DOI:
10.1039/D0NA00172D
(Paper)
Nanoscale Adv., 2020,
2, 4242-4250
Nickel sulfide-incorporated sulfur-doped graphitic carbon nitride nanohybrid interface for non-enzymatic electrochemical sensing of glucose†
Received
1st March 2020
, Accepted 30th July 2020
First published on 30th July 2020
Abstract
A nickel sulfide-incorporated sulfur-doped graphitic carbon nitride (NiS/S-g-C3N4) nanohybrid was utilized as an interface material for the non-enzymatic sensing of glucose in an alkaline medium (0.1 M NaOH). The precursors used in the preparation of NiS/S-g-C3N4 hybrid were thiourea and nickel nitrate hexahydrate as the sulfur and nickel sources, respectively. The HRTEM results reveal that NiS nanoparticles incorporated on the S-g-C3N4 nanosheet surface could enhance the electrocatalytic activity and electrical conductivity. The prepared NiS/S-g-C3N4 crystalline nature, surface functionalities, graphitic nature, thermal stability and surface composition were investigated using XRD, FT-IR, Raman spectroscopy, TGA and XPS analyses. The NiS/S-g-C3N4 modified electrode was used for the non-enzymatic sensing of glucose at an applied potential of 0.55 V vs. Ag/AgCl with a detection limit of 1.5 μM (S/N = 3), sensitivity of 80 μA mM−1 cm−2 and the response time of the fabricated sensor was close to 5 s. Different inorganic ions and organic substances did not interfere during glucose sensing. The NiS/S-g-C3N4 nanohybrid material could be extended for a real sample analysis and open the way for diverse opportunities in the electrochemical sensing of glucose.
1. Introduction
Glucose sensing has profound importance in the field of clinical diagnostics, biotechnology, environmental monitoring, pharmaceutical analysis and food production.1,2 The advanced testing and analyses of glucose levels in blood and urine samples are key markers to identify diabetics that has an increased rate of mortality next to cancer. Until now, the determination of glucose has been carried out by several methods, including fluorescence3 and optical spectroscopy,4 acoustic techniques,5 surface plasmon resonance spectroscopy,6 electrochemiluminescence,7 and electrochemical techniques.1,8 The advantage of electrochemical methods over other techniques is that they are compact, relatively inexpensive, reliable, sensitive and can achieve real-time analysis. In general, glucose detection mostly involves enzymatic reactions,9 but the use of enzyme (glucose oxidase) based biosensor are limited due to the instability of the enzyme activity, expensive fabrication cost, change of temperature, pH, and half shelf life. Even though commercial sensing devices are inferior to enzymatic systems, the last decade has seen growth in the research interest in non-enzymatic glucose sensing with different nanomaterials. The advances in nanotechnology have enabled new possibilities for making modernistic glucose sensors with non-enzymatic methods. The poor selectivity and surface fouling problem could be solved with electrocatalyst nanostructure-based non-enzymatic sensors and exhibit higher sensitivity compared with enzymatic systems.10
Glucose enzymatic biosensors require different modification strategies such as the electropolymerization of enzyme,11 cross-linking of enzyme,12 enzyme entrapping by sol–gel methods,13 wiring enzyme electrodes of glucose oxidase to electrochemically mediated polymer chains,14etc., to achieve stability. These efforts confirmed the stability of enzymatic glucose sensors for short-term period and facilitate one-time usage only. The enzymatic glucose sensor based on glucose oxidase is exposed to thermal and chemical conditions during modification, storage and usage. To overcome these limitations, non-enzymatic sensors based on the glucose oxidation reaction and catalysed by various electrocatalysts such as noble metal nanoparticles,15 transition metal oxides,16–18 carbon nanotubes,19 gold nanocages,20 polymer composites,11 alloys,21 complexes,22–24 Ni3S2/NiMoO4 nanowires,25 Ag–/Au–NiCo2O4 nanosheets,26 NiO hollow cages,27 porous NiCo2O4 nanoarrays,28 and Ni/Al layered double hydroxides,29 have been employed.
Niu et al. investigated non-enzymatic glucose sensing with three-dimensional porous nickel nanostructures with a low detection limit of 0.07 μM.30 Huo et al. reported non-enzymatic glucose detection using 3D-Ni3S2 nanosheet arrays supported on Ni foam with high electrocatalytic activity towards glucose detection, 1.2 μM, with a high sensitivity of 6148.0 μA mM−1 cm−2.31 The polymeric semiconductor, graphitic carbon nitride (g-C3N4), has recently acquired research interest in the area of electrochemical sensors due to its extensive unique properties. To increase the electrocatalytic activity and electrical conductivity, sulfur-based g-C3N4 has been tuned for quick electron transfer.22 Further, Tian et al. analyzed ultrathin graphitic carbon nitride nanosheets utilized as highly efficient electrocatalyst materials for glucose biosensing with a limit of detection and linear range of 11 μM and 1–12 mM, respectively.32 Kim et al. reported nickel sulfide nanostructures for non-enzymatic glucose sensing with a limit of detection of 0.82 μM.33 Also, to avoid the inactivation of analyte in the reaction solutions, various metal-based glucose sensors have been exploited. Along with these metals chalcogenides, the redox chemistry of transition metal chalcogenides (TMCs) has demonstrated higher activity of glucose oxidation due to its layer-dependent physical and chemical properties, which can enhance the redox activity.34 Among various TMCs, nickel sulfide (NiS) has been widely used as an electrode interface material for the non-enzymatic detection of glucose due to its high conductivity, but its use in electrochemical sensors for any analyte in combination with S-g-C3N4 has not been explored to date. Kim et al. reported morphology-controlled Ni3S2 utilized for non-enzymatic glucose sensors with a 0.82 μM limit of detection.33 The g-C3N4 electrochemical sensing activity was limited due to the surface fouling, chemical inertness and poor conductivity. Hence, the electrochemical performance of g-C3N4 could be enhanced by hybridization with other nanomaterials applied in the fabrication of electrochemical sensors.35,36 Functionalized g-C3N4 and its hybrid/composite materials have been employed for the electrochemical detection of glucose, nitrobenzene, H2O2, NADH, and mercuric ions.37–39 Moreover, Sun and co-workers investigated the g-C3N4 nanosheets utilized in electrochemical glucose bio-sensing with an 11 μM LoD in buffer solution.38 In addition, g-C3N4 nanosheets have been exploited with the enzymatic oxidation of glucose using glucose oxidase.40 Kannan et al. investigated the glucose oxidation through fabrication of NiS thin films used for a non-enzymatic glucose sensor with a 0.32 μM LoD and response time of <8 s.41 Hence, in this work, the NiS/S-g-C3N4 nanohybrid material was fabricated as the interface matrix and explored as an electrode material for glucose oxidation (Scheme 1) and the electrochemical parameters were investigated.
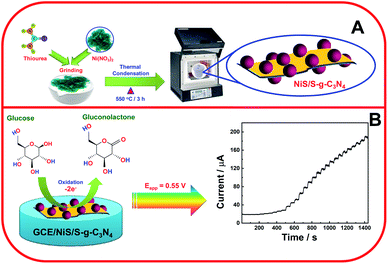 |
| Scheme 1 (A) Schematics for the synthesis of the NiS/S-g-C3N4 nanohybrid material and (B) schematics for the electrocatalytic oxidation of glucose at the NiS/S-g-C3N4 modified electrode and its electrochemical response. | |
2. Experimental methods
2.1. Chemicals and reagents
Chemicals such as thiourea (CH4N2S) and nickel nitrate hexahydrate (Ni(NO3)2·6H2O) were purchased from SRL laboratories and used as received. All other reagents were of analytical grade and used without further purification. Double distilled water was utilized throughout the experiments, which was collected from a MILLIPORE water system.
2.2. Synthesis of NiS-incorporated S-g-C3N4 nanosheets
The NiS-incorporated S-g-C3N4 nanosheets were synthesized by the following procedure (Scheme 1A). First, 3 g of thiourea with 600 mg of nickel nitrate hexahydrate were mixed in an agate mortar and pestle for an hour. The resulting mixture was transferred to an alumina crucible for calcination at 550 °C for 3 h. It was then allowed to cool down to room temperature in order to obtain NiS-engulfed S-doped g-C3N4. The bare S-g-C3N4 was prepared by the same procedure without the addition of nickel nitrate hexahydrate. The prepared samples were denoted as NiS/S-g-C3N4 and S-g-C3N4, respectively.
2.3. Fabrication of the NiS/S-g-C3N4/GCE nanohybrid
The modified glassy carbon electrode (GCE) was fabricated by dispersing 1 mg of the NiS/S-g-C3N4 in 1 mL distilled water and 5 μL of the solution was drop-casted onto a glassy carbon electrode (GCE) and allowed to dry at room temperature. The modified GCE was used as a working electrode and it was designated as NiS/S-g-C3N4/GCE.
3. Results and discussion
3.1. Morphological studies of S-g-C3N4–NiS nanohybrid materials
The HRTEM images of NiS/S-g-C3N4 are shown in Fig. 1(A and B). The NiS nanoparticles were randomly incorporated into S-g-C3N4 nanosheet layers. HRTEM results obviously reveal the interfacial contacts between NiS and S-g-C3N4, which promote the charge transfer and increase the active sites of the catalyst, thus improving the electrocatalytic activity and electrical conductivity during glucose sensing. In addition, High Angle Annular Dark Field – Scanning Transmission Electron Microscopy (HAADF-STEM) images confirm the presence of individual elements such as Ni, S, C and N in the NiS/S-g-C3N4 nanohybrid materials (Fig. 2(A–F)).
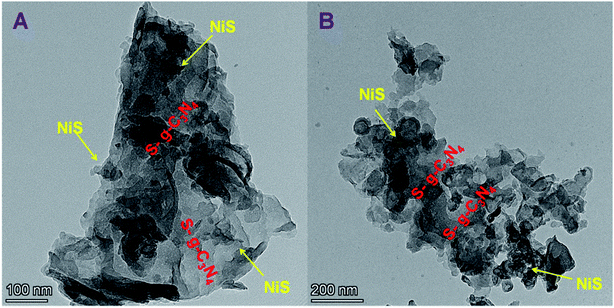 |
| Fig. 1 (A and B) HRTEM images obtained for the NiS/S-g-C3N4 nanohybrid material. | |
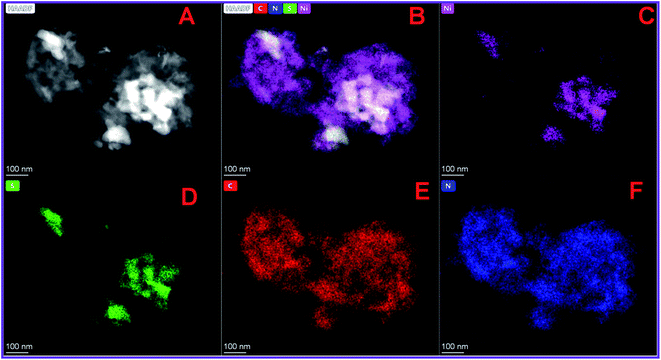 |
| Fig. 2 (A and B) HAADF-STEM image and elemental mapping of (C) Ni, (D) S, (E) C and (F) N in NiS/S-gC3N4 nanohybrid materials. | |
3.2. FT-IR and Raman spectral studies of NiS/S-g-C3N4 nanohybrid material
The surface functionalities and chemical bonding in the prepared samples were investigated by FT-IR spectroscopy, as shown in Fig. 3(A). The integration of NiS in S-g-C3N4 did not significantly alter the FT-IR spectrum of S-g-C3N4. However, the reduction in the spectrum intensity is said to be due to the integration of NiS in the NiS/S-g-C3N4 nanohybrid material. In both materials, the FT-IR signal observed in 1200 to 1650 cm−1 could be attributed to the stretching vibrations of heptazine heterocyclic (C6N7) units of S-g-C3N4.42 The peak at 810 cm−1 is due to the breathing vibration of triazine units, which is due to the condensed CN heterocycles.43 The bands from 2900 to 3500 cm−1 were attributed to the adsorbed water molecules and N–H vibration of the uncondensed amine groups. The presence of these bonds was found in the NiS/S-g-C3N4 nanohybrid material with low intensity due to the integration of NiS, which induced the change in the chemical bonding. However, NiS/S-g-C3N4 exhibits a peak at 617 cm−1, due to the Ni–S stretching vibration mode of NiS.44 The FT-IR spectra of bulk g-C3N4, g-C3N4 nanosheets, S-g-C3N4, and NiS/S-g-C3N4 are shown in Fig. S1.† There is no significant change in the triazine and heptazine units in the g-C3N4 polymeric network. However, the intensity variation represents the nature of the chemical bonding in the different g-C3N4 structures. The corresponding Raman spectra exhibited in Fig. S2† show the G band and D band; thus, the graphitic nature of the carbon nitride family could be confirmed in the bulk g-C3N4, g-C3N4 nanosheets, S-g-C3N4, and NiS/S-g-C3N4 samples.
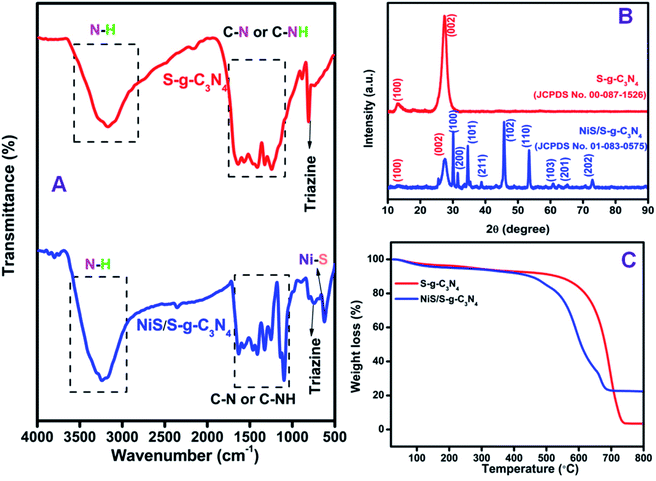 |
| Fig. 3 (A) FT-IR spectra, (B) XRD patterns and (C) TGA curves obtained for S-g-C3N4 and the NiS/S-g-C3N4 nanohybrid material. | |
3.3. X-ray diffraction analysis of NiS/S-g-C3N4 nanohybrid materials
The X-ray diffraction (XRD) pattern of the S-g-C3N4 and NiS/S-g-C3N4 nanohybrid material was studied to confirm the crystalline phase and the obtained results are shown in Fig. 3(B). The XRD pattern of S-g-C3N4 shows two main peaks at 2θ values of 13.3° and 27.5°, corresponding to the (100) and (002) planes, respectively;45 thus, the patterns are in good agreement with JCPDS card no: 00-087-1526. The peak at 27.5° appears due to the interlayer stacking of conjugated aromatic systems and the peak at 13.3° is due to the in-plane structural packing of aromatic systems.46 The XRD pattern of the NiS/S-g-C3N4 nanohybrid material shows peaks at 13.3° and 27.5°, corresponding to the (100) and (002) planes, respectively, due to the presence of S-g-C3N4 in the hybrid material. The other peaks at 30.2°, 31.7°, 34.7°, 39.3°, 45.9°, 53.6°, 60.5°, 65.1°, and 73.0° correspond to the (100), (200), (101), (211), (102), (110), (103), (201), and (202) planes due to the existence of NiS in the NiS/S-g-C3N4 nanohybrid material, respectively, and these peaks match well with JCPDS card no: 01-083-0575.
3.4. Thermo-gravimetric analysis of NiS/S-g-C3N4 nanohybrid material
The thermal stability and the composition of the prepared sample was studied using the thermo-gravimetric analysis (TGA) curves obtained at a heating rate of 10 °C min−1 under air atmosphere from room temperature to 800 °C. As shown in Fig. 3(C), the NiS/S-g-C3N4 nanohybrid material decomposes at lower temperatures compared with pure S-g-C3N4. When the increase in temperature is over 700 °C, the S-g-C3N4 undergoes complete decomposition. The combustion temperature of the nanohybrid material is reduced due to the presence of embedded NiS particles in the S-g-C3N4.
3.5. X-ray photoelectron spectroscopy studies of the NiS/S-g-C3N4 nanohybrid material
The surface composition and oxidation states of the elements in NiS/S-g-C3N4 were confirmed by X-ray photoelectron spectroscopic (XPS) analysis and the results shown in Fig. 4. The survey scan for the NiS/S-g-C3N4 nanohybrid material reveals the presence of C, N, Ni and S (Fig. 4(A)). The C 1s core-level spectrum (Fig. 4(B)) was deconvoluted into three peaks at 285.7, 284.8 and 282.2 eV, which correspond to N
C–N, in which sp2 bonded, C–O species on the g-C3N4 surface, and C–C are present in the g-C3N4 surface, respectively.47–49 The deconvoluted peaks for the N 1s core-level spectrum (Fig. 4(C)) are due to the presence of triazine rings C
N–C (403.2 eV), tertiary nitrogen N–(C)3 (405.3 eV) and amino functional groups (C–N–H) (407.3 eV), respectively.50Fig. 4(D) shows peaks at 860.5 and 862.4 eV in the Ni 2p spectrum, which are associated with Ni 2p3/2 and its satellite peak, and the peaks at 880.2 and 867.7 eV correspond to Ni 2p1/2 and its satellite peak51 in NiS/S-g-C3N4. The S 2p spectrum in Fig. 4(E) shows four main peaks at 160.0, 161.5, 163.1 and 165.4 eV. The peaks at 161.5 and 163.1 eV are attributed to the 2p3/2 and 2p1/2 of thiophene-S (–C–S–C–) due to spin–orbit coupling, while the peaks at 160.0 eV and 165.4 eV associated with S 2p1/2 and S 2p3/2, respectively, are consistent with the S 2p of NiS in NiS/S-g-C3N4.52 The XPS survey scan for S-g-C3N4 and its corresponding C 1s, N 1s and S 2p core-level spectra are shown in Fig. S3† for comparison.
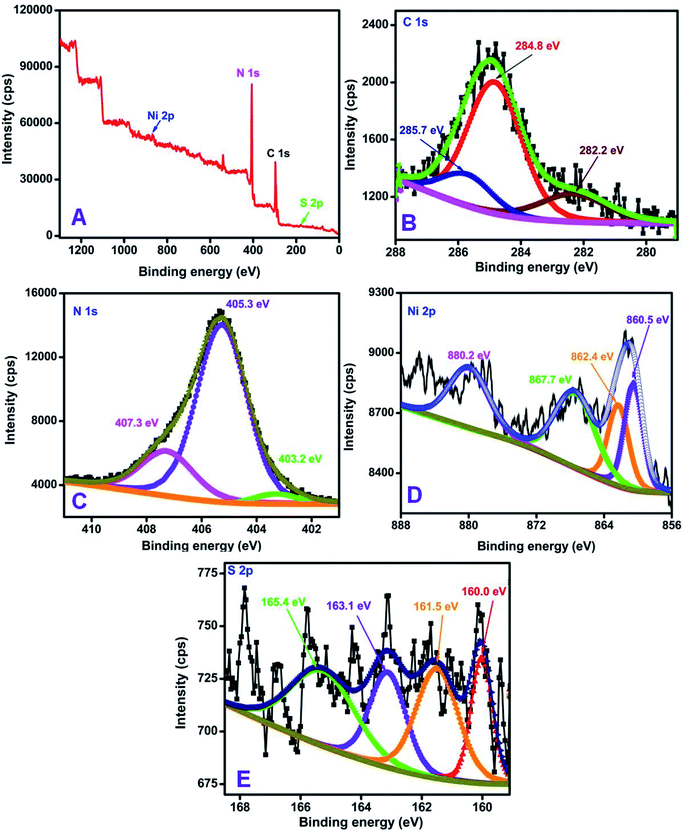 |
| Fig. 4 XPS spectra of the NiS/S-g-C3N4 nanohybrid material (A) survey scan, (B) C 1s, (C) N 1s, (D) Ni 2p, and (E) S 2p core-level spectra. | |
3.6. Electrochemical studies of the NiS/S-g-C3N4/GCE modified electrode
Prior to the electrochemical measurements, the electrode-dependent properties of the modified electrode were compared with the benchmark ferricyanide (Fe(CN)63−/4−) system in the potential window of −0.2 V to +0.8 V vs. Ag/AgCl (Fig. 5). The redox peak response of bare GCE at −0.2 V vs. Ag/AgCl corresponds to the oxidation/reduction process of the 1 mM Fe(CN)63−/4− in 0.1 M KCl solution. On modifying the bare electrode with S-g-C3N4, deviation in the peak current and a distorted peak potential were observed, which shows the limited electron transfer behavior of the modified S-g-C3N4. To increase and facilitate the electron transfer behavior of the modified electrode, the NiS-incorporated S-g-C3N4 nanohybrid material was chosen as an electrode modifier and the redox response in the ferricyanide solution shows a defined peak at +0.2 V as well as an additional peak corresponding to nickel sulfide at +0.65 V vs. Ag/AgCl with an ipa and peak to peak separation value of 285 μA cm−2 and 90 mV, respectively. The influence of electrolyte pH on glucose oxidation was studied for the NiS/S-g-C3N4 modified electrode using cyclic voltammetry. Starting from acidic pH 3 to neutral pH, the glucose response was negligible whereas, the modified system exhibited higher oxidation at higher pH 8–11, which showed the NiS interface oxidized the glucose at its maximum only in an alkaline medium. Hence, 0.1 M NaOH was used as the supporting electrolyte throughout the experiments. In addition, different loadings of material (0.5, 1.0, and 1.5 mg mL−1) were monitored for the NiS/S-g-C3N4 nanohybrid modified system. The glucose oxidation response was found to increase for the loading of NiS/S-g-C3N4 up to 1 mg mL−1 whereas, a decrease in the oxidation current was observed in the higher loading concentration of 1.5 mg mL−1 of g-C3N4 (Fig. S5†).
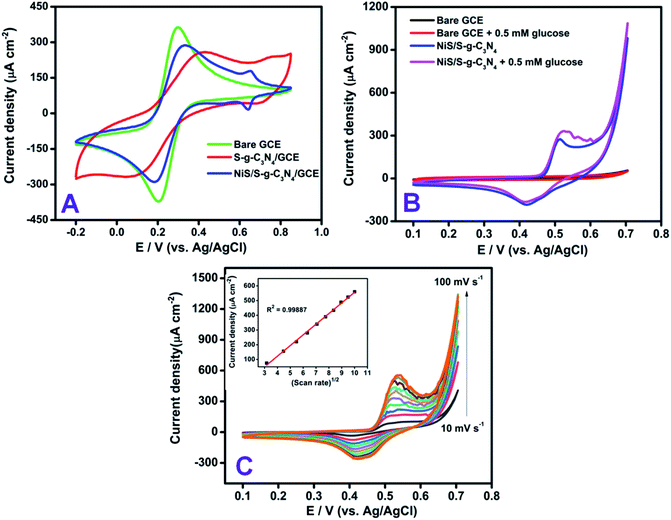 |
| Fig. 5 (A) Comparative CV responses of bare GCE, S-g-C3N4/GCE, and NiS/S-g-C3N4/GCE in 5 mM K3[Fe(CN)6] in 0.5 M KCl solution, (B) comparative CVs of step by step modification of bare GCE, bare GCE with 0.5 mM of glucose, NiS/S-g-C3N4 modified GCE and NiS/S-g-C3N4 modified GCE with 0.5 mM of glucose in 0.1 M NaOH solution at a scan rate of 50 mV s−1 and (C) CV responses of different scan rates (10–100 mV s−1) with 0.5 mM glucose at the NiS/S-g-C3N4 modified GCE in 0.1 M NaOH solution. Inset: plot of anodic (ipa) and cathodic peak current (ipc) vs. scan rate were obtained for 0.5 mM glucose at NiS/S-g-C3N4 modified GCE in 0.1 M NaOH solution. | |
In order to verify the electrocatalytic performance of the NiS/S-g-C3N4 nanohybrid material, CV measurements were recorded in 0.1 M NaOH solution containing 0.5 mM glucose, as shown in Fig. 5(B). The unmodified GCE did not show any appreciable electrocatalytic response. The significant peak current due to glucose oxidation was observed upon bare GC/NiS modification (Fig. S6†) whereas, the NiS/S-g-C3N4 modified GCE showed a greater anodic peak current response after the addition of 0.5 mM glucose in the NaOH solution.
This clearly indicates that the NiS/S-g-C3N4 modified electrode has good electrocatalytic performance towards the electro-oxidation of glucose in alkaline medium. Kim et al. reported nickel sulfide as an electroctalyst material for non-enzymatic glucose sensing.33 Sonkar et al. investigated non-enzymatic electrochemical sensing based on nickel complex-immobilized multiwall carbon nanotubes for glucose determination.53 The effect of scan rate was studied for NiS/S-g-C3N4/GCE by varying the scan rates ranging from 10 to 100 mV s−1 in Fe(CN)63−/4− dissolved in 0.1 M KCl as a supporting electrolyte. The oxidation and reduction peak currents of the modified electrode linearly increased by varying different applied scan rates on the electrode surface. The dual peak at +0.2 V and +0.65 V vs. Ag/AgCl were found to linearly trend with the respective scan rates, as shown in Fig. S4,† indicating that the Fe(CN)63−/4− redox couple is a diffusion-controlled process at NiS/S-g-C3N4/GCE. The regression coefficient was calculated to be R2 = 0.9998. The dependence of the peak current (Ip) and potential (Ep) with the scan rate (v) were then taken into account to analyze the glucose oxidation in 0.1 M NaOH as a supporting electrolyte. The addition of 0.5 mM glucose with different scan rates ranging from 10–100 mV s−1 was plotted in the inset of Fig. 5(C), which exhibits the diffusion-controlled electron transfer nature of the electrode. The obtained slope value, being greater than the theoretically expected value of 0.5 for a reversible process, suggests that the electrocatalytic oxidation of glucose can achieve the maximum. To show the steep increase in the concentration of glucose, linear sweep voltammetry was used. The linear increase in the oxidation current was obtained by each addition of increasing concentration glucose from 0 to 1 mM Fig. 6(A). From the slope value, the sensitivity was found to be 118 μA mM−1 cm−2 and the R2 value was 0.9993, which confirms the linear response of the modification.
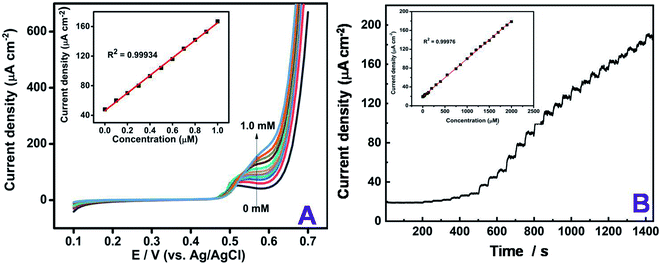 |
| Fig. 6 (A) LSV responses of NiS/S-g-C3N4/GCE in 0.1 M NaOH solution at a scan rate of 10 mV s−1 with different concentrations of glucose (0 to 1 mM); inset: calibration plot between current density vs. glucose concentration and (B) amperometric J–T response of NiS/S-g-C3N4 modified GCE upon spiking with sequential additions of 100 μM glucose in 0.1 M NaOH at 0.55 V vs. Ag/AgCl. | |
Based on the above findings from cyclic voltammetry experiments, the typical amperometric responses of the NiS/S-g-C3N4 modified GCE with the successive addition of glucose in the concentration ranges from 1 to 2100 μM were investigated and the results obtained are plotted in Fig. 6(B). A stepwise oxidation in the peak current with respect to increasing concentrations of glucose was observed, which in turn acts as a sensitive non-enzymatic glucose sensor with good linear range up to the concentration of 2.1 mM. The limit of quantification (LOQ) was calculated to be 1 μM. The NiS/S-g-C3N4 also exhibited a high sensitivity of 80 μA mM−1 cm−2 and the response time of the fabricated sensor was the average of the stepwise increment from the amperometric I–t curve during the glucose addition and the calibration plot between the steady state current density vs. glucose concentration, which was close to 5 s. The developed sensor showed an excellent detection of glucose even at low concentrations with a limit of detection (LOD) of 1.5 μM (S/N = 3). From the amperometric I–t curve, the diffusion coefficient was calculated by the following Cottrell equation,54
where
D represents the diffusion coefficient (cm
2 s
−1),
n,
c,
F,
A and
i are the number of electrons transferred, the bulk concentration of glucose (mol cm
−3), Faraday constant, surface area of GCE and the current controlled by the diffusion of glucose from the bulk solution to the electrode/solution interface.
55,56 On substituting the corresponding values into the Cottrell equation, the diffusion coefficient of glucose was calculated as 6.54 × 10
−10 cm
2 s
−1.
Table 1 summarizes a comparison of different electrochemical enzyme-less sensors using graphitic nitride and nickel sulfide based combinations for glucose sensing that indicated that the developed NiS/S-g-C3N4 sensor shows similar sensitivity and selectivity to previously reported materials.
Table 1 Various modified electrodes and their analytical performance in non-enzymatic glucose sensinga
CME |
Reaction method |
Determination techniques involved |
Linear range (μM to mM) |
Sensitivity (μA mM−1 cm−2) |
LOD (μM) |
Ref. |
CME – chemically modified electrode; CB – carbon black; NiS – nickel sulfide; CV – cyclic voltammetry; Amp I–t – amperometry; g-C3N4 – graphitic carbon nitride; Fe2O3 – iron oxide; Cu – copper; Ni – nickel; CA – chronoamperometry; EIS – electrochemical impedance spectroscopy; Au–NiCo2O4/Ni – gold–nickel cobalt oxide/nickel; Ni3S2 – nickel sulfide; rGO – reduced graphene oxide; PVP – polyvinylpyrrolidone; DPV – differential pulse voltammetry; LSV – linear sweep voltammetry.
|
g-C3N4/Fe2O3–Cu composite |
Non enzymatic |
CV, Amp I–t |
0.6–2.0 |
— |
0.3 |
57
|
Bulk Ni |
Non enzymatic |
CV, EIS, CA, Amp I–t |
0.5 to 4 |
2900 |
13 |
30
|
g-C3N4 nanosheets |
Enzymatic |
CV, EIS, Amp I–t |
50–2 |
21.7 |
5 |
40
|
3D Ni3S2 nanosheets/Ni foam |
Non enzymatic |
CV, EIS, CA, Amp I–t |
0.005–3.0 |
6148.0 |
1.2 |
31
|
Ni3S2/carbon nanotube |
Non enzymatic |
CV, EIS, CA, Amp I–t |
30 to 0.5 |
345 |
1 |
58
|
g-C3N4 nanosheets |
Non enzymatic |
CV, EIS, Amp I–t |
1 to 12 |
— |
11 |
32
|
NiS/rGO nanohybrid |
Non enzymatic |
CV, LSV, Amp I–t |
50–1.7 |
— |
10 |
59
|
PVP–NiS |
Non enzymatic |
CV, DPV, Amp I–t |
0.2–2.97 |
1013.76 |
4.6 |
60
|
Au–NiCo2O4/Ni foam |
Non enzymatic |
CA, Amp I–t |
0.005–0.045 |
44.86 |
2.64 |
26
|
Hierarchical Ni3S2 electrode |
Non enzymatic |
CV, EIS, Amp I–t |
0.0005–3 |
16 460 |
0.82 |
33
|
NiS/S-g-C
3
N
4
|
Non enzymatic
|
CV, LSV, Amp I–t
|
0.1 to 2.1
|
80
|
1.5
|
This work
|
Selectivity is an important property in sensor development for practical applications. The anti-inference effect with other common interfering chemicals present in the serum constituents was tested with glucose. Amperometric measurements were used to monitor the selective detection of glucose (200 μM) in the presence of important biomolecules (20 μM) like ascorbic acid, dopamine, uric acid, lactose, and sucrose, and metal ions like magnesium (Mg2+) and calcium (Ca2+) (400 μM). The glucose detection by amperometric signal was not significantly impacted in the presence of interfering compounds. The obtained current values for the interference compounds are given in the bar diagram in Fig. 7. This clearly shows the good selectivity of the present modified electrode. Further, the reproducibility of the present sensor was evaluated by amperometric measurements using the NiS/S-g-C3N4 modified GC electrode. The reproducibility of the measurement was verified by fabricating four different NiS/S-g-C3N4 modified electrodes and checking their glucose response (300 μM). The current deviation for the four electrodes is 4.4%, indicating that the present electrode fabrication and the glucose detection procedure are highly reproducible (Fig. S7†). The stability of the sensor was also checked by measuring the oxidation peak current density towards 300 μM glucose after 7 days. The current response was observed to decrease only to 92.77% after 7 days of storage, clearly indicating that the developed sensor system is highly stable (Fig. S8†).
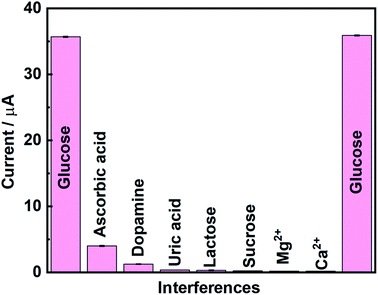 |
| Fig. 7 Selectivity studies using the NiS/S-g-C3N4 modified electrode with various interference compounds. | |
4. Conclusion
The present work demonstrated a simple and modest electrode fabrication for the non-enzymatic electrochemical sensing of glucose with nickel sulfide and sulfur-doped graphitic carbon nitride (NiS/S-g-C3N4) in a 0.1 M NaOH medium. The NiS/S-g-C3N4 nanohybrid material was prepared by a simple single-step pyrolysis method. The morphological study reveals that the successful incorporation of NiS nanoparticles on the S-g-C3N4 nanosheet surface benefits the sensor electrode by promoting electrocatalytic processes and electrical conductivity. In order to show the enhanced electrocatalytic behavior of glucose, the LSV technique was utilized in which a linear increase in the anodic peak current due to glucose oxidation was observed. The prepared NiS/S-g-C3N4 nanohybrid material was utilized in glucose sensing and showed a limit of detection of 1.5 μM (S/N = 3) with a sensitivity of 80 μA mM−1 cm−2 and the response time of the fabricated sensor was close to 5 s. The presence of inorganic ions and organic substances did not interfere with the glucose sensing. Other biosensor requirement studies and the test results were also satisfactory. The NiS/S-g-C3N4 nanohybrid material could expand the various opportunities of the electrochemical sensing of glucose.
Conflicts of interest
The authors declare no competing financial interest.
Acknowledgements
Dr A. Pandikumar thanks DST-SERB, New Delhi for the financial support through Early Career Research (ECRA) Award (SERB File No.: ECR/2017/001758). Mr S. Vinoth and Dr K. S. Shalini Devi are recipients of DST-Inspire Fellowship (IF170687) and DST-SERB National Post-Doctoral Fellowship (PDF/2019/001764), respectively. The financial support from the DST-Inspire faculty award is gratefully acknowledged by Dr S. Radhakrishnan (DST/INSPIRE/04/2015/002259). The authors thank CIF, CSIR-CECRI for the instrument facilities.
References
- J. Wang, Chem. Rev., 2008, 108, 814–825 CrossRef CAS PubMed
.
- J. Gonzalo-Ruiz, M. Asunción Alonso-Lomillo and F. Javier Muñoz, Biosens. Bioelectron., 2007, 22, 1517–1521 CrossRef CAS PubMed
.
- J. C. Pickup, F. Hussain, N. D. Evans, O. J. Rolinski and D. J. S. Birch, Biosens. Bioelectron., 2005, 20, 2555–2565 CrossRef CAS PubMed
.
- M. S. Steiner, A. Duerkop and O. S. Wolfbeis, Chem. Soc. Rev., 2011, 40, 4805–4839 RSC
.
- J. Luo, P. Luo, M. Xie, K. Du, B. Zhao, F. Pan, P. Fan, F. Zeng, D. Zhang, Z. Zheng and G. Liang, Biosens. Bioelectron., 2013, 49, 512–518 CrossRef CAS PubMed
.
- D. Li, J. Wu, P. Wu, Y. Lin, Y. Sun, R. Zhu, J. Yang and K. Xu, Sens. Actuators, B, 2015, 213, 295–304 CrossRef CAS
.
- Y. Z. Wang, H. Zhong, X. R. Li, G. Q. Liu, K. Yang, M. Ma, L. L. Zhang, J. Z. Yin, Z. P. Cheng and J. K. Wang, Sens. Actuators, B, 2016, 230, 449–455 CrossRef CAS
.
- A. Heller and B. Feldman, Chem. Rev., 2008, 108, 2482–2505 CrossRef CAS PubMed
.
- J. Wu and F. Yin, J. Electroanal. Chem., 2013, 694, 1–5 CrossRef CAS
.
- P. Si, Y. Huang, T. Wang and J. Ma, RSC Adv., 2013, 3, 3487–3502 RSC
.
- J. Li and X. Lin, Biosens. Bioelectron., 2007, 22, 2898–2905 CrossRef CAS PubMed
.
- B. Wu, G. Zhang, S. Shuang and M. M. F. Choi, Talanta, 2004, 64, 546–553 CrossRef CAS PubMed
.
- K. Han, Z. Wu, J. Lee, I. S. Ahn, J. W. Park, B. R. Min and K. Lee, Biochem. Eng. J., 2005, 22, 161–166 CrossRef CAS
.
- T. J. Ohara, R. Rajagopalan and A. Heller, Anal. Chem., 1994, 66, 2451–2457 CrossRef CAS PubMed
.
- F. Kurniawan, V. Tsakova and V. M. Mirsky, Electroanalysis, 2006, 18, 1937–1942 CrossRef CAS
.
- Y. Mu, D. Jia, Y. He, Y. Miao and H. L. Wu, Biosens. Bioelectron., 2011, 26, 2948–2952 CrossRef CAS PubMed
.
- Y. Ding, Y. Wang, L. Su, M. Bellagamba, H. Zhang and Y. Lei, Biosens. Bioelectron., 2010, 26, 542–548 CrossRef CAS PubMed
.
- C. Li, Y. Su, S. Zhang, X. Lv, H. Xia and Y. Wang, Biosens. Bioelectron., 2010, 26, 903–907 CrossRef CAS PubMed
.
- X. M. Chen, Z. J. Lin, D. J. Chen, T. T. Jia, Z. M. Cai, X. R. Wang, X. Chen, G. N. Chen and M. Oyama, Biosens. Bioelectron., 2010, 25, 1803–1808 CrossRef CAS PubMed
.
- R. A. Soomro, O. P. Akyuz, R. Ozturk and Z. H. Ibupoto, Sens. Actuators, B, 2016, 233, 230–236 CrossRef CAS
.
- I. H. Yeo and D. C. Johnson, J. Electroanal. Chem., 2000, 484, 157–163 CrossRef CAS
.
- C. Barrera, I. Zhukov, E. Villagra, F. Bedioui, M. A. Páez, J. Costamagna and J. H. Zagal, J. Electroanal. Chem., 2006, 589, 212–218 CrossRef CAS
.
- L. Özcan, Y. Şahin and H. Türk, Biosens. Bioelectron., 2008, 24, 512–517 CrossRef PubMed
.
- K. Wang, J. J. Xu and H. Y. Chen, Biosens. Bioelectron., 2005, 20, 1388–1396 CrossRef CAS PubMed
.
- P. Kannan, F. Chen, H. Jiang, H. Wang, R. Wang, P. Subramanian and S. Ji, Analyst, 2019, 144, 4925–4934 RSC
.
- K. K. Naik, A. Gangan, B. Chakraborty and C. S. Rout, Analyst, 2018, 143, 571–579 RSC
.
- Z. H. Ibupoto, A. Nafady, R. A. Soomro, Sirajuddin, S. T. Hussain Sherazi, M. I. Abro and M. Willander, RSC Adv., 2015, 5, 18773–18781 RSC
.
- X. Luo, M. Huang, D. He, M. Wang, Y. Zhang and P. Jiang, Analyst, 2018, 143, 2546–2554 RSC
.
- I. Gualandi, Y. Vlamidis, L. Mazzei, E. Musella, M. Giorgetti, M. Christian, V. Morandi, E. Scavetta and D. Tonelli, ACS Appl. Nano Mater., 2019, 2, 143–155 CrossRef CAS
.
- X. Niu, M. Lan, H. Zhao and C. Chen, Anal. Chem., 2013, 85, 3561–3569 CrossRef CAS PubMed
.
- H. Huo, Y. Zhao and C. Xu, J. Mater. Chem. A, 2014, 2, 15111–15117 RSC
.
- J. Tian, Q. Liu, C. Ge, Z. Xing, A. M. Asiri, A. O. Al-Youbi and X. Sun, Nanoscale, 2013, 5, 8921–8924 RSC
.
- S. Kim, S. H. Lee, M. Cho and Y. Lee, Biosens. Bioelectron., 2016, 85, 587–595 CrossRef CAS PubMed
.
- Y. J. Yang, J. Zi and W. Li, Electrochim. Acta, 2014, 115, 126–130 CrossRef CAS
.
- L. Liu, H. Lv, C. Wang, Z. Ao and G. Wang, Electrochim. Acta, 2016, 206, 259–269 CrossRef CAS
.
- H. Zhang, Q. Huang, Y. Huang, F. Li, W. Zhang, C. Wei, J. Chen, P. Dai, L. Huang, Z. Huang, L. Kang, S. Hu and A. Hao, Electrochim. Acta, 2014, 142, 125–131 CrossRef CAS
.
- Y. Zhang, X. Bo, A. Nsabimana, C. Luhana, G. Wang, H. Wang, M. Li and L. Guo, Biosens. Bioelectron., 2014, 53, 250–256 CrossRef CAS PubMed
.
- J. Tian, Q. Liu, C. Ge, Z. Xing, A. M. Asiri, A. O. Al-Youbi and X. Sun, Nanoscale, 2013, 5, 8921–8924 RSC
.
- M. Sadhukhan and S. Barman, J. Mater. Chem. A, 2013, 1, 2752–2756 RSC
.
- K. J. Tian, H. Liu, Y. P. Dong, X. F. Chu and S. B. Wang, Colloids Surf., A, 2019, 581, 123808 CrossRef CAS
.
- P. K. Kannan and C. S. Rout, Chem.–Eur. J., 2015, 21, 9355–9359 CrossRef CAS PubMed
.
- S. Vinoth, P. M. Rajaitha and A. Pandikumar, Compos. Sci. Technol., 2020, 195, 108192 CrossRef CAS
.
- M. H. Vu, M. Sakar, C. C. Nguyen and T. O. Do, ACS Sustainable Chem. Eng., 2018, 6, 4194–4203 CrossRef CAS
.
- B. Jansi Rani, N. Dhivya, G. Ravi, S. S. Zance, R. Yuvakkumar and S. I. Hong, ACS Omega, 2019, 4, 10302–10310 CrossRef CAS PubMed
.
- H. Sha, Y. Zhang, Y. Wang, H. Ke, X. Xiong and N. Jia, Biosens. Bioelectron., 2019, 124–125, 59–65 CrossRef CAS PubMed
.
- H. Zhao, H. Yu, X. Quan, S. Chen, Y. Zhang, H. Zhao and H. Wang, Appl. Catal., B, 2014, 152–153, 46–50 CrossRef CAS
.
- M. Jourshabani, Z. Shariatinia, G. Achari, C. H. Langford and A. Badiei, J. Mater. Chem. A, 2018, 6, 13448–13466 RSC
.
- S. Vinoth, P. Sampathkumar, K. Giribabu and A. Pandikumar, Ultrason. Sonochem., 2019, 104855 Search PubMed
.
- S. Vinoth, R. Ramaraj and A. Pandikumar, Mater. Chem. Phys., 2020, 245, 122743 CrossRef CAS
.
- K. Wang, Q. Li, B. Liu, B. Cheng, W. Ho and J. Yu, Appl. Catal., B, 2015, 176–177, 44–52 CAS
.
- M. B. Zakaria, C. Li, Q. Ji, B. Jiang, S. Tominaka, Y. Ide, J. P. Hill, K. Ariga and Y. Yamauchi, Angew. Chem., 2016, 128, 8566–8570 CrossRef
.
- J. Wen, X. Li, H. Li, S. Ma, K. He, Y. Xu, Y. Fang, W. Liu and Q. Gao, Appl. Surf. Sci., 2015, 358, 204–212 CrossRef CAS
.
- P. K. Sonkar, V. Ganesan, S. A. John, D. K. Yadav and R. Gupta, RSC Adv., 2016, 6, 107094–107103 RSC
.
- J. Li and X. Lin, Sens. Actuators, B, 2007, 126, 527–535 CrossRef CAS
.
- B. Dinesh, K. S. Shalini Devi and U. M. Krishnan, ACS Appl. Bio Mater., 2019, 2, 1740–1750 CrossRef CAS
.
- J. Li, H. Xie and L. Chen, Sens. Actuators, B, 2011, 153, 239–245 CrossRef CAS
.
- L. Liu, M. Wang and C. Wang, Electrochim. Acta, 2018, 265, 275–283 CrossRef CAS
.
- T. W. Lin, C. J. Liu and C. S. Dai, Appl. Catal., B, 2014, 154–155, 213–220 CrossRef CAS
.
- S. Radhakrishnan and S. J. Kim, RSC Adv., 2015, 5, 44346–44352 RSC
.
- S. Jana, G. Mondal, B. C. Mitra, P. Bera, B. Chakraborty, A. Mondal and A. Ghosh, New J. Chem., 2017, 41, 14985–14994 RSC
.
Footnote |
† Electronic supplementary information (ESI) available. See DOI: 10.1039/d0na00172d |
|
This journal is © The Royal Society of Chemistry 2020 |