31 The 2D layered structure, as a template, can provide the confined reaction space to grow certain 2D materials. More importantly, owing to the wide choices of metal elements and organic ligand molecules in MOFs, a variety of 2D nanosheets involving ultra-thin carbon, layered double hydroxide and metal oxide nanosheets can be easily prepared. The mainstream of MOF-derived 2D materials lies in the doped carbon nanosheets, encompassing the heteroatom dopants (N, P, B or S) and specific metal/metal oxide modifiers (Co, Ni, Fe, V, Mn, etc.). Kong et al. fabricated nitrogen-doped wrinkled carbon foils using a 2D Mn-MOF sacrificial template, where the MOF did not serve as a hard template but participated in the chemical reaction.32 The main preparation process is depicted in Fig. 4a, mainly dealing with pyrolysis and etching. After annealing in ammonia, Na and Mn components/N-rich carbon foils (MNC-1) were derived. Subsequently, 2D porous nitrogen-doped carbon nanosheets (NC-1) can be formed upon acid treatment. Fig. 4b shows the TEM images of the bulk Mn-MOF (i), 2D Mn-MOF (ii), MNC-1 (iii) and NC-1 (iiii). Obviously, the final porous carbon nanosheets inherited the 2D structure of the original MOF template. During the gas phase reaction, gas molecules reacted, consumed, nucleated and grew in the confinement space on the surface of the MOF material, leading to the formation of 2D nanostructures. Indeed, the template method with the assistance of MOF materials is quite practicable. Notably, various 2D structures of metal oxides, phosphides, sulfides or selenides can be in target produced by changing the precursor salt and annealing atmosphere.33–36
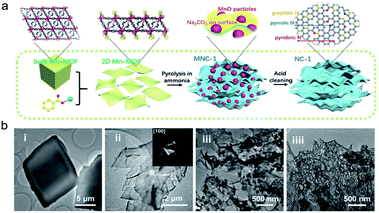 |
| Fig. 4 (a) Schematic of the formation process of NC-1 from bulk Mn-MOF. (b) TEM images of the bulk Mn-MOF (i), 2D Mn-MOF (ii), MNC-1 (iii) and NC-1 (iiii). Reproduced with permission.32 Copyright 2018, Wiley-VCH. | |
Notwithstanding these advances, it should be noted that the elimination of the MOF template without any change is not an easy task. In addition, the inherently sophisticated pore structure is more or less destroyed due to the elevated annealing temperature. Taking this into account, conductive MOF species are an effective material solution.
2.1.4. Other 2D templates.
Other 2D templates, including, but not limited to, LDHs,37 hydroxides38 and 2D metal oxides,39 have also been utilized to synthesize emerging 2D materials by the space-confined growth. A representative work by Wang et al. reported an innovative template route for synthesizing MoS2 flakes in rhomboid shape with controllable layer numbers. The MoS2 flakes were prepared by layer-by-layer sulfurization of MoO2 microcrystals, wherein MoO2 was employed as the reaction-confined platform and Mo source at the same time.40 Inspired by this investigation, self-template strategies have been widely utilized in attaining a wide variety of 2D materials, such as NbS2,41 WS2,42 and SnS2.43
2.2. 3D templates
3D textured materials can also be employed as the guiding templates to synthesize 2D materials. Distinct from the 2D templating scenario, the confined growth can only occur at the surfaces of 3D templates. In turn, the 3D structures can be disintegrated throughout sonication to form 2D layers. In this section, typical 3D templates such as cubic salt crystals and metal foams are described.
2.2.1. 3D salt crystals.
Salt, the most widely used template, is employed to synthesize materials of various types and scales, due to the low price, superior solubility in water and periodic unit cell. Along this line, Xiao et al. reported a generic salt-templated strategy for attaining 2D transition metal oxides, including hexagonal-MoO3, MoO2, MnO and hexagonal-WO3.15 The confined growth on the surface realized the matching of crystal lattices. Recently, Wei et al. have harnessed the CVD technology to synthesize nitrogen-doped graphene (NG) nanosheets by a NaCl template-assisted method.44 The employment of plasma-enhanced CVD (PECVD) enabled the direct growth of NG nanosheets on a recrystallized salt crystal at relatively low temperatures (600–700 °C) in a controllable fashion, thereby creating a facile production process and lowering the production cost. Fig. 5a illustrates the typical fabrication. First, the NG nanosheet is conformally grown on NaCl cages with pyridine as both carbon and nitrogen sources. Then, the salt template is dissolved in deionized water by a simple washing procedure (the SEM and TEM images of NG hollow cages shown in Fig. 5b–d). Finally, 2D NG nanosheets can be directly formed through sonication. The growth on the surface is mainly attributed to different electric field intensities and catalytic activities of the gas molecules, such as the difference between the side face and the edge. Therefore, the fragile connection parts between the different side faces are easy to break up, resulting in the formation of uniform 2D nanosheets (Fig. 5e). In addition to metal oxides and graphenes,45–47 2D metal nitrides, sulfides, selenides and phosphides (e.g., MoN, MoS2, and MoP2) can also be synthesized by virtue of salt-templated routes.48–50
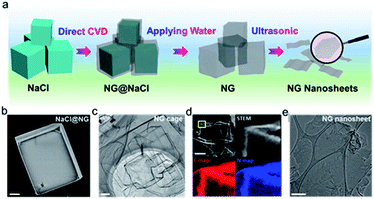 |
| Fig. 5 (a) Schematic of the salt-templated CVD growth of NG nanosheets directly on the NaCl crystal. (b) SEM image of an individual NaCl@NG powder. (c) TEM image of the NG cage. (d) STEM image and elemental maps of the NG cage. (e) TEM image of the as-obtained NG nanosheet. Reproduced with permission.44 Copyright 2019, American Chemical Society. | |
For instance, in an attempt to synthesizing 2D metal phosphides, Li et al. developed a general salt-templating strategy. The fabrication process is shown in Fig. 6. (NH4)2HPO4 is employed as a phosphorus source and mixed with metal nitrate precursors, which are then coated onto the surface of the salt template. The thus-obtained composites are annealed in a H2/Ar atmosphere to generate various metal phosphides, such as Co2P, MoP2, Ni12P5 and WP2.51
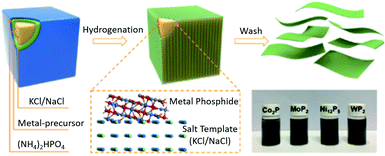 |
| Fig. 6 Synthesis process of 2D metal phosphides. Reproduced with permission.51 Copyright 2018, Royal Society of Chemistry. | |
Another feasible strategy for obtaining 2D materials lies in using melted salt as the template. In contrast to the conventional mild condition with a processing temperature lower than 900 °C, this approach requires high-temperature annealing (over 900 °C). The droplets of molten salt act as a paradigm to induce separated flat structures to construct 2D nanoplates. In this regard, Zhu et al. used a melted ZnCl2/KCl salt mixture as the porous template to grow vertically aligned graphitic carbon nanosheets and their metal carbide hybrids (WC, TaC and NbC).52 In addition, ultrathin amorphous carbon nanosheets were synthesized by a molten-salt route in a scalable manner.53
2.2.2. 3D metal foams.
3D metal foams are often used as the template to fabricate 3D nanomaterials. For example, Ni foams can guide carbon species to form a porous hierarchical architecture. Subsequently, a 3D graphene foam can be prepared throughout a simple acid etching process.54 Recently, Xing et al. have reported an effective synthesis route to attain high-crystalline g-C3N4 nanosheets by utilizing Ni foams as the confined template.55 The scheme for the formation of 2D g-C3N4 nanosheets is shown in Fig. 7. The recrystallized process of dicyandiamide on the Ni surface occurs, followed by the pyrolysis that would promote the surface-confined growth of C3N4, whilst the stacking of nanosheets are suppressed on a Ni foam. The 2D nanosheets were obtained via one-step acid etching. Taken together, the 3D metal foam is a favourable candidate due to its high catalytic activity (compared with non-metal 3D templates) and good mechanical stability to grow graphenes and/or other 2D materials. However, it is worth noting that metallic residuals and impurities could remain in target products due to any incomplete etching process of templates. Likewise, in comparison with the salt template route, the employment of acid etchants is normally environmentally unfriendly.
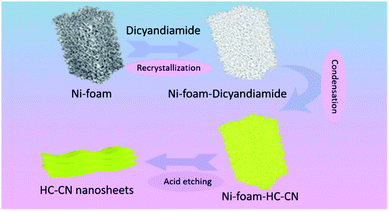 |
| Fig. 7 Schematic of the formation of 2D g-C3N4 nanosheets. Reproduced with permission.55 Copyright 2018, American Chemical Society. | |
2.2.3. 3D liquid metal alloys.
Owing to the unique structure of metallic cores and active surfaces, liquid metals and metal alloys show great potential for synthesizing 2D materials.56 Liquid metals are featured by the surface effect of “self-limiting native-oxide skin”, which was considered to be promising for preparing atomically thin materials.57 In 2017, Zavabeti et al. reported the phenomenon that the self-limiting thin oxide layer occurred naturally in the gallium-based and other liquid metal alloys. Based on the observation, different atomically thin 2D materials such as HfO2, Al2O3 and Gd2O3 were successfully achieved by manipulating different precursor elements in the alloys.58 Two principal approaches for the isolation of the surface oxide are shown in Fig. 8. The former method is called van der Waals (vdW) exfoliation, where the metal oxide layers are easily exfoliated, owing to the weak combination between the metal core and the surface skin (Fig. 8a). The latter one is more scalable, utilizing the air bubble to remove the surface oxide skin (Fig. 8b). Following this innovative work, 2D GaS,59 GaPO4,60 SnO,61 AlOOH,62 and GaN63 can be in target prepared. Nevertheless, this strategy has certain limitations, where only 2D materials that are thermodynamically stable with liquid metals can be synthesized.
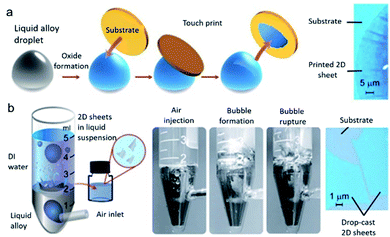 |
| Fig. 8 (a) Schematic of the van der Waals exfoliation technique. The pristine liquid metal droplet is first exposed to an oxygen-containing environment. Touching the liquid metal with a suitable substrate allows transfer of the interfacial oxide layer. An optical image is shown at the right. (b) Schematic of the gas injection method (left), photographs of the bubble bursting through the liquid metal (center), and an optical image of the resulting sheets drop-casted onto a SiO2/Si wafer (right). Reproduced with permission.58 Copyright 2017, Science. | |
2.2.4. Other 3D templates.
In addition, certain biomorphic architectures are feasible to function as templates benefiting from the 3D hierarchical configuration and enriched pore structures, such as diatomites.64–66 It is an indirect method to grow 2D materials, compared with 2D templates. Nevertheless, it exhibits some distinct advantages including high yields and low by-product concentrations. Therefore, versatile 3D templates provide a new perspective for the synthesis of 2D materials.
3. Device applications
The application sectors of 2D materials cover a wide range of fields, including energy storage and conversion, sensing, and biomedicine. We herein pay special attention to the applications in aqueous energy storages. Owing to the distinct features, including high safety (compared with traditional organic electrolytes), high rate performance (high ion mobility within aqueous electrolytes), low pollution concern and operation feasibility, aqueous-based EES devices perfectly meet the current demands for flexible and wearable electronics.4 However, the major challenge existing in this realm is the relatively unsatisfactory energy density, which stems from the undesirable electrode materials. In turn, acquisition of suitable cathode materials has become a bottleneck. In this regard, effective material design strategies, especially the abovementioned template-confined method, have been introduced to synthesize high-performance 2D materials to boost the energy density of aqueous-based EES systems. On the one hand, the ultrathin structure of the 2D materials provide a highly exposed surface area to facilitate electrolyte infiltration and ion adsorption, especially beneficial to the electric double-layer capacitive behaviour. Furthermore, various redox active species are easily decorated on the surface or layered space of 2D materials by a template-assisted method, resulting in heterojunction materials. The different components in heterostructure materials can synergistically enhance the charge storage in EES devices. On the other hand, the superior electron and ion conductivity of 2D materials is crucial for the electrode materials to promote electrochemical reactions and reduce electrochemical polarization, compared with other bulk materials. This section highlights the applications of template-confinement-derived 2D materials in supercapacitors and aqueous zinc metal batteries (zinc ion batteries and zinc air batteries). In a broader context, Table 1 summarizes the templates, obtained materials and versatile applications.
Table 1 Summary of the template-assisted materials and applicationsa
Templates |
2D materials |
Applications |
Ref. |
LIB: lithium ion battery; LIC: lithium ion capacitor; LSB: lithium-sulfur battery; SIB: sodium ion battery; PIC: potassium ion capacitor; SC: supercapacitor; ZIB: zinc ion battery; ZAB: zinc air battery; FET: field effect transistor.
|
GO |
2GO/TiO2 |
Desalination |
17
|
GO |
TiO2/ZnO/Fe2O3/SiO2 |
LIB |
18
|
GO |
ZnO/Al2O3/Mn2O3/CuO |
SC |
19
|
GO |
TixNbyOz/NixCoyOz/MnxCoyOz |
LIB |
20
|
GO |
YBa2Cu3O7−δ |
Superconducting |
21
|
Graphene |
LDH |
SC |
22
|
GO |
Au |
N/A |
23
|
MoS2 |
Pd/Pt/Ag |
Electrocatalysis |
24
|
MoS2 |
Al/Ag/Cu/Mn/Mo/Ni/Pd/Ru/Re/Zn |
N/A |
25
|
MoS2 |
MoxC-graphene |
Electrocatalysis |
26
|
rGO |
MOFS |
LIB |
30
|
Graphene |
Conductive MOFs |
SC |
31
|
MOFs |
N doped carbon |
SIB |
32
|
MOFs |
NiCo2O4 |
SC |
33
|
LDH |
Au |
Electrocatalysis |
37
|
Mg(OH)2 |
Graphene |
SC |
38
|
CuO |
alpha-Fe2O3 |
Ferromagnetism |
39
|
MoO2 |
MoS2 |
N/A |
40
|
MoS2 |
NbS2 |
Memristors |
41
|
Al2O3 |
WS2 |
FET |
42
|
NaCl |
N doped graphene |
SC |
44
|
Na2CO3 |
Carbon |
PIC |
45
|
NaCl |
Carbon |
ZAB |
46
|
NaCl |
Carbon |
LIC |
47
|
NaCl |
MoN |
SC |
48
|
ZIF-67 |
CoPO |
Electrocatalysis |
50
|
KCl/NaCl |
Co2P/MoP2/Ni12P5/WP2 |
Electrocatalysis |
51
|
ZnCl2/KCl |
Graphitic carbon |
LIB/electrocatalysis |
52
|
KCl/CaCl2 |
Amorphous carbon |
LIB |
53
|
Ni foam |
g-C3N4 |
Electrocatalysis |
55
|
Ga liquid |
HfO2/Al2O3/Gd2O3 |
N/A |
58
|
Ga liquid |
GaS |
FET |
59
|
Ga liquid |
GaPO4 |
N/A |
60
|
Tin liquid |
SnO |
FET |
61
|
Ga–Al liquid |
AlOOH |
Flux membranes |
62
|
Ga liquid |
GaN |
FET |
63
|
Diatomites |
VN/Mo2N/WN |
SC |
64
|
Diatomites |
N-doped graphene |
LSB |
65
|
Polystyrene |
MnO2/N-doped carbon |
SC |
71
|
Pitch |
g-C3N4 |
SC |
72
|
MoS2 |
Polypyrrole |
SC |
75
|
MXenes |
Polypyrrole |
SC |
76
|
MOFs |
MnOx |
ZIB |
83
|
GO |
MnO2 |
ZIB |
84
|
MOFs |
NiCo2O4 |
ZIB |
86
|
Ni foam |
Co3O4 |
ZIB |
87
|
Ni foam |
Ni–Co LDH |
ZIB |
88
|
Co(OH)2 |
MOFs |
SC |
90
|
MOF |
MnO2 |
SC |
92
|
Ni foam |
Ni3S2/Ni |
ZIB |
94
|
Ni foam |
NiO/Ni |
ZIB |
95
|
GO |
ZnO@RGO |
ZIB |
97
|
Graphene |
Zn–Al LDH |
ZIB |
98
|
NaCl |
Co3O4/Carbon |
ZAB |
112
|
MOFs |
Co–N/C/MnO2 |
ZAB |
113
|
GO |
Co3O4/Graphene |
ZAB |
114
|
FeCl3/SiO2 |
FeCo–Nx–carbon |
ZAB |
115
|
g-C3N4 |
N–P co-doped carbon |
ZAB |
115
|
3.1. Supercapacitors
Supercapacitors, featured by high power density (>10
000 W kg−1) and impressive cycling stability (>10
000 cycles), manifest unique advantages in the large-scale grid systems. Nevertheless, the main shortcoming of such systems is the mediocre energy density (<10 W h kg−1). The energy density of supercapacitors can be calculated according to the equation: E = 1/2 CV2. Therefore, it is of paramount significance to design suitable electrode materials with high capacitance for supercapacitors to realize advanced energy density.3,67 As aforementioned, the template-confined method is beneficial for producing a variety of metal oxides,68,69 nitrides,70 and heteroatom-doped carbons,71,72 which are promising candidates with pseudocapacitive features.
Typically, processing of 2D MXene flakes into hollow spheres and 3D architectures by a template method is reported by Zhao et al.73 Inspired by this work, Yu et al. reported the 2D porous MXene (Ti3C2Tx) obtained by the melamine formaldehyde template, which were employed as the ink for 2D and 3D printing of supercapacitors.74 The template-assisted strategy promoted the MXene to form an N-doped crumpled structure, showing great potentials for flexible and printable supercapacitors. The schematic of direct MXene-N ink printing is shown in Fig. 9. The N-doped porous structure can increase the surface area and conductivity to enhance ion diffusion. In terms of 2D screen printing, MXene-N micro-symmetrical supercapacitors delivered favourable areal capacitances (70.1/62.5 mF cm−2 at 10/100 mV s−1). As for 3D printing, the MXene-N ink-based device also displayed high areal capacitances of 8.2, 5.2, and 3.4 F cm−2 for three-, two-, and one-layered electrodes respectively at a scan rate of 10 mV s−1. The template-confined synthesis, when combined with printing technology, exhibits high potentials for supercapacitor applications.
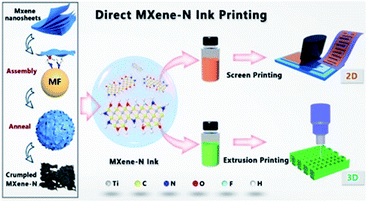 |
| Fig. 9 Schematic of direct MXene-N ink printing. Left panel: synthesis of crumpled MXene-N. Right panel: versatile MXene-N inks designed for printing, namely, screen printing of the 2D pattern (top row) and extrusion printing of the 3D architecture (bottom row). Reproduced with permission.74 Copyright 2019, Wiley-VCH. | |
It is worth mentioning that some conductive polymers can also be synthesized by the template confined method as electrode materials for supercapacitors. Tang and co-workers reported a feasible strategy to grow ultrathin polypyrrole films on MoS2 monolayers, where the MoS2 nanolayer was employed as both a self-confined template and an electrochemically active species.75 The specific capacitance of MoS2/PPy nanocomposites reached 700 F g−1 at a scan rate of 10 mV s−1 in the electrolyte of 1 M KCl. Significantly, the energy density of MoS2/PPy can harvest 83.3 W h kg−1 at a power density of 3332 W kg−1, outperforming other related studies in this field. Subsequently, Boota et al. utilized the layer spacing between titanium carbide as a reaction vessel to produce 2D polypyrrole. The Ti3C2Tx/PPy composite yielded the highest capacitance of 416 F g−1 at a scan rate of 5 mV s−1 with a durable long-term performance of 25
000 cycles.76
Taken together, the designed 2D nanomaterials via a template-confined strategy display unique advantages, such as controllable microstructure, large surface area and modulated elemental doping. Of note, in combination with emerging device fabrication technology (e.g., printing), the thus-prepared 2D electrode materials would greatly contribute to increased energy density of supercapacitors.
3.2. Batteries
The aqueous batteries have already become a research hotspot, owing to their high safety and relatively high energy density. The aqueous battery system mainly includes alkali metal ion batteries (lithium, sodium and potassium ions) and multivalent ion batteries (zinc, magnesium, aluminium and calcium ions).77 In this part, zinc metal batteries are chosen as a representative to discuss the significance of 2D materials synthesized by the templated strategy in the realm of aqueous batteries.78
3.2.1. Neutral zinc ion batteries.
Neutral electrolyte-based zinc ion batteries store energy by the intercalation/deintercalation of zinc ions in the cathode but by the dissolution/deposition of zinc ions in the anode.2 Consequently, the intercalated reaction mechanism requires fairly large layer spacings of electrode materials, rapid ion-solid phase diffusion rates and favourable structure stability. In this regard, 2D nanomaterials such as 2D van der Waals layered materials (MoS2 and VS2)79,80 and layered metal oxides (V2O5 and MnO2)79,81–83 have become attractive due to the large interlayer spacings for Zn ion insertion. However, the major concern is raised from the electrode collapse upon cycling because of the fragile structure. Recently, Guo et al. have synthesized ultrathin δ-MnO2 nanosheets by using GO as the template for aqueous Zn–MnO2 batteries.84 The as-prepared δ-MnO2 nanosheets exhibited a favourable capacity of 133 mA h g−1 at a current density of 100 mA g−1 over 100 cycles. The ultrathin morphology facilitated the uniform current distribution and improved cycle stability. Another example by Ren et al. demonstrated the usage of polystyrene latex microspheres as templates to synthesize 2D porous MnO2 nanosheets. The constructed Zn ion battery also showed a high specific capacity of 262.9 mA h g−1 at 300 mA g−1.85 Obviously, the template-confined growth strategy shows great advantages in the control of diversified hierarchical structures and accordingly enhances the electrochemical performance of neutral Zn ion batteries.
3.2.2. Alkaline zinc ion batteries.
The reaction mechanism of alkaline electrolyte-based zinc ion batteries is somewhat different from that of the neutral systems. It lies in the reversible redox reactions of hydroxyl groups on or near the surface, leading to the excellent rate performance and high energy density.86 However, its development has been obstructed by the poor conductivity of cathode materials (Co3O4,87 NiO, Ni(OH)2, and Ni–Co-LDH88,89) and inferior stability of Zn plates in the alkaline media. As for the cathode material design, MOF architectures have been reported as sacrificial templates for the target synthesis of 2D carbons and metallic-based nanosheets. The metal phosphides and selenides, after a simple annealing process, can be easily obtained. Recently, Zeng et al. have synthesized the P–NiCo2O4−x cathode material using MOF as the template, followed by annealing under NaH2PO2·H2O.86 The thus-fabricated P–NiCo2O4−x//Zn battery presented an impressive specific capacity of 361.3 mA h g−1 at a high current density of 3.0 A g−1 in an alkaline electrolyte. Briefly, the 2D MOF species, as a generic self-sacrificial template, offers great potential for the synthesis of desired cathode materials for alkaline Zn ion batteries. In particular, the adjustable metal source (Co MOFs,90 Ni MOFs,91 Mn MOFs,92 and Fe MOFs93), the composite material (from the organic ligand) and modulated doped source (N, P, S, Se, and B doping) enable MOFs to act as versatile templates. The 2D materials from those MOFs can achieve increased conductivity and ample reactive sites, targeting the construction of high-performance alkaline Zn ion batteries. Certainly, in addition to the MOFs and its derivatives, the Ni foam could serve as a self-standing template to synthesize 2D nanosheets (Ni3S2/Ni,94 NiO/Ni,95 and Co3S4/Ni96) in situ without the post-removal of the foam. Apart from the cathode materials, the template-assisted synthesized 2D material is also employed as the anode in the alkaline zinc ion battery systems. For example, Sun et al. utilized GO as a template to synthesize 2D ZnO nanosheets, achieving a high capacity of 510 mA h g−1.97 Likewise, Yang et al. reported a Zn–Al LDHs/graphene composite by utilizing GO templates, harvesting a good cyclic stability (capacity retention: 94.9% after 800 cycles) as the anode material substituting the conventional ZnO/Zn anode.98
3.2.3. Zinc air batteries.
Zinc air batteries gain merits in the high energy density and low safety concerns, which is considered as one of the most promising energy storage systems. Different from the reaction mechanisms mentioned above, it is based on surface catalytic reactions rather than solid phase ion intercalations or near-surface redox reactions.99 Therefore, the key is to design effective cathode materials, which exhibit advanced catalytic activities for both oxygen evolution reactions (OERs) and oxygen reduction reactions (ORRs). The 2D nanosheets of doped carbons (N doped,100 P doped,101 S doped,102 and B doped103) and/or metal compounds (Co3O4,104 CoS,105 CoN,106 CoP,107 Ni–FeN,108 CuCo2S4,109 Fe2O3,110 and NiCo2O4 (ref. 111)), are widely probed as OER and ORR catalysts owing to the large surface area and enriched active sites. This, in turn, possesses huge implication in zinc air batteries. In this regard, the template-confined growth strategy is a suitable solution to synthesize cathode materials for Zn–air batteries. For instance, Ding and colleagues reported the preparation of 2D porous metal–based carbon nanosheets (Co–Co3O4/C) utilizing the melted NaCl salt as the template.112 Upon high-temperature carbonization, the NaCl formed nanodroplets at 900 °C, where these nanodroplets penetrated into the interior of the carbon precursors and formed the 2D porous structure (shown in Fig. 10a). The final products were labelled as M-1 (0.2 g Co(NO3)2·6H2O), M-2 (0.4 g Co(NO3)2·6H2O) and M-3 (0.8 g Co(NO3)2·6H2O). From Fig. 10b–g, the as-derived Co–Co3O4/C nanosheets show favourable electrochemical performances, realizing high open circuit voltage, good rate performance and small polarization.
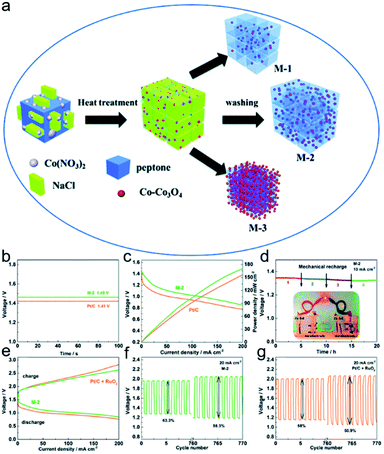 |
| Fig. 10 (a) Synthesis route of Co–Co3O4/C samples. (b) Open circuit voltage of M-2 and Pt/C primary Zn–air batteries. (c) Polarization and power density curves. (d) Long-term galvanostatic discharge of the primary Zn–air battery. (e) Charge and discharge curves of the M-2 and Pt/C + RuO2 cells. (f and g) Discharge and charge curves of current density: 20 mA cm−2, electrolyte: 6 M KOH + 0.2 M Zn(CH3COO)2. Reproduced with permission.112 Copyright 2019, Royal Society of Chemistry. | |
The salt template-assisted method aids to achieve thin 2D sheet structures, thereby enhancing the contact of electrolytes with electrodes and the rate of gas diffusion. Alongside the salt template, MOFs,113 rGO,114 silica,115 g-C3N4 (ref. 116) have also been employed as templates to grow 2D nanosheets for Zn–air batteries. The template-confined approach tends to introduce various functional defects, providing a new perspective for the design of cathode materials for Zn–air batteries.
4. Conclusion and outlook
Continuously growing attention has been paid to 2D materials to date because of their fascinating structures and properties. A template-confined route has been investigated as a simple and universal strategy to synthesize 2D materials harnessing a wide suite of merits including abundant surface-active sites, large surface areas, enlarged layer spacings, controllable doping amounts and tailored morphologies. In the foregoing sections, we summarized the recent advances in the realm of template-confined methods and the thus-derived 2D materials.
Nevertheless, there still exist several daunting challenges: (1) a relatively high cost aspect has to be maintained for the process of template production and subsequent template removal; (2) the sustainability of such a method deserves to be reconsidered, as the 2D template and 3D metal foam template are difficult to be recycled and reutilized; (3) certain templates are highly unlikely to be separated from the produced materials. For instance, it is a big task to remove the TMD or MOF templates. Interestingly, such “issues” can be useful in the in situ synthesis of heterojunctions and composites, which may lead to the realization of unique physical and chemical properties of materials.
Certainly, there is still a long way to utilize the template-assisted synthesized 2D materials to achieve higher energy density and rate performance for the aqueous EES devices. More importantly, how to take advantage of the template method and enlarge their role in energy storage systems is a question. In this respect, several solutions are proposed: (1) more attention could be paid to the employment of 2D materials in other components apart from electrodes, i.e., functional separators, especially for aqueous Zn batteries; (2) there is large scope for research in aqueous hybrid capacitors, including Zn ion hybrid capacitors, K ion hybrid capacitors and Mg ion hybrid capacitors, where the 2D materials prepared via a template-confined route might serve as suitable electrodes. Indeed, smart utilization of various templates would not only alleviate the issues of material stacking and agglomeration during synthesis but also help creating innovative architectures with desired properties. It is anticipated that the template-confined growth strategies are versatile enough to produce high-quality 2D materials for aqueous energy storage applications in a practical manner.
Conflicts of interest
There are no conflicts to declare.
Acknowledgements
The authors acknowledge the support from the National Natural Science Foundation of China (51702225) and Natural Science Foundation of Jiangsu Province (BK20170336). The authors also acknowledge the support from Suzhou Key Laboratory for Advanced Carbon Materials and Wearable Energy Technologies, Suzhou, China.
References
- S. Park, S. W. Heo, W. Lee, D. Inoue, Z. Jiang, K. Yu, H. Jinno, D. Hashizume, M. Sekino, T. Yokota, K. Fukuda, K. Tajima and T. Someya, Self-powered ultra-flexible electronics via nano-grating-patterned organic photovoltaics, Nature, 2018, 561, 516–521 CrossRef CAS PubMed.
- X. Zeng, J. Hao, Z. Wang, J. Mao and Z. Guo, Recent progress and perspectives on aqueous Zn-based rechargeable batteries with mild aqueous electrolytes, Energy Storage Materials, 2019, 20, 410–437 CrossRef.
- Y. Shao, M. F. El-Kady, J. Sun, Y. Li, Q. Zhang, M. Zhu, H. Wang, B. Dunn and R. B. Kaner, Design and mechanisms of asymmetric supercapacitors, Chem. Rev., 2018, 118, 9233–9280 CrossRef CAS PubMed.
- C. Yang, J. Chen, X. Ji, T. P. Pollard, X. Lu, C. J. Sun, S. Hou, Q. Liu, C. Liu, T. Qing, Y. Wang, O. Borodin, Y. Ren, K. Xu and C. Wang, Aqueous Li-ion battery enabled by halogen conversion-intercalation chemistry in graphite, Nature, 2019, 569, 245–250 CrossRef CAS PubMed.
- Y. Q. Li, H. Shi, S. B. Wang, Y. T. Zhou, Z. Wen, X. Y. Lang and Q. Jiang, Dual-phase nanostructuring of layered metal oxides for high-performance aqueous rechargeable potassium ion microbatteries, Nat. Commun., 2019, 10, 4292 CrossRef PubMed.
- Q. Yang, S. Cui, Y. Ge, Z. Tang, Z. Liu, H. Li, N. Li, H. Zhang, J. Liang and C. Zhi, Porous single-crystal NaTi2(PO4)3 via liquid transformation of TiO2 nanosheets for flexible aqueous Na-ion capacitor, Nano Energy, 2018, 50, 623–631 CrossRef CAS.
- J. Ming, J. Guo, C. Xia, W. Wang and H. N. Alshareef, Zinc-ion batteries: Materials, mechanisms, and applications, Mater. Sci. Eng. R, 2019, 135, 58–84 CrossRef.
- Z. Li, K. Xiang, W. Xing, W. C. Carter and Y.-M. Chiang, Reversible aluminum-ion intercalation in Prussian blue analogs and demonstration of a high-power aluminum-ion asymmetric capacitor, Adv. Energy Mater., 2015, 5, 1401410 CrossRef.
- K. Li, Y. Shao, S. Liu, Q. Zhang, H. Wang, Y. Li and R. B. Kaner, Aluminum-ion-intercalation supercapacitors with ultrahigh areal capacitance and highly enhanced cycling stability: Power supply for flexible electrochromic devices, Small, 2017, 13, 1700380 CrossRef PubMed.
- R. Raccichini, A. Varzi, S. Passerini and B. Scrosati, The role of graphene for electrochemical energy storage, Nat. Mater., 2015, 14, 271–279 CrossRef CAS PubMed.
- Y. Dou, L. Zhang, X. Xu, Z. Sun, T. Liao and S. X. Dou, Atomically thin non-layered nanomaterials for energy storage and conversion, Chem. Soc. Rev., 2017, 46, 7338–7373 RSC.
- Y. Chen, Z. Fan, Z. Zhang, W. Niu, C. Li, N. Yang, B. Chen and H. Zhang, Two-dimensional metal nanomaterials: Synthesis, properties, and applications, Chem. Rev., 2018, 118, 6409–6455 CrossRef CAS PubMed.
- R. Dong, T. Zhang and X. Feng, Interface-assisted synthesis of 2D materials: Trend and challenges, Chem. Rev., 2018, 118, 6189–6235 CrossRef CAS PubMed.
- Y. Liu, J. Goebl and Y. Yin, Templated synthesis of nanostructured materials, Chem. Soc. Rev., 2013, 42, 2610–2653 RSC.
- X. Xiao, H. Song, S. Lin, Y. Zhou, X. Zhan, Z. Hu, Q. Zhang, J. Sun, B. Yang, T. Li, L. Jiao, J. Zhou, J. Tang and Y. Gogotsi, Scalable salt-templated synthesis of two-dimensional transition metal oxides, Nat. Commun., 2016, 7, 11296 CrossRef CAS PubMed.
- X. Zhang, L. Hou, A. Ciesielski and P. Samorì, 2D materials beyond graphene for high-performance energy storage applications, Adv. Energy Mater., 2016, 6, 1600671 CrossRef.
- P. Sun, Q. Chen, X. Li, H. Liu, K. Wang, M. Zhong, J. Wei, D. Wu, R. Ma, T. Sasaki and H. Zhu, Highly efficient quasi-static water desalination using monolayer graphene oxide/titania hybrid laminates, NPG Asia Mater., 2015, 7, e162 CrossRef CAS.
- Y. Saito, X. Luo, C. Zhao, W. Pan, C. Chen, J. Gong, H. Matsumoto, J. Yao and H. Wu, Filling the gaps between graphene oxide: A general strategy toward nanolayered oxides, Adv. Funct. Mater., 2015, 25, 5683–5690 CrossRef CAS.
- P. Y. Chen, M. Liu, T. M. Valentin, Z. Wang, R. Spitz Steinberg, J. Sodhi, I. Y. Wong and R. H. Hurt, Hierarchical metal oxide topographies replicated from highly textured graphene oxide by intercalation templating, ACS Nano, 2016, 10, 10869–10879 CrossRef CAS PubMed.
- A. A. AbdelHamid, J. H. Soh, Y. Yu and J. Y. Ying, Graphene oxide-templated synthesis of ternary oxide nanosheets for high-performance Li-ion battery anodes, Nano Energy, 2018, 44, 399–410 CrossRef CAS.
- R. Boston, A. Bell, V. P. Ting, A. T. Rhead, T. Nakayama, C. F. J. Faul and S. R. Hall, Graphene oxide as a template for a complex functional oxide, CrystEngComm, 2015, 17, 6094–6097 RSC.
- D. Du, X. Wu, S. Li, Y. Zhang, W. Xing, L. Li, Q. Xue, P. Bai and Z. Yan, Remarkable supercapacitor performance of petal-like LDHs vertically grown on graphene/polypyrrole nanoflakes, J. Mater. Chem. A, 2017, 5, 8964–8971 RSC.
- V. S. Marangoni, L. D. Germano, C. C. C. Silva, E. A. de Souza and C. M. Maroneze, Engineering two-dimensional gold nanostructures using graphene oxide nanosheets as a template, Nanoscale, 2018, 10, 13315–13319 RSC.
- X. Huang, Z. Zeng, S. Bao, M. Wang, X. Qi, Z. Fan and H. Zhang, Solution-phase epitaxial growth of noble metal nanostructures on dispersible single-layer molybdenum disulfide nanosheets, Nat. Commun., 2013, 4, 1444 CrossRef PubMed.
- A. C. Domask, K. A. Cooley, B. Kabius, M. Abraham and S. E. Mohney, Room temperature van der Waals epitaxy of metal thin films on molybdenum disulfide, Cryst. Growth Des., 2018, 18, 3494–3501 CrossRef CAS.
- J. Wu, L. Ma, A. Samanta, M. Liu, B. Li, Y. Yang, J. Yuan, J. Zhang, Y. Gong, J. Lou, R. Vajtai, B. Yakobson, A. K. Singh, C. S. Tiwary and P. M. Ajayan, Growth of molybdenum carbide-graphene hybrids from molybdenum disulfide atomic layer template, Adv. Mater. Interfaces, 2017, 4, 1600866 CrossRef.
- D. Feng, T. Lei, M. R. Lukatskaya, J. Park, Z. Huang, M. Lee, L. Shaw, S. Chen, A. A. Yakovenko, A. Kulkarni, J. Xiao, K. Fredrickson, J. B. Tok, X. Zou, Y. Cui and Z. Bao, Robust and conductive two-dimensional metal−organic frameworks with exceptionally high volumetric and areal capacitance, Nat. Energy, 2018, 3, 30–36 CrossRef CAS.
- C. Liu, J. Wang, J. Wan, Y. Cheng, R. Huang, C. Zhang, W. Hu, G. Wei and C. Yu, Amorphous metal–organic framework dominated nanocomposites with both compositional and structural heterogeneity for oxygen evolution, Angew. Chem., Int. Ed., 2020, 59, 3630–3637 CrossRef CAS PubMed.
- Q. Jiang, P. Xiong, J. Liu, Z. Xie, Q. Wang, X. Yang, E. Hu, Y. Cao, J. Sun, Y. Xu and L. Chen, A redox-active 2D metal–organic framework for efficient lithium storage with extraordinary high capacity, Angew. Chem., Int. Ed., 2020, 132, 5311–5315 CrossRef.
- C. Liu, X. Huang, J. Liu, J. Wang, Z. Chen, R. Luo, C. Wang, J. Li, L. Wang, J. Wan and C. Yu, A general approach to direct growth of oriented metal–organic framework nanosheets on reduced graphene oxides, Adv. Sci., 2020, 7, 1901480 CrossRef CAS PubMed.
- H. Wu, W. Zhang, S. Kandambeth, O. Shekhah, M. Eddaoudi and H. N. Alshareef, Conductive metal-organic frameworks selectively grown on laser-scribed graphene for electrochemical microsupercapacitors, Adv. Energy Mater., 2019, 9, 1900482 CrossRef.
- L. Kong, J. Zhu, W. Shuang and X.-H. Bu, Nitrogen-doped wrinkled carbon foils derived from MOF nanosheets for superior sodium storage, Adv. Energy Mater., 2018, 8, 1801515 CrossRef.
- C. Guan, X. Liu, W. Ren, X. Li, C. Cheng and J. Wang, Rational design of metal-organic framework derived hollow NiCo2O4 arrays for flexible supercapacitor and electrocatalysis, Adv. Energy Mater., 2017, 7, 1602391 CrossRef.
- Y. Zhao, L. Hu, S. Zhao and L. Wu, Preparation of MnCo2O4@Ni(OH) core-shell flowers for asymmetric supercapacitor materials with ultrahigh specific capacitance, Adv. Funct. Mater., 2016, 26, 4085–4093 CrossRef CAS.
- H. Hu, Bu Y. Guan and X. W. Lou, Construction of complex CoS hollow structures with enhanced electrochemical properties for hybrid supercapacitors, Chem, 2016, 1, 102–113 CAS.
- L. Li, Y. Q. Zhang, X. Y. Liu, S. J. Shi, X. Y. Zhao, H. Zhang, X. Ge, G. F. Cai, C. D. Gu, X. L. Wang and J. P. Tu, One-dimension MnCo2O4 nanowire arrays for electrochemical energy storage, Electrochim. Acta, 2014, 116, 467–474 CrossRef CAS.
- L. Wang, Y. Zhu, J. Q. Wang, F. Liu, J. Huang, X. Meng, J. M. Basset, Y. Han and F. S. Xiao, Two-dimensional gold nanostructures with high activity for selective oxidation of carbon-hydrogen bonds, Nat. Commun., 2015, 6, 6957 CrossRef CAS PubMed.
- J. Yan, Q. Wang, T. Wei, L. Jiang, M. Zhang, X. Jing and Z. Fan, Template-assisted low temperature synthesis of functionalized graphene for ultrahigh volumetric performance supercapacitors, ACS Nano, 2014, 8, 4721–4729 Search PubMed.
- W. Cheng, J. He, T. Yao, Z. Sun, Y. Jiang, Q. Liu, S. Jiang, F. Hu, Z. Xie, B. He, W. Yan and S. Wei, Half-unit-cell alpha-Fe2O3 semiconductor nanosheets with intrinsic and robust ferromagnetism, J. Am. Chem. Soc., 2014, 136, 10393–10398 CrossRef CAS PubMed.
- X. Wang, H. Feng, Y. Wu and L. Jiao, Controlled synthesis of highly crystalline MoS2 flakes by chemical vapor deposition, J. Am. Chem. Soc., 2013, 135, 5304–5307 CrossRef CAS PubMed.
- B. Wang, H. Luo, X. Wang, E. Wang, Y. Sun, Y.-C. Tsai, H. Zhu, P. Liu, K. Jiang and K. Liu, Bifunctional NbS2-based asymmetric heterostructure for lateral and vertical electronic devices, ACS Nano, 2020, 14, 175–184 CrossRef CAS PubMed.
- Y. Zhang, Y. Zhang, Q. Ji, J. Ju, H. Yuan, J. Shi, T. Gao, D. Ma, M. Liu and Y. J. A. n. Chen, Controlled growth of high-quality monolayer WS2 layers on sapphire and imaging its grain boundary, ACS Nano, 2013, 7, 8963–8971 CrossRef CAS PubMed.
- G. Su, V. G. Hadjiev, P. E. Loya, J. Zhang, S. Lei, S. Maharjan, P. Dong, P. M. Ajayan, J. Lou and H. J. N. l. Peng, Chemical vapor deposition of thin crystals of layered semiconductor SnS2 for fast photodetection application, Nano Lett., 2014, 15, 506–513 CrossRef PubMed.
- N. Wei, L. Yu, Z. Sun, Y. Song, M. Wang, Z. Tian, Y. Xia, J. Cai, Y. Y. Li, L. Zhao, Q. Li, M. H. Rummeli, J. Sun and Z. Liu, Scalable salt-templated synthesis of nitrogen-doped graphene nanosheets toward printable energy storage, ACS Nano, 2019, 13, 7517–7526 CrossRef CAS PubMed.
- J. Chen, B. Yang, H. Hou, H. Li, L. Liu, L. Zhang and X. Yan, Disordered, large interlayer spacing, and oxygen-rich carbon nanosheets for potassium ion hybrid capacitor, Adv. Energy Mater., 2019, 9, 1803894 CrossRef.
- Y. Wang, L. Tao, Z. Xiao, R. Chen, Z. Jiang and S. Wang, 3D carbon electrocatalysts in situ constructed by defect-rich nanosheets and polyhedrons from NaCl-sealed zeolitic imidazolate frameworks, Adv. Funct. Mater., 2018, 28, 1705356 CrossRef.
- R. Shi, C. Han, H. Li, L. Xu, T. Zhang, J. Li, Z. Lin, C.-P. Wong, F. Kang and B. Li, NaCl-templated synthesis of hierarchical porous carbon with extremely large specific surface area and improved graphitization degree for high energy density lithium ion capacitors, J. Mater. Chem. A, 2018, 6, 17057–17066 RSC.
- X. Xiao, H. Yu, H. Jin, M. Wu, Y. Fang, J. Sun, Z. Hu, T. Li, J. Wu, L. Huang, Y. Gogotsi and J. Zhou, Salt-templated synthesis of 2D metallic MoN and other nitrides, ACS Nano, 2017, 11, 2180–2186 CrossRef CAS PubMed.
- H. Wang, C. Ji, X. Zhang, Y. Sun, Y. Wang, Q. Pan and Q. Li, Synthesis of FeS nanoparticles embedded in MoS2/C nanosheets as high-performance anode material for lithium-ion batteries, Energy Technol, 2019, 7, 1801132 CrossRef.
- G. Anandhababu, Y. Huang, D. Babu, M. Wu and Y. Wang, Oriented growth of ZIF-67 to derive 2D porous CoPO nanosheets for electrochemical-/photovoltage-driven overall water splitting, Adv. Funct. Mater., 2018, 28, 1706120 CrossRef.
- T. Li, H. Jin, Z. Liang, L. Huang, Y. Lu, H. Yu, Z. Hu, J. Wu, B. Xia and G. Feng, Synthesis of single crystalline two-dimensional transition-metal phosphides via a salt-templating method, Nanoscale, 2018, 10, 6844–6849 RSC.
- J. Zhu, K. Sakaushi, G. Clavel, M. Shalom, M. Antonietti and T. P. Fellinger, A general salt-templating method to fabricate vertically aligned graphitic carbon nanosheets and their metal carbide hybrids for superior lithium ion batteries and water splitting, J. Am. Chem. Soc., 2015, 137, 5480–5485 CrossRef CAS PubMed.
- Y. Wang, W. Tian, L. Wang, H. Zhang, J. Liu, T. Peng, L. Pan, X. Wang and M. Wu, A tunable molten-salt route for scalable synthesis of ultrathin amorphous carbon nanosheets as high-performance anode materials for lithium-ion batteries, ACS Appl. Mater. Interfaces, 2018, 10, 5577–5585 CrossRef CAS PubMed.
- K. Chen, L. Shi, Y. Zhang and Z. Liu, Scalable chemical-vapour-deposition growth of three-dimensional graphene materials towards energy-related applications, Chem. Soc. Rev., 2018, 47, 3018–3036 RSC.
- W. Xing, W. Tu, Z. Han, Y. Hu, Q. Meng and G. Chen, Template-induced high-crystalline g-C3N4 nanosheets for enhanced photocatalytic H2 evolution, ACS Energy Lett, 2018, 3, 514–519 CrossRef CAS.
- X. Xiao, H. Wang, P. Urbankowski and Y. Gogotsi, Topochemical synthesis of 2D materials, Chem. Soc. Rev., 2018, 47, 8744–8765 RSC.
- T. Daeneke, K. Khoshmanesh, N. Mahmood, I. de Castro, D. Esrafilzadeh, S. Barrow, M. Dickey and K. Kalantar-Zadeh, Liquid metals: fundamentals and applications in chemistry, Chem. Soc. Rev., 2018, 47, 4073–4111 RSC.
- A. Zavabeti, J. Z. Ou, B. J. Carey, N. Syed, R. Orrell-Trigg, E. L. H. Mayes, C. Xu, O. Kavehei, A. P. O'Mullane and R. B. Kaner, A liquid metal reaction environment for the room-temperature synthesis of atomically thin metal oxides, Science, 2017, 358, 332–335 CrossRef CAS PubMed.
- B. Carey, J. Ou, R. Clark, K. Berean, A. Zavabeti, A. Chesman, S. Russo, D. Lau, Z. Xu and Q. Bao, Wafer-scale two-dimensional semiconductors from printed oxide skin of liquid metals, Nat. Commun., 2017, 8, 14482 CrossRef CAS PubMed.
- N. Syed, A. Zavabeti, J. Ou, M. Mohiuddin, N. Pillai, B. Carey, B. Zhang, R. Datta, A. Jannat and F. Haque, Printing two-dimensional gallium phosphate out of liquid metal, Nat. Commun., 2018, 9, 3618 CrossRef PubMed.
- T. Daeneke, P. Atkin, R. Orrell-Trigg, A. Zavabeti, T. Ahmed, S. Walia, M. Liu, Y. Tachibana, M. Javaid and A. Greentree, Wafer-scale synthesis of semiconducting SnO monolayers from interfacial oxide layers of metallic liquid tin, ACS Nano, 2017, 11, 10974–10983 CrossRef CAS PubMed.
- A. Zavabeti, B. Zhang, I. Castro, J. Ou, B. Carey, M. Mohiuddin, R. Datta, C. Xu, A. Mouritz and C. McConville, Green synthesis of low-dimensional aluminum oxide hydroxide and oxide using liquid metal reaction media: Ultrahigh flux membranes, Adv. Funct. Mater., 2018, 28, 1804057 CrossRef.
- Y. Chen, K. Liu, J. Liu, T. Lv, B. Wei, T. Zhang, M. Zeng, Z. Wang and L. Fu, Growth of 2D GaN single crystals on liquid metals, J. Am. Chem. Soc., 2018, 140, 16392–16395 CrossRef CAS PubMed.
- Y. Yi, L. Yu, Z. Tian, Y. Song, Y. Shao, L. Gao, J. Sun and Z. Liu, Biotemplated synthesis of transition metal nitride architectures for flexible printed circuits and wearable energy storages, Adv. Funct. Mater., 2018, 28, 1805510 CrossRef.
- Q. Li, Y. Song, R. Xu, L. Zhang, J. Gao, Z. Xia, Z. Tian, N. Wei, M. H. Rummeli, X. Zou, J. Sun and Z. Liu, Biotemplating growth of nepenthes-like N-doped graphene as a bifunctional polysulfide scavenger for Li−S batteries, ACS Nano, 2018, 12, 10240–10250 CrossRef CAS PubMed.
- T. Guo, Y. Song, Z. Sun, Y. Wu, Y. Xia, Y. Li, J. Sun, K. Jiang, S. Dou and J. Sun, Bio-templated formation of defect-abundant VS2 as a bifunctional material toward high-performance hydrogen evolution reactions and lithium−sulfur batteries, J. Energy Chem., 2020, 42, 34–42 CrossRef.
- Z. Tian, X. Tong, G. Sheng, Y. Shao, L. Yu, V. Tung, J. Sun, R. B. Kaner and Z. Liu, Printable magnesium ion quasi-solid-state asymmetric supercapacitors for flexible solar-charging integrated units, Nat. Commun., 2019, 10, 4913 CrossRef PubMed.
- P. Liu, Y. Zhu, X. Gao, Y. Huang, Y. Wang, S. Qin and Y. Zhang, Rational construction of bowl-like MnO2 nanosheets with excellent electrochemical performance for supercapacitor electrodes, Chem. Eng. J., 2018, 350, 79–88 CrossRef CAS.
- T. Kou, B. Yao, T. Liu and Y. Li, Recent advances in chemical methods for activating carbon and metal oxide-based electrodes for supercapacitors, J. Mater. Chem. A, 2017, 5, 17151–17173 RSC.
- Y. Zhong, X. Xia, F. Shi, J. Zhan, J. Tu and H. Fan, Transition metal carbides and nitrides in energy storage and conversion, Adv. Sci., 2016, 3, 1500286 CrossRef PubMed.
- H. Liu, D. Zhai, M. Wang, J. Liu, X. Chen and Z. Zhang, Urea-modified phenol-formaldehyde resins for the template-assisted synthesis of nitrogen-doped carbon nanosheets as electrode material for supercapacitors, ChemElectroChem, 2019, 6, 885–891 CrossRef CAS.
- D. Wang, Y. Wang, Y. Chen, W. Liu, H. Wang, P. Zhao, Y. Li, J. Zhang, Y. Dong, S. Hu and J. Yang, Coal tar pitch derived N-doped porous carbon nanosheets by the in-situ formed g-C3N4 as a template for supercapacitor electrodes, Electrochim. Acta, 2018, 283, 132–140 CrossRef CAS.
- M. Zhao, X. Xie, C. Ren, T. Makaryan, B. Anasori, G. Wang and Y. Gogotsi, Hollow MXene spheres and 3D macroporous MXene frameworks for Na-ion storage, Adv. Mater., 2017, 29, 1702410 CrossRef PubMed.
- L. Yu, Z. Fan, Y. Shao, Z. Tian, J. Sun and Z. Liu, Versatile N-doped MXene ink for printed electrochemical energy storage application, Adv. Energy Mater., 2019, 9, 1901839 CrossRef.
- H. Tang, J. Wang, H. Yin, H. Zhao, D. Wang and Z. Tang, Growth of polypyrrole ultrathin films on MoS2 monolayers as high-performance supercapacitor electrodes, Adv. Mater., 2015, 27, 1117–1123 CrossRef CAS PubMed.
- M. Boota, B. Anasori, C. Voigt, M. Zhao, M. Barsoum and Y. Gogotsi, Pseudocapacitive electrodes produced by oxidant-free polymerization of pyrrole between the layers of 2D titanium carbide (MXene), Adv. Mater., 2016, 28, 1517–1522 CrossRef CAS PubMed.
- L. Dong, W. Yang, W. Yang, Y. Li, W. Wu and G. Wang, Multivalent metal ion hybrid capacitors: a review with a focus on zinc-ion hybrid capacitors, J. Mater. Chem. A, 2019, 7, 13810–13832 RSC.
- M. Song, H. Tan, D. Chao and H. Fan, Recent advances in Zn-ion batteries, Adv. Funct. Mater., 2018, 28, 1802564 CrossRef.
- H. Li, Q. Yang, F. Mo, G. Liang, Z. Liu, Z. Tang, L. Ma, J. Liu, Z. Shi and C. Zhi, MoS2 nanosheets with expanded interlayer spacing for rechargeable aqueous Zn-ion batteries, Energy Storage Materials, 2019, 19, 94–101 CrossRef.
- P. He, M. Yan, G. Zhang, R. Sun, L. Chen, Q. An and L. Mai, Layered VS2 nanosheet-based aqueous Zn ion battery cathode, Adv. Energy Mater., 2017, 7, 1601920 CrossRef.
- D. Chao, W. Zhou, C. Ye, Q. Zhang, Y. Chen, L. Gu, K. Davey and S. Qiao, An electrolytic Zn-MnO2 battery for high-voltage and scalable energy storage, Angew. Chem., Int. Ed., 2019, 58, 7823–7828 CrossRef CAS PubMed.
- M. Yan, P. He, Y. Chen, S. Wang, Q. Wei, K. Zhao, X. Xu, Q. An, Y. Shuang, Y. Shao, K. Mueller, L. Mai, J. Liu and J. Yang, Water-lubricated intercalation in V2O5.nH2O for high-capacity and high-rate aqueous rechargeable zinc batteries, Adv. Mater., 2018, 30, 1703725 CrossRef PubMed.
- Y. Fu, Q. Wei, G. Zhang, X. Wang, J. Zhang, Y. Hu, D. Wang, L. Zuin, T. Zhou, Y. Wu and S. Sun, High-performance reversible aqueous Zn-ion battery based on porous MnOx nanorods coated by MOF-derived N-doped carbon, Adv. Energy Mater., 2018, 8, 1801445 CrossRef.
- C. Guo, H. Liu, J. Li, Z. Hou, J. Liang, J. Zhou, Y. Zhu and Y. Qian, Ultrathin δ-MnO2 nanosheets as cathode for aqueous rechargeable zinc ion battery, Electrochim. Acta, 2019, 304, 370–377 CrossRef CAS.
- H. Ren, J. Zhao, L. Yang, Q. Liang, S. Madhavi and Q. Yan, Inverse opal manganese dioxide constructed by few-layered ultrathin nanosheets as high-performance cathodes for aqueous zinc-ion batteries, Nano Res., 2019, 12, 1347–1353 CrossRef CAS.
- Y. Zeng, Z. Lai, Y. Han, H. Zhang, S. Xie and X. Lu, Oxygen-vacancy and surface modulation of ultrathin nickel cobaltite nanosheets as a high-energy cathode for advanced Zn-ion batteries, Adv. Mater., 2018, 30, 1802396 CrossRef PubMed.
- X. Wang, F. Wang, L. Wang, M. Li, Y. Wang, B. Chen, Y. Zhu, L. Fu, L. Zha, L. Zhang, Y. Wu and W. Huang, An aqueous rechargeable Zn//Co3O4 battery with high energy density and good cycling behavior, Adv. Mater., 2016, 28, 4904–4911 CrossRef CAS PubMed.
- H. Chen, Z. Shen, Z. Pan, Z. Kou, X. Liu, H. Zhang, Q. Gu, C. Guan and J. Wang, Hierarchical micro-nano sheet arrays of nickel-cobalt double hydroxides for high-rate Ni-Zn batteries, Adv. Sci., 2019, 6, 1802002 CrossRef PubMed.
- Y. Zeng, Y. Meng, Z. Lai, X. Zhang, M. Yu, P. Fang, M. Wu, Y. Tong and X. Lu, An ultrastable and high-performance flexible fiber-shaped Ni-Zn battery based on a Ni-NiO heterostructured nanosheet cathode, Adv. Mater., 2017, 29, 1702698 CrossRef PubMed.
- T. Deng, Y. Lu, W. Zhang, M. Sui, X. Shi, D. Wang and W. Zheng, Inverted design for high-performance supercapacitor via Co(OH)2-derived highly oriented MOF electrodes, Adv. Energy Mater., 2018, 8, 1702294 CrossRef.
- Q. Cheng, K. Tao, X. Han, Y. Yang, Z. Yang, Q. Ma and L. Han, Ultrathin Ni-MOF nanosheet arrays grown on polyaniline decorated Ni foam as an advanced electrode for asymmetric supercapacitors with high energy density, Dalton Trans., 2019, 48, 4119–4123 RSC.
- K. Zhao, Z. Xu, Z. He, G. Ye, Q. Gan, Z. Zhou and S. Liu, Vertically aligned MnO2 nanosheets coupled with carbon nanosheets derived from Mn-MOF nanosheets for supercapacitor electrodes, J. Mater. Sci., 2018, 53, 13111–13125 CrossRef CAS.
- X. Zhang, Q. Liu, X. Shi, A. Asiri and X. Sun, An Fe-MOF nanosheet array with superior activity towards the alkaline oxygen evolution reaction, Inorg. Chem. Front., 2018, 5, 1405–1408 RSC.
- Z. Huang, X. Li, Q. Yang, L. Ma, F. Mo, G. Liang, D. Wang, Z. Liu, H. Li and C. Zhi, Ni3S2/Ni nanosheet arrays for high-performance flexible zinc hybrid batteries with evident two- stage charge and discharge processes, J. Mater. Chem. A, 2019, 7, 18915 RSC.
- Q. Chen, J. Li, C. Liao, G. Hu, Y. Fu, O. Asare, S. Shi, Z. Liu, L. Zhou and L. Mai, Ni foam supported NiO nanosheets as high-performance free-standing electrodes for hybrid supercapacitors and Ni–Zn batteries, J. Mater. Chem. A, 2018, 6, 19488 RSC.
- Si. Zhang, B. Yin, Y. Luo, L. Shen, B. Tang, Z. Kou, X. Liu, D. Lim, D. Gu, Z. Wang and H. Gong, Fabrication and theoretical investigation of cobaltosic sulfide nanosheets for flexible aqueous Zn/Co batteries, Nano Energy, 2020, 68, 104314 CrossRef CAS.
- L. Sun, Z. Yi, J. Lin, F. Liang, Y. Wu, Z. Cao and L. Wang, Fast and energy efficient synthesis of ZnO@RGO and its application in Ni−Zn secondary battery, J. Phys. Chem. C, 2016, 120, 12337–12343 CrossRef CAS.
- H. Yang, Z. Yang, X. Wen and L. Liu, The in-situ growth of zinc-aluminium layered double hydroxides on graphene and its application as anode active materials for Zn-Ni secondary battery, Electrochim. Acta, 2017, 252, 507–515 CrossRef CAS.
- H. Wang, C. Tang and Q. Zhang, A review of precious-metal-free bifunctional oxygen electrocatalysts: Rational design and applications in Zn−air batteries, Adv. Funct. Mater., 2018, 28, 1803329 CrossRef.
- L. Wang, C. Yang, S. Dou, S. Wang, J. Zhang, X. Gao, J. Ma and Y. Yu, Nitrogen-doped hierarchically porous carbon networks: synthesis and applications in lithium-ion battery, sodium-ion battery and zinc-air battery, Electrochim. Acta, 2016, 219, 592–603 CrossRef CAS.
- W. Lei, O. Deng, G. Li, Z. Cano, X. Wang, D. Luo, Y. Liu, D. Wang and Z. Chen, Two-dimensional phosphorus-doped carbon nanosheets with tunable porosity for oxygen reactions in zinc-air batteries, ACS Catal., 2018, 8, 2464–2472 CrossRef CAS.
- Y. Su, Z. Yao, F. Zhang, H. Wang, Z. Mics, E. Cánovas, M. Bonn, X. Zhuang and X. Feng, Sulfur-enriched conjugated polymer nanosheet derived sulfur and nitrogen co-doped porous carbon nanosheets as electrocatalysts for oxygen reduction reaction and zinc-air battery, Adv. Funct. Mater., 2016, 26, 5893–5902 CrossRef CAS.
- Y. Guo, P. Yuan, J. Zhang, Y. Hu, I. Amiinu, X. Wang, J. Zhou, H. Xia, Z. Song, Q. Xu and S. Mu, Carbon nanosheets containing discrete Co-Nx-By-C active sites for efficient oxygen electrocatalysis and rechargeable
Zn–Air Batteries, ACS Nano, 2018, 12, 1894–1901 CrossRef CAS PubMed.
- L. Xu, Q. Jiang, Z. Xiao, X. Li, J. Huo, S. Wang and L. Dai, Plasma-engraved Co3O4 nanosheets with oxygen vacancies and high surface area for the oxygen evolution reaction, Angew. Chem., Int. Ed., 2016, 55, 5277–5281 CrossRef CAS.
- J. Yin, Y. Li, F. Lv, M. Lu, K. Sun, W. Wang, L. Wang, F. Cheng, Y. Li, P. Xi and S. Guo, Oxygen vacancies dominated NiS2/CoS2 interface porous nanowires for portable Zn–Air batteries driven water splitting devices, Adv. Mater., 2017, 29, 1704681 CrossRef.
- Q. Xu, H. Jiang, Y. Li, D. Liang, Y. Hu and C. Li, In-situ enriching active sites on co-doped Fe-Co4N@N-C nanosheet array as air cathode for flexible rechargeable Zn-air batteries, Appl. Catal., B, 2019, 256, 117893 CrossRef CAS.
- X. Qin, Z. Wang, J. Han, Y. Luo, F. Xie, G. Cui, X. Guo and X. Sun, Fe-doped CoP nanosheet arrays: an efficient bifunctional catalyst for zinc–air batteries, Chem. Commun., 2018, 54, 7693–7696 RSC.
- Y. Fan, S. Ida, A. Staykov, T. Akbay, H. Hagiwara, J. Matsuda, K. Kaneko and T. Ishihara, Ni-Fe nitride nanoplates on nitrogen-doped graphene as a synergistic catalyst for reversible oxygen evolution reaction and rechargeable Zn-Air battery, Small, 2017, 13, 1700099 CrossRef PubMed.
- Y. Li, J. Yin, L. An, M. Lu, K. Sun, Y. Zhao, F. Cheng and P. Xi, Metallic CuCo2S4 nanosheets of atomic thickness as efficient bifunctional electrocatalysts for portable, flexible Zn-air batteries, Nanoscale, 2018, 10, 6581–6588 RSC.
- Y. Zang, H. Zhang, X. Zhang, R. Liu, S. Liu, G. Wang, Y. Zhang and H. Zhao, Fe/Fe2O3 nanoparticles anchored on Fe-N-doped carbon nanosheets as bifunctional oxygen electrocatalysts for rechargeable zinc-air batteries, Nano Res., 2016, 9, 2123–2137 CrossRef CAS.
- W. Liu, J. Bao, L. Xu, M. Guan, Z. Wang, J. Qiu, Y. Huang, J. Xia, Y. Lei and H. Li, NiCo2O4 ultrathin nanosheets with oxygen vacancies as bifunctional electrocatalysts for Zn-air battery, Appl. Surf. Sci., 2019, 478, 552–559 CrossRef CAS.
- J. Ding, S. Ji, H. Wang, H. Gai, F. Liu, B. Pollet and R. Wang, N-doped porous transition metal-based carbon nanosheet networks as a multifunctional electrocatalyst for rechargeable zinc-air batteries, Chem. Commun., 2019, 55, 2924–2927 RSC.
- Y. Chen, Y. Guo, H. Cui, Z. Xie, X. Zhang, J. Wei and Z. Zhou, Bifunctional electrocatalysts of MOF-derived Co-N/C on bamboo-like MnO nanowires for high-performance liquid- and solid-state Zn–air batteries, J. Mater. Chem. A, 2018, 6, 9716–9722 RSC.
- T. Zhou, W. Xu, N. Zhang, Z. Du, C. Zhong, W. Yan, H. Ju, W. Chu, H. Jiang, C. Wu and Y. Xie, Ultrathin cobalt oxide layers as electrocatalysts for high-performance flexible Zn–air batteries, Adv. Mater., 2019, 31, e1807468 CrossRef PubMed.
- S. Li, C. Cheng, X. Zhao, J. Schmidt and A. Thomas, Active salt/silica-templated 2D mesoporous FeCo-Nx-carbon as bifunctional oxygen electrodes for zinc-air batteries, Angew. Chem., Int. Ed., 2018, 57, 1856–1862 CrossRef CAS PubMed.
- J. Ren, G. Yuan, C. Weng, L. Chen and Z. Yuan, Hierarchically porous heteroatoms-doped Vesica-like carbons as highly efficient bifunctional electrocatalysts for Zn–air batteries, ChemCatChem, 2018, 10, 5297–5305 CrossRef CAS.
|
This journal is © The Royal Society of Chemistry 2020 |