DOI:
10.1039/D0NA00242A
(Paper)
Nanoscale Adv., 2020,
2, 3209-3221
Synergetic effects of thymoquinone-loaded porous PVPylated Fe3O4 nanostructures for efficient pH-dependent drug release and anticancer potential against triple-negative cancer cells†
Received
28th March 2020
, Accepted 3rd June 2020
First published on 3rd June 2020
Abstract
Porous iron oxide nanostructures have attracted increasing attention due to their potential biomedical applications as nanocarriers for cancer and many other therapies as well as minimal toxicity. Herbal anti-cancer agent thymoquinone loaded on Fe3O4 nanoparticles is envisaged to offer solution towards cancer treatment. The purpose of the present study was to investigate the efficacy of thymoquinone-loaded PVPylated Fe3O4 magnetic nanoparticles (TQ-PVP-Fe3O4 NPs) against triple-negative breast cancer (TNBC) cells. The porous PVPylated Fe3O4 NPs were prepared by a simple solvothermal process, whereas the thymoquinone drug was loaded via the nanoprecipitation method. Fourier transform infrared (FTIR) spectroscopic analysis confirmed the molecular drug loading, and surface morphological observation further confirmed this. The quantity of thymoquinone adsorbed onto the porous PVPylated Fe3O4 NPs was studied by thermogravimetric analysis (TGA). The positive surface charge of TQ-PVP-Fe3O4 NPs facilitates the interaction of the NPs with cancer (MDA-MB-231) cells to enhance the biological functions. In addition, the anticancer potential of NPs involving cytotoxicity, apoptosis induction, reactive oxygen species (ROS) generation, and changes in the mitochondrial membrane potential (ΔΨm) of TNBC cells was evaluated. TQ-PVP-Fe3O4 NP-treated cells effectively increased the ROS levels leading to cellular apoptosis. The study shows that the synthesized TQ-PVP-Fe3O4 NPs display pH-dependent drug release in the cellular environment to induce apoptosis-related cell death in TNBC cells. Hence, the prepared TQ-PVP-Fe3O4 NPs may be a suitable drug formulation for anticancer therapy.
1. Introduction
Cancer is one of the most prominent diseases caused by abnormal growth of cells and still the major public health problem with growing incidences and mortality rate. The available chemotherapeutic drugs are widely used to treat only the final stage, and in addition, they induce numerous side effects.1,2 Therefore, there is a critical need for the development of an innovative new drug delivery system with desirable biocompatibility to treat cancer with no or minimal adverse effects.3 Loading of therapeutic agents on nanoparticles (NPs) via appropriate surfactants or linkers might be a possible solution to effectively enhance the drug delivery to the specific site.4 Herein, magnetic Fe3O4 NPs with superparamagnetic properties possess numerous benefits due to their biocompatibility and less toxicity. Furthermore, these magnetic NPs have preferable features such as water solubility, colloidal stability, high yields, cost effectiveness and eco-friendliness.5 Moreover, they have the ability to transport and deliver the payloads in the desired sites using surface-exposed magnetic field and diminish the toxicity towards healthy tissue per cells.6 Similarly, the monodisperse Fe3O4 NPs have an affinity to accumulate specifically in cancer tissues per cells.
During preparation, pure Fe3O4 NPs aggregate due to the strong magnetic interaction between the particles. Besides, the lack of functional groups on their surface limits their biological applications.7 Hence, the magnetic NPs require possible surface modifications to become targeted drug delivery systems.8,9 Biocompatible polymers such as polyvinylpyrrolidone (PVP), dextran, polyethylene glycol (PEG), and chitosan can be used to surface functionalize the magnetic particles, which can serve as drug carriers capable of releasing the loaded drugs or molecules to the specific site.10,11 Among those, PVP is a widely used polymer due to its effective usage towards clinical applications especially for pH-dependent drug release and is considered as a more favorable biocompatible polymer for loading novel drugs.12 Thereby, in the present study, we preferred to use this polymer for the surface encapsulation of Fe3O4 nanoparticles for carrying anticancer drugs.
Thymoquinone (2-isopropyl-5-methylbenzo-1,4-quinone) is a naturally occurring active herbal molecule and a constituent of volatile oil derived from the Nigella sativa seeds, which is used for treating many diseases.13,14 It also possesses other beneficial activities such as antibacterial, anti-inflammatory, antidiabetic, antioxidant, analgesic, anti-ulcerogenic, anti-carcinogenic, anti-arthritic, anti-neoplastic, anti-mutagenic and antitumor functions via different mechanisms.15–17 Moreover, it enhances the activities of superoxide dismutase (SOD), catalase, glutathione, glutathione transferase and quinone reductase due to their antioxidant properties.18,19 Besides, the antitumor effects of thymoquinone on blood, lungs, breast, pancreas, colon, prostate, bone, brain, skin, ovaries, head and neck, liver, cervix and liver are reported in the literature.17,20 In addition, thymoquinone shows manifold functions; moreover, the mechanism depends on cellular microenvironments. It has several functional activities against cancer cells including cell cycle arrest, DNA damage, ROS generation, preventing NFκB activation and induced cellular apoptosis.14,21 In addition, in breast cancer cells it increases the cell cycle arrest due to their direct interaction with phases of G0/G1 and G2/M and induces apoptosis via the activation of the caspase pathway.22 Further, it is employed in in vivo animal models for the treatment of acute respiratory distress syndrome and also considered to be a potential therapeutic agent for inhibiting tumor angiogenesis and tumor growth.23,24 Thymoquinone also induces erythrocyte oxidative stress and haematological alterations during colon cancer promotion.24,25 The active effects of thymoquinone on the improvement of targeting cell signaling and survival pathways to resist the cancer cell growth as well as to show better bioactivities were confirmed.
However, the thymoquinone drug has limitations such as poor performance with regard to aqueous solubility, biocompatibility and cell or tissue targeted activity, which hamper its therapeutic use. Therefore, the present study focuses on the development of surface-modified porous magnetic nanoparticles carrying thymoquinone for effective anticancer applications overcoming the above limitations.
In this study, the porous PVPylated Fe3O4 magnetic nanoparticles were prepared by a solvothermal process and the herbal-based thymoquinone drug was loaded via the nanoprecipitation method. This porous magnetic nanocarrier possibly improves the drug loading and releases the drug under acidic conditions prevailing in the tumor cellular environment. The physico-chemical, surface and morphological properties of the formulated pure Fe3O4 NPs and TQ-PVP-Fe3O4 NPs were discussed. Furthermore, the anticancer-associated cellular functions of these TQ-PVP-Fe3O4 NPs were studied in MDA-MB-231 (Scheme 1). The obtained results clearly indicated the ability of the prepared TQ-PVP-Fe3O4 NPs to display effective nanodrug delivery functions in cultured MDA-MB-231 cells and significantly improve the anticancer efficacy by inducing apoptosis-related factors. Hence, we envision that the features of TQ-PVP-Fe3O4 NPs may potentially enable them to serve as magnetic biomaterials for future cancer treatment.
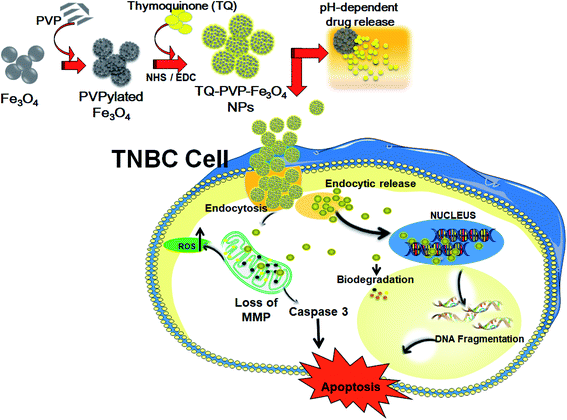 |
| Scheme 1 Schematic of the formation and synthesis of pure Fe3O4, PVPylated Fe3O4, TQ-PVP-Fe3O4 NPs and their interaction and drug delivery behavior against triple-negative breast cancer cell line model. | |
2. Experimental
2.1. Preparation of PVPylated Fe3O4 NPs
The pure Fe3O4 and PVPylated Fe3O4 NPs were formulated according to earlier reports.26,27 Anhydrous ferric chloride (FeCl3), urea and potassium hydroxide (KOH) were used as an initial precursor and base medium. Ethylene glycol (EG) and polyvinylpyrrolidone K-30 (PVP) were employed as the solvent and surfactant, respectively. In the typical solvothermal synthesis, 2.5 mM FeCl3 was dissolved in 40 mL of EG. Then, 5 mM KOH and 0.5 mM urea were dissolved in 30 mL of EG. These two solutions were mixed well with continuous stirring at ambient temperature for 1 h to obtain a homogeneous solution. Following this, a required amount of PVP was added to the homogeneous solution and again stirred at room temperature for 30 min. The final solution was transferred into a 75 mL Teflon-lined stainless steel autoclave and heated at 210 °C for 15 h. Once the reaction was completed, a black suspension obtained was allowed to cool down at room temperature. The resultant black precipitates were collected using a strong external magnet and washed several times with deionized (DI) water and ethanol to remove impurities and unbound molecules. The resultant PVPylated Fe3O4 NPs were dried in a vacuum oven at 60 °C overnight.
2.2. Preparation of thymoquinone-loaded Fe3O4 NPs
TQ-PVP-Fe3O4 NPs were synthesised by a previously reported nanoprecipitation method with slight modifications.26 Briefly, 200 mg PVPylated Fe3O4 NPs were suspended in 40 mL DI water and ultra-sonicated to acquire homogeneously dispersed particles. The pre-determined concentrations of 1-ethyl-3[3-dimethylaminopropyl]carbodiimide hydrochloride (EDC) and N-hydroxysuccinimide (NHS) were added and allowed for further ultra-sonication. Then, a required amount of thymoquinone was added and stirred at room temperature for 24 h. Finally, the resultant black precipitate was washed with DI water to remove the unloaded drug molecules. At the end of the process, TQ-PVP-Fe3O4 NPs were collected using a strong magnet applied externally and NPs were further dried in a vacuum oven.
2.3. Characterization
The phase purity and crystallinity of the pure Fe3O4, PVPylated Fe3O4 and TQ-PVP-Fe3O4 NPs were studied by X-ray diffraction (XRD, model D5005D, Germany). The chemical structure, functional groups and thymoquinone loading on PVPylated Fe3O4 NPs were analysed by Fourier transform infrared (FTIR) spectroscopy (FITR, Jasco Tensor 27, Japan). The surface micrograph of pure Fe3O4, PVPylated-Fe3O4 and TQ-PVP-Fe3O4 NPs were viewed using a field emission scanning electron microscope (FESEM, Quanta-250 FEG, Germany) with line scanning using an energy-dispersive X-ray spectrometer (EDX, Bruker, Germany) and transmission electron microscope (TEM, JEOL JEM-1200, Japan). The surface charge of magnetic NPs was measured using a zeta potential analyzer (Nicomp Zetasizer 380ZLS, USA). The weight loss and thermal stabilities of the pure Fe3O4, PVPylated-Fe3O4 and TQ-PVP-Fe3O4 NPs were measured by thermogravimetric analysis (TGA) under an oxygen gas flow (TA-TGA Q-500, TA Instrument, USA). A vibrating sample magnetometer (VSM, EV X model, USA) was used to measure the superparamagnetic behaviour of the pure Fe3O4 and drug-loaded Fe3O4 NPs at room temperature. The specific surface area, pore volume and pore size distribution of PVPylated Fe3O4 NPs were measured using a Brunner–Emmett–Teller surface area analyzer (BET, Microtarc, BELSORP-max, Japan).
2.4. MTT assay
The methyl thiazolyl tetrazolium (MTT) bromide assay was used to measure the cell viability in pure Fe3O4, PVPylated Fe3O4 and TQ-PVP-Fe3O4 NP-treated TNBC (MDA-MB-231) cells. Briefly, cultured cells were seeded in a 96-well plate at a density of 1 × 104 cells per well and incubated overnight. Then, these cells were treated with different concentrations of the prepared TQ-PVP-Fe3O4 NPs for 24 h. Then, the cells were treated with 100 μL per well of MTT solution (5 mg mL−1 concentration) and kept for incubation at 37 °C under dark conditions for another 4 h. The purple-colored formazan crystals produced from viable cells were dissolved in 100 μL of dimethyl sulfoxide. The absorbance was measured at 620 nm using a multi-well plate reader, and the observed values were expressed as a percentage relative to the respective control group.
2.5. Fluorescence microscopic analysis
By fluorescence microscopic analysis, the cellular internalization of NPs with cancer cells at different time points of incubation was studied. Briefly, RITC (rhodamine-B isothiocyanate)-labelled TQ-PVP-Fe3O4 NPs were treated and the level of internalization was quantified by fluorescence microscopy. Besides, rhodamine 123 (Rh 123)- and DAPI-stained cells were analyzed for the induction of apoptosis in cells, for which the MDA-MB-231 cells were cultured in 6-well plates at 37 °C with 5% CO2 for 48 h. Then, the cells were treated with TQ-PVP-Fe3O4 NPs with different time intervals (0, 1, 2, 4 and 8 h). Following this, the medium was removed, and the NP-treated cells were gently washed twice with phosphate-buffered saline (PBS) and fixed in 3% paraformaldehyde for 20 min. The cells were re-washed with PBS and stained with 10 μg mL−1 4′,6-diamidino-2-phenylindole (DAPI) at 37 °C in a dark place for 20 min. Consequently, the DAPI-stained cells were treated with 10 μg mL−1 Rh-123 at 37 °C for 30 min in the darkness. The cells were then washed with methanol and air dried and investigated for changes in ΔΨm at an appropriate wavelength by fluorescence microscopy. The assessment of DNA damage in nuclear materials was performed by DNA fragmentation analysis using agarose gel electrophoresis.8
2.6. Assessment of reactive oxygen species (ROS)
By 2′,7′-dichlorfluorescein-diacetate (DCFH-DA) staining, the intracellular ROS production was analyzed using 2′,7′-dichloroflorescein (DCF) signals from the dying cells. These observed DCF (green) fluorescence intensity was relatively proportional to the quantity of ROS generated from the dead cells. Briefly, the cells cultured in a six-well plate for 24 h were treated with TQ-PVP-Fe3O4 NPs at dose-dependent concentrations (20, 40, 60 and 80 μg mL−1). Then, these cells were again washed with ice-cold PBS and incubated with DCFH-DA (50 μM in a final concentration) at 37 °C in the darkness for 30 min. Further, the cells were washed twice and sustained in 1 mL of PBS. ROS production was assessed using a fluorescence microscope at emission and excitation wavelengths of 530 nm and 488 nm, respectively, depending on the intensity of fluorescence as compared to the non-treated cells.
2.7. Statistical analysis
Data analyses were performed by Student's t test or one-way ANOVA using the GraphPad Prism 6.0 software. The error bars presented the mean ± standard deviation (SD) of three independent measurements (n = 3).
3. Results and discussion
The fabrication of TQ-PVP-Fe3O4 NPs involves three steps as depicted in Scheme 1. Primarily, the ferric chloride decomposes at high temperatures (210 °C) in a polyol medium, which results in the generation of Fe species that exclusively reacts with KOH in the closed reaction system to form monodisperse spherical Fe3O4 NPs. Similarly, the self-assembly of the primary seeded particles influences the building blocks of monodisperse porous shape by tuning homogeneous nucleation via in situ addition of PVPylated polymers. The in situ surface modification could improve water solubility, prolong the circulation time and impart a stealth-shielding shell. Further, the surfaces of PVPylated Fe3O4 NPs were modified with the active site by addition of NHS and EDC via ultra-sonication. Accordingly, the amino and carboxyl groups were used to impart to the surface the hydrophilic nature and tendency to encapsulate drug molecules.26 Finally, the chosen drug thymoquinone (TQ) was uniformly loaded into the porous PVPylated Fe3O4 NPs via physical adsorption by the nanoprecipitation method to form TQ-PVP-Fe3O4 NPs. These TQ-PVP-Fe3O4 NPs were further studied to investigate their anticancer potentials in triple-negative breast cancer cells, and the interaction of NPs with cancer cells is presented in Scheme 1.
3.1. Structural characterization analysis
Powder X-ray diffraction (XRD) was used for evaluating the crystal structure and phase purity of Fe3O4, PVPylated Fe3O4 and TQ-PVP-Fe3O4 NPs (Fig. 1a). The obtained diffraction peaks of the samples were directly indexed to the cubic phase of Fe3O4 standards (JCPDS#: 89-3854) without impurities.27,28 Scherer's formula was used to calculate the average crystalline size. The crystalline size of Fe3O4 NPs was approximately 21 nm, whereas the size of the particles was decreased to 18 and 16 nm for PVPylated Fe3O4 and TQ-PVP-Fe3O4 NPs, respectively. Moreover, it was found that the intensity of the corresponding XRD peaks decreased in PVPylated and TQ-PVP-Fe3O4 NPs due to the presence of amorphous materials (polymer/drugs) on their surface of Fe3O4 NPs. The corresponding XRD peaks did not show any induced peak shift and phase transition during the addition (in situ) of polymers or drug-loaded Fe3O4 NPs.
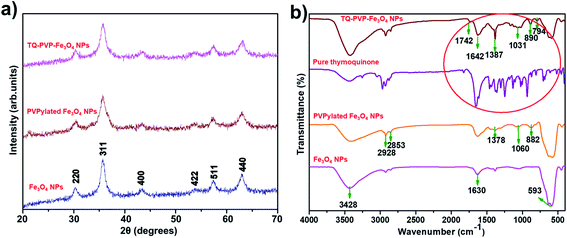 |
| Fig. 1 (a) X-ray diffraction patterns of pure Fe3O4, PVPylated Fe3O4 and TQ-PVP-Fe3O4 NPs and (b) Fourier transform infrared spectra of pure Fe3O4, PVPylated Fe3O4, pure thymoquinone and TQ-PVP-Fe3O4 NPs. The circle indicates the corresponding thymoquinone FTIR peak matching with TQ-PVP-Fe3O4 NPs. | |
Further, the functional group and chemical structure of pure Fe3O4, PVPylated Fe3O4, pure thymoquinone and TQ-PVP-Fe3O4 NPs were analyzed by FTIR spectroscopy (Fig. 1b). It was found that pure Fe3O4 NPs exhibited strong and weak absorption peaks at 593 cm−1 and 466 cm−1 attributed to the Fe–O–Fe stretching vibration of the spinel structure. These absorption peaks confirmed the distribution of Fe ions in both octahedral and tetrahedral sites in the formation of Fe3O4 NPs.27,28 Similarly, PVPylated Fe3O4 and TQ-PVP-Fe3O4 NPs showed the Fe–O bond in a slight shift towards lower wavenumber and sharp intensity peaks due to chemisorption of organic molecules.29 In PVPylated Fe3O4 NPs, the broad peaks at 882 cm−1 and 1060 cm−1 could be ascribed to the CH2 rocking and C–H stretching vibrations. In addition, the sharp intense peak at 1632 cm−1 represents the C
O stretching vibration, which further confirmed the successful wrapping of PVPylated and Fe3O4 NPs.30 Moreover, the peaks at 2853 cm−1 and 2928 cm−1 could be assigned to the symmetric and asymmetric vibrations of –CH2 molecules, respectively. The FTIR spectrum for pure thymoquinone has major peaks in the range of 600–1800 cm−1, which substantiated the presence of carboxyl, hydroxyl, amide and aromatic groups.31 The prominent peaks at 1031 cm−1 and 890 cm−1 could be assigned to the C
O stretching and C–H bending vibrations of the aromatic group, confirming the presence of TQ-PVP-Fe3O4 NPs. The obtained peaks at 1387 cm−1 and 1642 cm−1 could be assigned to the C–N stretching vibration of the amide group and the C
O stretching vibration of the carboxylic group, which closely matched with those of pure thymoquinone. Hence, it was confirmed that these obtained prominent peaks represent the successful loading of thymoquinone drug into PVPylated Fe3O4 NPs. In addition, all samples exhibited a broad peak at 3400–3450 cm−1, which could be assigned to the O–H stretching vibration of hydroxyl groups. Thus, the FTIR spectral results confirmed the formation of the metal-oxide and the loading of drug molecules onto the surface of Fe3O4 NPs.
3.2. Field emission scanning electron microscopic (FESEM) analysis
The FESEM analysis of Fe3O4, PVPylated Fe3O4 and TQ-PVP-Fe3O4 NPs was performed at various magnifications, as displayed in Fig. 2a–i. The pure Fe3O4 NPs shown in Fig. 2a–c exhibit monodisperse agglomeration-free spherical structures with a smooth surface in the size range of 30–50 nm, which is clearly visible in highly magnified images. Due to the in situ addition of PVP, the NPs were shattered into small-sized nanocrystals and compactly packed with magnetic dipole interaction to form monodisperse porous hierarchical spherical structures, as represented in Fig. 2d–f. The size of the porous spherical structures was determined to be >100 nm with a number of ultra-small pores and voids. These pores were produced due to the uniform nucleation growth of organic–inorganic particles.32 Thus, the higher surface energy would implement the aggregation of smaller crystals to form hierarchal nanostructures due to the Ostwald ripening process.33 The nitrogen adsorption–desorption isotherm confirmed that the specific surface area, average pore size and pore volume of PVPylated Fe3O4 NPs (as shown in ESI Fig. S1†) were 68.70 m2 g−1, 5.27 nm and 0.0907 cm3 g−1, respectively. The FESEM image of TQ-PVP-Fe3O4 NPs is shown in Fig. 2g–i. The thymoquinone drug molecules were also uniformly loaded in porous nanostructures, and it was highlighted in the micrograph (Fig. 2i). The high surface area and nanometric pore size of PVPylated Fe3O4 could stimulate high thymoquinone drug loading via physical adsorption mechanism. Accordingly, the results of TQ-PVP-Fe3O4 NPs did not show any noteworthy changes in the shape or size when the drug was embedded into Fe3O4 NPs. This indicated that magnetic NPs did not show any significant changes such as dissolution or growth of nanocrystals during the drug loading or encapsulation process.34
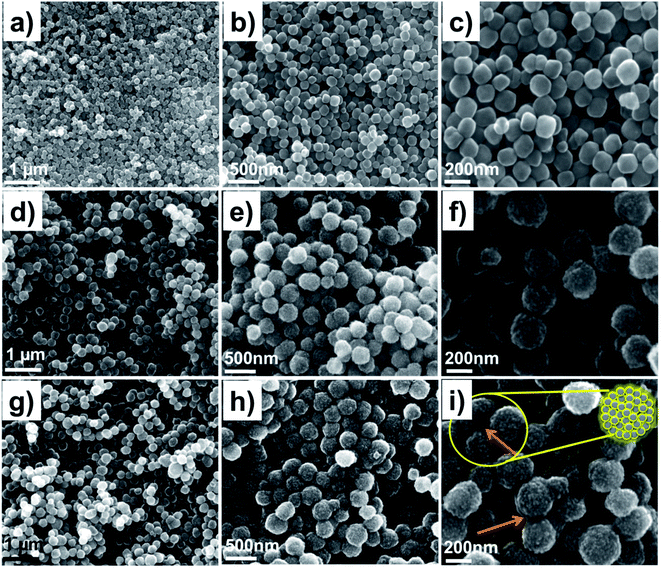 |
| Fig. 2 Field emission scanning electron microscopic (FESEM) images of low and high magnifications for (a–c) pure Fe3O4, (d–f) PVPylated Fe3O4 and (g–i) TQ-PVP-Fe3O4 NPs (the inset in (i) represents the schematic diagram of thymoquinone loading effects on PVPylated Fe3O4 NPs). | |
A transmission electron microscope (TEM) was further used to confirm the morphologies of Fe3O4, PVPylated Fe3O4 and TQ-PVP-Fe3O4 NPs, as shown in Fig. 3a–c. The pure Fe3O4 NPs are monodisperse and spherical in shape in the size range of ∼30–50 nm (Fig. 3a). Adding a structure directing agent, PVP, the monodisperse porous hierarchical spherical structures without agglomeration are clearly displayed in Fig. 3b. This porous nanostructure can adsorb more quantity of the thymoquinone drug (Fig. 3c) due to nanosized pore volume (Fig. S2†). The TEM morphologies further confirmed the successful thymoquinone loading in PVPylated Fe3O4 NPs.
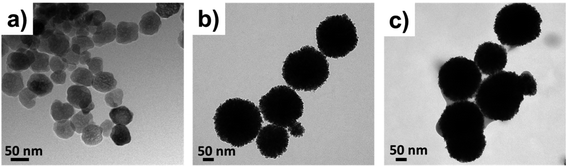 |
| Fig. 3 Transmission electron microscopic (TEM) images of (a) pure Fe3O4, (b) PVPylated Fe3O4 and (c) TQ-PVP-Fe3O4 NPs. | |
3.3. Analysis of magnetic properties
The magnetic properties of pure Fe3O4, PVPylated Fe3O4 and TQ-PVP-Fe3O4 NPs were studied using a vibrating sample magnetometer at room temperature with an applied magnetic field of 2T (Fig. 4). The absence of hysteresis loops with negligible coercivity and remanence implied that pure Fe3O4 NPs were superparamagnetic and did not alter the behavior of either PVPylated Fe3O4 or the loading of thymoquinone to their surface. The saturation magnetization (Ms) of pure Fe3O4 NPs was found to be 61 emu g−1 and the Ms value was slightly lower than the bulk counterpoint of 92 emu g−1.27 The energy of superparamagnetic particles in an applied field was directly proportional to their size via the number of magnetic molecules in a single magnetic domain. Moreover, if the energy of particles were compared with thermal energy, thermal fluctuations significantly reduce the total magnetic moments in a given field.35 This might be a reason for decreasing the saturation magnetization of pure NPs by reducing the size when compared to bulk counterpoints. The Ms value was decreased to 48 emu g−1 for PVPylated Fe3O4 NPs. The presence of non-magnetic materials in Fe3O4 NPs decreased the Ms value due to the spin canting effect and smaller particle sizes.5 In the case of TQ-PVP-Fe3O4 NPs, the Ms value was further decreased to 30 emu g−1. The strong binding of amorphous thymoquinone drug shielded on the Fe3O4 NPs might be decreased in the effective magnetic moment due to an increase in the surface spin disorientation.36 Therefore, the chemisorption of the non-magnetic layer quenched the Ms values and further confirmed the surface entrapment of the magnetic NPs. These results confirm that the changes in magnetic properties strongly depend on the size, shape and surface effects of Fe3O4 NPs.
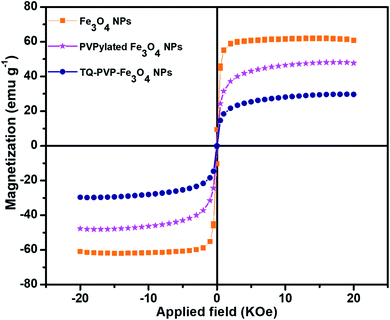 |
| Fig. 4 Room temperature hysteresis loops for pure Fe3O4, PVPylated Fe3O4 and TQ-PVP-Fe3O4 NPs. | |
3.4. Zeta potential measurements
The stability and dispersibility of Fe3O4 NPs depend on their surface chemistry, and it was studied by zeta potential analysis. Generally, the surface charge polarity of Fe3O4 NPs was changed by de-protonation and protonation effects, according to the following chemical equations.37
Fe–OH + H+ ↔ Fe–OH2+ [protonation] |
Fe–OH ↔ Fe–O− + H+ [de-protonation] |
Based on the above reaction principle, the surface charges of Fe3O4 NPs were analyzed and the interaction of bio-molecules was studied. Thus, it was found that PVPylated Fe3O4 NPs showed negative surface charge (−24 mV) due to deprotonated surface effects, and TQ-PVP-Fe3O4 NPs exhibited a positive surface charge of 6.1 mV (Fig. 5a). The change in surface charge confirms the strong interaction between the drug and Fe3O4 NPs. Moreover, it was well reported that the positively charged nanocarriers could easily bind to the negatively surface charged plasma membrane and gradually increase TQ-PVP-Fe3O4 NP uptake rates due to the electrostatic process.38 Thus, the surface charge changed from positive to negative surface, confirming the successful loading of thymoquinone drug into PVPylated Fe3O4 NPs.
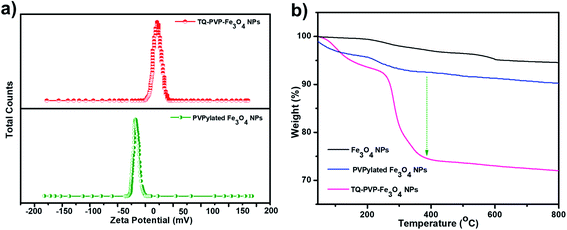 |
| Fig. 5 (a) Zeta potential of PVPylated Fe3O4 and TQ-PVP-Fe3O4 NPs and (b) thermogravimetric measurements of pure Fe3O4, PVPylated Fe3O4 and TQ-PVP-Fe3O4 NPs. | |
3.5. Thermal analysis
The amount of organic molecules adsorbed onto the surface of pure Fe3O4, PVPylated Fe3O4 and TQ-PVP-Fe3O4 NPs were quantified by thermogravimetric analysis (TGA) (Fig. 5b). The weight loss below 200 °C of all samples represents the desorption of water molecules. There was no significant weight loss with the increase in temperature, and this confirmed the high stability of pure Fe3O4 NPs. Upon addition of PVP to Fe3O4 NPs, slight weight loss (4.1%) was observed above 200 °C due to the decomposition of PVP molecules, and this observation was reported previously.39 TQ-PVP-Fe3O4 NPs show two distinct weight losses corresponding to the strong binding of the thymoquinone drug and water molecules. The first weight loss of 7% was observed at a temperature of 50–250 °C due to the endothermic loss of surface hydroxyl groups40 and the presence of PVP. Upon increasing the temperature above 250 °C, the second weight loss was obtained from the degradation of thymoquinone drug molecules. The high percentage of the weight loss was observed due to the strong wrapping of the drug as substantiated by the observation of 18.3% of thymoquinone drug loading in PVPylated Fe3O4 NPs. These results clearly indicated that thymoquinone could stably be loaded into Fe3O4 NPs. Finally, increasing the temperature above 400 °C resulted in no weight loss due to the strong Fe–O bond force constant, and therefore, more energy was required to effect the fracture of NPs.
3.6. Drug release profiles
The drug release behaviour under different pH conditions depends on the interior force between NPs and drug, size, surface behaviour, rate of dehydration and hydration of polymers. The in vitro drug release activity of TQ-PVP-Fe3O4 NPs was analysed in a phosphate buffer solution (pH 7.4) at a temperature of 37 °C to maintain the experimental condition similar to body fluids. The drug releasing profiles of TQ-PVP-Fe3O4 NPs were analysed by different pH conditions (4.6, 5.3 and 7.4). The two stages of drug release profiles were demonstrated such as early burst release under the basic condition and further fast release rate under acidic pH conditions, respectively. The thymoquinone release rate was primarily fast and becoming slower in extended time points (18 h). Initially, 26% of thymoquinone was released gradually (18 h) at pH 7.4. When decreasing the pH to 5.3 and 4.6, the drug release rate was rapid under acidic conditions. The thymoquinone release rate gradually increased and reached a maximum value of 74.5% and 85% in 18 h under two different acidic pH conditions (5.3 and 4.6), respectively (Fig. 6). In the acidic pH, the porous PVPylated Fe3O4 is exposed to acid etching, resulting in the expansion of pores that accelerates sustained drug release.41 This pore expansion is attributed to high drug release in an acidic environment, which could be due to the diffusion and swelling42,43 of porous PVPylated Fe3O4 nanocarriers. Therefore, the thymoquinone release rate was attained efficiently under acidic conditions than under basic conditions. In addition, the in vitro drug release was depending not only on the pH-triggered release behaviour but also on the physicochemical properties of PVPylated Fe3O4 NPs.
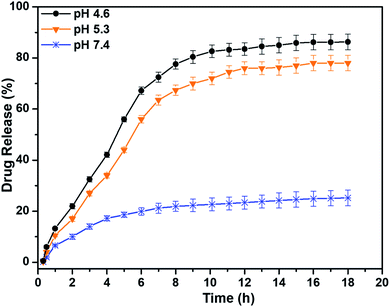 |
| Fig. 6 Drug release profiles of TQ-PVP-Fe3O4 NPs under various pH conditions. The obtained values are expressed as mean ± SD (n = 3) of triplicate measurements. | |
3.7.
In vitro anticancer analysis of TQ-PVP-Fe3O4 NPs
The MTT assay showed the cytotoxic effects of control, PVPylated Fe3O4, pure thymoquinone and TQ-PVP-Fe3O4 NPs in a chosen time point (24 h) against breast cancer (MDA-MB-231) cells (Fig. 7a). The cell viability percentages of TQ-PVP-Fe3O4 NP-treated cells were reduced as compared to that of control cells (untreated and drug-unloaded NPs). It was observed that the maximum concentrations of PVPylated Fe3O4 had no significant cytotoxicity in the cancer cells, and this confirmed the biocompatible property of NPs. The obtained inhibitory concentrations (IC50) were 50 μg mL−1 for TQ-PVP-Fe3O4 NPs. This growth inhibition caused by TQ-PVP-Fe3O4 NPs was higher than that caused by pure thymoquinone due to the strong electrostatic interaction between the positively charged TQ-PVP-Fe3O4 NPs and the negatively charged MDA-MB-231 cell membranes.12 This behavior enhances the NPs uptake as well as toxicity to cancerous cells as reported earlier.44 Therefore, TQ-PVP-Fe3O4 NPs can cause high cytotoxicity to MDA-MB-231 cells in a dose-dependent manner. In addition, the fluorescence microscopic analysis of RITC-TQ-PVP-Fe3O4 NPs clearly indicated the time-dependent cellular internalization (0 h, 1 h, 2 h, 4 h and 8 h) and the level of uptake of NPs by cancer cells (Fig. 7b). The graph shows the mean fluorescence intensity of the internalized NPs by cancer cells (Fig. 7c). These data provide the evidence of TQ-PVP-Fe3O4 in tumor cell delivery due to effective cell internalization of NPs for promoting the event of apoptosis. Further, the results of this study clearly showed an increase in cytotoxicity of thymoquinone, and it was due to the sustained release of the loaded drug molecules from PVPylated Fe3O4 NPs and/or their more effective uptake by cells. The observations confirm the cytotoxic behavior of NPs in their wide range of concentration and the doses closes to IC50 values exhibit enhanced therapeutic abilities of TQ-PVP-Fe3O4 NPs.
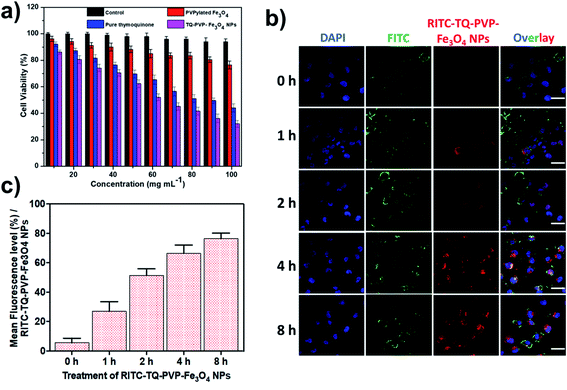 |
| Fig. 7 (a) Cytotoxicity assessment results of control, PVPylated Fe3O4, pure thymoquinone and TQ-PVP-Fe3O4 NPs in MDA-MB-231 cells at different concentrations and the expressed values are mean ± SD (n = 3). (b) Fluorescence microscopic analysis of IC50 concentrations of RITC-TQ-PVP-Fe3O4 NPs in cellular internalization at different time intervals of incubation. DAPI (nucleus) and FITC (cell membrane) stains were used to cell organelles. Scale bars 50 μm. (c) Graph showing the quantified level of internalized NPs with cancer cells at different time points; the values were calculated depending on the mean fluorescence levels from RITC-TQ-PVP-Fe3O4 NPs. | |
3.8. Analysis of mitochondrial membrane potentials (ΔΨm) and DNA damage
The changes in mitochondrial membrane potential (ΔΨm) can be visualized by rhodamine-123 (Rh 123) staining. Rh 123 is a cationic fluorescent dye and can be retained by functional mitochondria with high ΔΨm. TQ-PVP-Fe3O4 NP-treated cells were stained with Rh-123 and the loss of ΔΨm in cells was observed based on changes in their potentials after treating the cells with its IC50 for a period of 0, 1, 2, 4, and 8 h (Fig. 8). DAPI and Rh-123 were able to form fluorescent complexes with nuclear material and active mitochondrial membranes of the cells, respectively. Hence, these dyes were useful in detecting the apoptotic condensed nuclei and ΔΨm loss. The study observations showed that TQ-PVP-Fe3O4 NP-treated cells revealed the diminished size of apoptotic nuclei (green + blue color) and the assembly of condensed chromatin at the periphery of the nuclear membrane. The fragmented nuclear bodies and mitochondrial membranes were also observed in these cells. Besides, mitochondrial localization staining (Rh 123) of MDA-MB-231 cells treated with TQ-PVP-Fe3O4 NPs was observed in green color. The accumulation of fluorescence in MDA-MB-231 cells was also clearly visualized by fluorescence microscopy. These studies showed that TQ-PVP-Fe3O4 NPs induce mitochondrial membrane potential loss against MDA-MB-231 cancer cells. The quantitative analysis was performed to analyze the level of mitochondrial membrane potential loss as compared to lower time points (Fig. 8b). It was observed that there is an increased amount of Rh-123 uptake when the cells are exposed to prolonged duration (1 h, 2 h, 4 h and 8 h). It may happen due to the interaction of thymoquinone and surface receptors on the triple-negative breast cancer cells via surface functional groups45,46 of NPs, which adversely affects the cellular function and phenotype and induces cell death and cancerous tissue apoptosis.
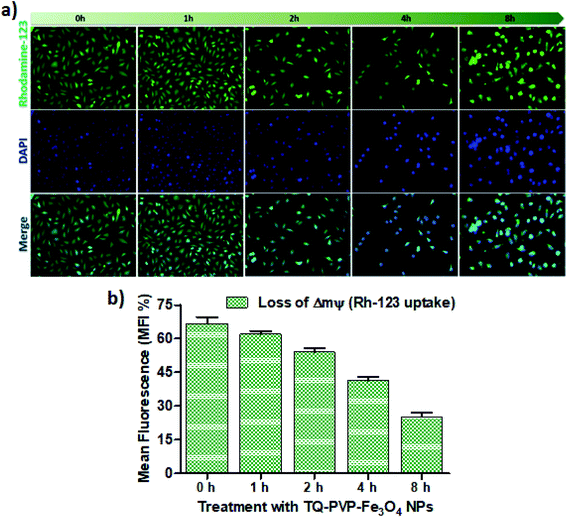 |
| Fig. 8 (a) Fluorescence microscopic images (20× magnification) of DAPI and Rh-123-stained MDA-MB-231 cells treated with TQ-PVP-Fe3O4 NPs for different time periods. The panel of images shows the induction of apoptosis and loss of ΔΨm in MDA-MB-231 cells; DAPI staining (blue color) shows the condensed and fragmented nuclear materials in TQ-PVP-Fe3O4 NP-treated cells as compared with untreated (control) cells; Rh-123 staining (green) indicates the loss of ΔΨm. The active mitochondrial membranes uptake Rh-123 and emit fluorescent green color and (b) quantitative analysis of loss of mitochondrial membrane potential based on the Rh-123 fluorescence level from MDA-MB-231 cells treated with TQ-PVP-Fe3O4 NPs. | |
3.9. Reactive oxygen species generation and nuclear fragmentation
Further, we examined the release of reactive oxygen species (ROS) from TQ-PVP-Fe3O4 NP-treated MDA-MB-231 cells to confirm the activation of the apoptosis signaling cascade. These were assessed by fluorescent DCFH-DA staining from apoptosis-induced cells. It was noticed that there was an increased amount of DCF signals through esterase activity, which might be due to the release of peroxidase cytochrome c in the cytoplasm. This resulted in higher ROS production in TQ-PVP-Fe3O4 NP-treated MDA-MB-231 cells than untreated (control) cells. Similarly, the production of ROS was increased in TQ-PVP-Fe3O4 NP-treated MDA-MB-231 cells in a dose-dependent manner, as displayed in Fig. 9a–e. This ROS fluorescence level indicates the amount of ROS generation from cancer cells due to treatment with NPs at different concentrations (Fig. 9f). The increase in ROS production in a dose-dependent manner was observed by the increased fluorescent intensity of treated cells. These findings suggested that the increased release rate of ROS generation can cause ΔΨm loss, which leads to the activation of intrinsic apoptosis cascade. It was also reported that the interaction of TQ-PVP-Fe3O4 NPs with MDA-MB-231 cells could induce oxidative stress through ROS production over the antioxidant defense strategies of cells.44,46,47 Thus, these increased ROS levels in cancer cells resulted in a change of mitochondrial membrane integrity and oxidative stress-associated DNA damage that could consequently lead to apoptosis.12 These effects of TQ-PVP-Fe3O4 NPs on cellular ROS production were investigated under a fluorescent microscope for their potentials to show green fluorescence from DCF through the intracellular oxidation of DCFH-DA (fluorescent dye). In addition, it is shown that TQ-PVP-Fe3O4 NPs of this study have the potential to increase intracellular ROS levels as well as to activate intrinsic apoptosis.44,46 Thus, the present study implies that the ROS generation and the associated oxidative stress induced by TQ-PVP-Fe3O4 NPs could effectively result in the activation of an intrinsic apoptotic pathway as revealed by extensive DNA damage of treated cancer cells and cell death (Scheme 1 and Fig. 10).
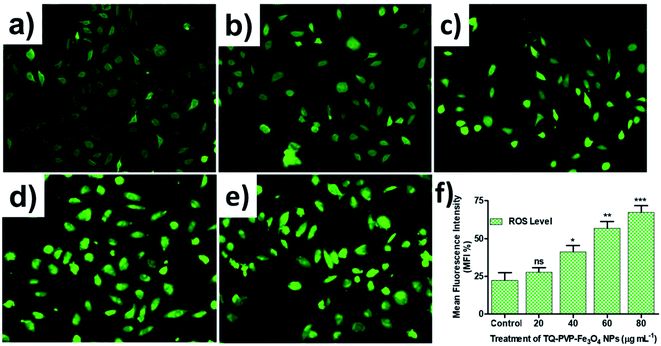 |
| Fig. 9 Fluorescent microscopic images (40× magnification) of intra-cellular ROS production after TQ-PVP-Fe3O4 NP treatment under the conditions of (a) control, (b) at concentrations of 20, (c) 40, (d) 60, and (e) 80 μg mL−1 for 24 h, and (f) quantitative analysis of released ROS from MDA-MB-231 cells upon treatment with different concentrations of TQ-PVP-Fe3O4 NPs; the level of fluorescence is compared to the MFI of the respective control (untreated) cells after 24 h of treatment. ns = Non-significant; *p < 0.05; **p < 0.01; ***p < 0.001. | |
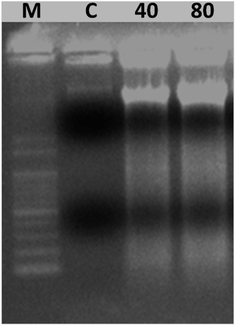 |
| Fig. 10 DNA fragmentation analysis of control and TQ-PVP-Fe3O4 NP-treated MDA-MB-231 cells. The fragmented DNA samples are loaded as M-marker DNA, C-control, and TQ-PVP-Fe3O4 NPs at concentrations of 40 μg mL−1 and 80 μg mL−1. | |
From the outcomes of this study, the enhanced mechanism of cellular uptake and growth inhibitory effects in MDA-MB-231 cells by TQ-PVP-Fe3O4 NPs was clearly demonstrated (as shown in Scheme 1). It was also assumed that TQ-PVP-Fe3O4 NPs effectively enter into the cellular system through the endocytosis process. The porous shape and positive zeta potential values play an important role in negatively charged tumor uptake and intracellular distribution. Then, TQ-PVP-Fe3O4 NPs might directly interact with the change in mitochondrial membrane potentials and agglomerate around the nuclear membrane of the nucleus.45 In this biological environment, the thymoquinone drug may be released from PVPylated Fe3O4 NPs via disruption of weak van der Waals force.12,26 Upon release, thymoquinone could accelerate apoptosis progression through DNA damage or membrane blebbing, resulting in cellular decomposition. In addition, TQ-PVP-Fe3O4 NPs possess high water solubility, good stability and increased anti-proliferation activity on tumor cells not only to enhance the drug index of the formulation but also to reduce the unwanted side effects of targeted drug delivery applications.
4. Conclusion
In summary, monodisperse porous PVPylated Fe3O4 NPs have been successfully synthesized by a solvothermal method. Thymoquinone was chosen as a model anticancer drug, which was loaded within porous PVPylated Fe3O4 NPs by the nanoprecipitation method. The FESEM morphological analysis confirmed the surface modification of magnetic NPs, preventing the agglomeration as well as forming porous nanospheres with a size range of ∼100 nm with superparamagnetic properties. The presence of PVPylated polymers could enhance water solubility, drug release and stability during the administration of TQ-PVP-Fe3O4 NPs. Moreover, it could deliver pre-determined encapsulated drugs more rapidly in an acidic pH environment as compared to a basic pH environment, showing the efficiency for rapid tumoricidal action. Anticancer studies of these Fe3O4 NPs demonstrated less toxic effects on MDA-MB-231 cells, whereas TQ-PVP-Fe3O4 NPs showed higher cytotoxicity. In addition, the particle size also played a vital role for targeting and releasing drug molecules in MDA-MB-231 cancer cells. It was also observed that TQ-PVP-Fe3O4 NP accumulation in tumor cells showed significant anticancer effects due to cellular decomposition and apoptosis. Hence, the developed method showed an effective manner of delivering water-insoluble therapeutic compounds to target cancer cells and enhance biological applications.
Conflicts of interest
There are no conflicts to declare.
Acknowledgements
The authors would like to acknowledge the DST-PURSE, DST-FIST and UGC-SAP, Government of India for the instrumental facilities to the department.
References
- A. Diana, E. Franzese, S. Centonze, F. Carlino, C. M. Della Corte, J. Ventriglia, A. Petrillo, F. De Vita, R. Alfano and F. Ciardiello, Triple-negative breast cancers: systematic review of the literature on molecular and clinical features with a focus on treatment with innovative drugs, Curr. Oncol. Rep., 2018, 20(10), 76 CrossRef PubMed.
- N. Olov, S. Bagheri-Khoulenjani and H. Mirzadeh, Combinational drug delivery using nanocarriers for breast cancer treatments: a review, J. Biomed. Mater. Res., Part A, 2018, 106(8), 2272–2283 CrossRef CAS PubMed.
- Z. Xu, X. Chen, Z. Sun, C. Li and B. Jiang, Recent progress on mitochondrial targeted cancer therapy based on inorganic nanomaterials, Mater. Today Chem., 2019, 12, 240–260 CrossRef CAS.
- B. D. White, C. Duan and H. E. Townley, Nanoparticle Activation Methods in Cancer Treatment, Biomolecules, 2019, 9(5), 202 CrossRef CAS PubMed.
- S. R. Kumar, M. Paulpandi, M. ManivelRaja, D. Mangalaraj, C. Viswanathan, S. Kannan and N. Ponpandian, An in vitro analysis of H1N1 viral inhibition using polymer coated superparamagnetic Fe3O4 nanoparticles, RSC Adv., 2014, 4(26), 13409–13418 RSC.
- T. Vangijzegem, D. Stanicki and S. Laurent, Magnetic iron oxide nanoparticles for drug delivery: applications and characteristics, Expert Opin. Drug Delivery, 2019, 16(1), 69–78 CrossRef CAS PubMed.
- M. Bilal, Y. Zhao, T. Rasheed and H. M. Iqbal, Magnetic nanoparticles as versatile carriers for enzymes immobilization: a review, Int. J. Biol. Macromol., 2018, 120, 2530–2544 CrossRef CAS PubMed.
- R. Vivek, R. Thangam, S. R. Kumar, C. Rejeeth, S. Sivasubramanian, S. Vincent, D. Gopi and S. Kannan, HER2 targeted breast cancer therapy with switchable “Off/On” multifunctional “Smart” magnetic polymer core–shell nanocomposites, ACS Appl. Mater. Interfaces, 2016, 8(3), 2262–2279 CrossRef CAS PubMed.
- O. S. Fenton, K. N. Olafson, P. S. Pillai, M. J. Mitchell and R. Langer, Advances in biomaterials for drug delivery, Adv. Mater., 2018, 30(29), 1705328 CrossRef PubMed.
- D. Bobo, K. J. Robinson, J. Islam, K. J. Thurecht and S. R. Corrie, Nanoparticle-based medicines: a review of FDA-approved materials and clinical trials to date, Pharm. Res., 2016, 33(10), 2373–2387 CrossRef CAS PubMed.
- S. K. Yen, P. Padmanabhan and S. T. Selvan, Multifunctional iron oxide nanoparticles for diagnostics, therapy and macromolecule delivery, Theranostics, 2013, 3(12), 986 CrossRef CAS PubMed.
- S. Bhattacharya, M. Ahir, P. Patra, S. Mukherjee, S. Ghosh, M. Mazumdar, S. Chattopadhyay, T. Das, D. Chattopadhyay and A. Adhikary, PEGylated-thymoquinone-nanoparticle mediated retardation of breast cancer cell migration by deregulation of cytoskeletal actin polymerization through miR-34a, Biomaterials, 2015, 51, 91–107 CrossRef CAS PubMed.
- M. A. Khan, M. Tania and J. Fu, Epigenetic role of thymoquinone: impact on cellular mechanism and cancer therapeutics, Drug Discovery Today, 2019, 24, 2315–2322 CrossRef CAS PubMed.
- M. Rafati, A. Ghasemi, M. Saeedi, E. Habibi, E. Salehifar, M. Mosazadeh and M. Maham,
Nigella sativa L. for Prevention of Acute Radiation Dermatitis in Breast Cancer: A Randomized, Double-blind, Placebo-controlled, Clinical Trial, Complement. Ther. Med., 2019, 102205 CrossRef PubMed.
- Y. K. Mahmoud and H. M. Abdelrazek, Cancer: thymoquinone antioxidant/pro-oxidant effect as potential anticancer remedy, Biomed. Pharmacother., 2019, 115, 108783 CrossRef CAS PubMed.
- M. Bule, S. Nikfar, M. Amini and M. Abdollahi, The Antidiabetic Effect of Thymoquinone: A Systematic Review and Meta-analysis of Animal Studies, Food Res. Int., 2020, 127, 108736 CrossRef CAS PubMed.
- M. Imran, A. Rauf, I. A. Khan, M. Shahbaz, T. B. Qaisrani, S. Fatmawati, T. Abu-Izneid, A. Imran, K. U. Rahman and T. A. Gondal, Thymoquinone: a novel strategy to combat cancer: a review, Biomed. Pharmacother., 2018, 106, 390–402 CrossRef CAS PubMed.
- O. R. Johnson-Ajinwo, A. Richardson and W.-W. Li, Synthesis and Evaluation of Thymoquinone Analogues as Anti-Ovarian Cancer Agents, Proceedings, 2019, 22(1), 42 CrossRef.
- R. Schneider-Stock, I. H. Fakhoury, A. M. Zaki, C. O. El-Baba and H. U. Gali-Muhtasib, Thymoquinone: fifty years of success in the battle against cancer models, Drug Discovery Today, 2014, 19(1), 18–30 CrossRef CAS PubMed.
- M. Yusufi, S. Banerjee, M. Mohammad, S. Khatal, K. V. Swamy, E. M. Khan, A. Aboukameel, F. H. Sarkar and S. Padhye, Synthesis, characterization and anti-tumor activity of novel thymoquinone analogs against pancreatic cancer, Bioorg. Med. Chem. Lett., 2013, 23(10), 3101–3104 CrossRef CAS PubMed.
- K. M. Sutton, C. D. Doucette and D. W. Hoskin, NADPH quinone oxidoreductase 1 mediates breast cancer cell resistance to thymoquinone-induced apoptosis, Biochem. Biophys. Res. Commun., 2012, 426(3), 421–426 CrossRef CAS PubMed.
- B. R. Acharya, A. Chatterjee, A. Ganguli, S. Bhattacharya and G. Chakrabarti, Thymoquinone inhibits microtubule polymerization by tubulin binding and causes mitotic arrest following apoptosis in A549 cells, Biochimie, 2014, 97, 78–91 CrossRef CAS PubMed.
- W. H. Suriyah, A. R. Kasmuri, F. H. N. Foong, D. Afriza and S. J. A. Ichwan, Comparison of the in vitro and in vivo toxic effects of thymoquinone using oral cancer HSC-3 and HSC-4 cell lines, oral fibroblasts, HACAT cell line, and Zebrafish embryos, Mater. Today: Proc., 2019, 16, 2108–2114 Search PubMed.
- S. A. Helmy, M. El-Mesery, A. El-Karef, L. A. Eissa and A. M. El Gayar, Thymoquinone upregulates TRAIL/TRAILR2 expression and attenuates hepatocellular carcinoma in vivo model, Life Sci., 2019, 233, 116673 CrossRef CAS PubMed.
- M. Ogden, S. B. Karaca, G. Aydin, U. Yuksel, A. T. Dagli, S. Akkaya and B. Bakar, The Healing Effects of Thymoquinone and Dexpanthenol in Sciatic Nerve Compression Injury in Rats, J. Invest. Surg., 2019, 1–9 CrossRef PubMed.
- S. R. Kumar, S. Priyatharshni, V. Babu, D. Mangalaraj, C. Viswanathan, S. Kannan and N. Ponpandian, Quercetin conjugated superparamagnetic magnetite nanoparticles for in vitro analysis of breast cancer cell lines for chemotherapy applications, J. Colloid Interface Sci., 2014, 436, 234–242 CrossRef PubMed.
- S. R. Kumar, V. Jayavignesh, R. Selvakumar, K. Swaminathan and N. Ponpandian, Facile synthesis of yeast cross-linked Fe3O4 nanoadsorbents for efficient removal of aquatic environment contaminated with As (V), J. Colloid Interface Sci., 2016, 484, 183–195 CrossRef PubMed.
- S. R. Kumar, M. M. Raja, D. Mangalaraj, C. Viswanathan and N. Ponpandian, Surfactant free solvothermal synthesis of monodispersed 3D hierarchical Fe3O4 microspheres, Mater. Lett., 2013, 110, 98–101 CrossRef CAS.
- G. G. Utkan, F. Sayar, P. Batat, S. Ide, M. Kriechbaum and E. Pişkin, Synthesis and characterization of nanomagnetite particles and their polymer coated forms, J. Colloid Interface Sci., 2011, 353(2), 372–379 CrossRef CAS PubMed.
- S. R. Kumar, L. Marianna, S. Gianni, A. J. Nathanael, S. Hong, T. H. Oh, D. Mangalaraj, C. Viswanathan and N. Ponpandian, Hydrophilic polymer coated monodispersed Fe3O4 nanostructures and their cytotoxicity, Mater. Res. Express, 2014, 1(1), 015015 CrossRef.
- M. S. Al-Qubaisi, A. Rasedee, M. H. Flaifel, E. E. M. Eid, S. Hussein-Al-Ali, F. H. Alhassan, A. M. Salih, M. Z. Hussein, Z. Zainal, D. Sani, A. H. Aljumaily and M. I. Saeed, Characterization of thymoquinone/hydroxypropyl-β-cyclodextrin inclusion complex: Application to anti-allergy properties, Eur. J. Pharm. Sci., 2019, 133, 167–182 CrossRef CAS PubMed.
- B. Kong, J. Tang, Z. Wu, J. Wei, H. Wu, Y. Wang, G. Zheng and D. Zhao, Ultralight mesoporous magnetic frameworks by interfacial assembly of prussian blue nanocubes, Angew. Chem., Int. Ed., 2014, 53(11), 2888–2892 CrossRef CAS PubMed.
- H. Qu, D. Caruntu, H. Liu and C. J. O'Connor, Water-dispersible iron oxide magnetic nanoparticles with versatile surface functionalities, Langmuir, 2011, 27(6), 2271–2278 CrossRef CAS PubMed.
- C. S. Lee, H. H. Chang, P. K. Bae, J. Jung and B. H. Chung, Bifunctional Nanoparticles Constructed Using One-Pot Encapsulation of a Fluorescent Polymer and Magnetic (Fe3O4) Nanoparticles in a Silica Shell, Macromol. Biosci., 2013, 13(3), 321–331 CrossRef CAS PubMed.
- K. V. Shafi, A. Gedanken, R. Prozorov and J. Balogh, Sonochemical preparation and size-dependent properties of nanostructured CoFe2O4 particles, Chem. Mater., 1998, 10(11), 3445–3450 CrossRef CAS.
- S. Ghosh, A. Badruddoza, M. Uddin and K. Hidajat, Adsorption of chiral aromatic amino acids onto carboxymethyl-β-cyclodextrin bonded Fe3O4/SiO2 core–shell nanoparticles, J. Colloid Interface Sci., 2011, 354(2), 483–492 CrossRef CAS PubMed.
- P. Papaphilippou, M. Christodoulou, O.-M. Marinica, A. Taculescu, L. Vekas, K. Chrissafis and T. Krasia-Christoforou, Multiresponsive polymer conetworks capable of responding to changes in pH, temperature, and magnetic field: synthesis, characterization, and evaluation of their ability for controlled uptake and release of solutes, ACS Appl. Mater. Interfaces, 2012, 4(4), 2139–2147 CrossRef CAS PubMed.
- S.-H. Wu, Y. Hung and C.-Y. Mou, Mesoporous silica nanoparticles as nanocarriers, Chem. Commun., 2011, 47(36), 9972–9985 RSC.
- S. Afzal, R. Khan, T. Zeb, M. ur Rahman, S. Ali, G. Khan, Z. ur Rahman and A. Hussain, Structural, optical, dielectric and magnetic properties of PVP coated magnetite (Fe3O4) nanoparticles, J. Mater. Sci.: Mater. Electron., 2018, 29(23), 20040–20050 CrossRef.
- J. Zheng, Z. Liu, X. Zhao, M. Liu, X. Liu and W. Chu, One-step solvothermal synthesis of Fe3O4@C core–shell nanoparticles with tunable sizes, Nanotechnology, 2012, 23(16), 165601 CrossRef CAS PubMed.
- K. Cheng, S. Peng, C. Xu and S. Sun, Porous hollow Fe3O4 nanoparticles for targeted delivery and controlled release of cisplatin, J. Am. Chem. Soc., 2009, 131(30), 10637–10644 CrossRef CAS PubMed.
- S. Bhattacharya, A. Ghosh, S. Maiti, M. Ahir, G. H. Debnath, P. Gupta, M. Bhattacharjee, S. Ghosh, S. Chattopadhyay and P. Mukherjee, Delivery of thymoquinone through hyaluronic acid-decorated mixed Pluronic® nanoparticles to attenuate angiogenesis and metastasis of triple-negative breast cancer, J. Controlled Release, 2020, 322, 357–374 CrossRef CAS PubMed.
- P. Upadhyay, S. Sarker, A. Ghosh, P. Gupta, S. Das, M. Ahir, S. Bhattacharya, S. Chattopadhyay, S. Ghosh and A. Adhikary, Transferrin-decorated thymoquinone-loaded PEG-PLGA nanoparticles exhibit anticarcinogenic effect in non-small cell lung carcinoma via the modulation of miR-34a and miR-16, Biomater. Sci., 2019, 7(10), 4325–4344 RSC.
- E.-S. A. Arafa, Q. Zhu, Z. I. Shah, G. Wani, B. M. Barakat, I. Racoma, M. A. El-Mahdy and A. A. Wani, Thymoquinone up-regulates PTEN expression and induces apoptosis in doxorubicin-resistant human breast cancer cells, Mutat. Res., Fundam. Mol. Mech. Mutagen., 2011, 706(1–2), 28–35 CrossRef CAS PubMed.
- M. Shah, N. Ullah, M. H. Choi, M. O. Kim and S. C. Yoon, Amorphous amphiphilic P (3HV-co-4HB)-b-mPEG block copolymer synthesized from bacterial copolyester via melt transesterification: nanoparticle preparation, cisplatin-loading for cancer therapy and in vitro evaluation, Eur. J. Pharm. Biopharm., 2012, 80(3), 518–527 CrossRef CAS PubMed.
- R. Thangam, S. Sundarraj, R. Vivek, V. Suresh, S. Sivasubramanian, M. Paulpandi, S. V. Karthick, A. S. Ragavi and S. Kannan, Theranostic potentials of multifunctional chitosan–silver–phycoerythrin nanocomposites against triple negative breast cancer cells, RSC Adv., 2015, 5(16), 12209–12223 RSC.
- A. H. El-Far, S. K. Al Jaouni, W. Li and S. A. Mousa, Protective roles of thymoquinone nanoformulations: Potential nanonutraceuticals in human diseases, Nutrients, 2018, 10(10), 1369 CrossRef PubMed.
Footnote |
† Electronic supplementary information (ESI) available. See DOI: 10.1039/d0na00242a |
|
This journal is © The Royal Society of Chemistry 2020 |
Click here to see how this site uses Cookies. View our privacy policy here.