DOI:
10.1039/C9MT00248K
(Paper)
Metallomics, 2020,
12, 92-103
Anti-cancer activities of metal-based complexes by regulating the VEGF/VEGFR2 signaling pathway and apoptosis-related factors Bcl-2, Bax, and caspase-9 to inhibit angiogenesis and induce apoptosis†
Received
4th October 2019
, Accepted 4th November 2019
First published on 4th November 2019
Abstract
Three novel single crystals of the metal-based complexes Cu-1, Cu-2, and Co-1 were obtained and characterized. Compared with Cu-2 and Co-1, Cu-1 showed remarkable activities of anti-cervical cancer, anti-cisplatin-resistant non-small cell lung cancer and anti-angiogenesis by downregulating the expressions of important proteins in the VEGF/VEGFR2 signaling pathway to inhibit angiogenesis and cancer cell proliferation, induce apoptosis, and suppress migration and metastasis. Moreover, Cu-1 dramatically inhibited the expression of the anti-apoptotic protein Bcl-2 and up-regulated the expressions of the proapoptotic proteins caspase-9 and Bax to induce the apoptosis of tumor cells, simultaneously decreasing the density of endothelial cells to inhibit tumor angiogenesis in cisplatin-resistant tumors.
Significance to metallomics
Copper is a metal element that is needed in life, and the human body needs a few milligrams of copper every day. Compared with cisplatin, copper-based active compounds are less likely to cause metal enrichment and toxicity and are regarded as the succedaneum of cisplatin drugs. In this manuscript, Cu-1 was shown to be a potential anti-cancer and anti-angiogenesis copper-containing drug, and it was effective for those human cancers which have already become resistant to cisplatin. This manuscript would be a good contribution to the rational development of metal complexes as promising anticancer drugs. All authors have agreed to contribute to “Metallomics”.
|
Introduction
Malignant tumors can produce endothelial cells to generate their own vasculatures for providing nutrition1,2 and promoting tumorigenesis, invasion, deterioration, and metastasis;3,4 tumor cells cause overexpressed vascular endothelial growth factor (VEGF), which promotes angiogenesis in tumors.5,6 Therefore, finding out new drugs for inhibiting angiogenesis and simultaneously killing tumor cells for oncotherapy is immediately required to enhance the therapeutic efficacy. However, the search for anti-cancer drugs has been beset with many challenges. Angiogenesis is a complex multistep process.7 It has been reported that copper is an essential cofactor during the angiogenesis process, where it can induce the production of cytokines, endothelial cell proliferation and migration.8–11 Animal cancer studies, including head and neck cancer,12 hepatocellular carcinoma,13 breast cancer14 and lung cancer,15 have demonstrated that the effects of copper chelation on the suppression of angiogenesis and the decrease in the tumor volume are not cell type specific.7 However, these studies only establish an association between copper levels and angiogenesis, where the reduction in copper levels significantly inhibits angiogenesis and the anti-angiogenic potential of copper-based compounds has barely been focused on. In fact, copper-based active compounds have been greatly considered for the development of anti-cancer drugs;16–20 they are regarded as the succedaneum of cisplatin drugs21,22 and are likely to have different mechanisms of action, biodistribution, and toxicity compared with the platinum drugs used currently.23 Furthermore, our previous studies found that the mixed-ligand copper-based complexes [Cu2(C10H8N2)(C12H11O4N)2(CH3OH)2]·2H2O24 and [Cu(C12H8N2)(C12H11O4N)]·4H2O25 have multi-functional anti-cancer activities in vitro. They exhibit good cell uptake under physiological conditions and induce imbalance of the level of reactive oxygen species (ROS) in tumor cells and vascular endothelial cells, simultaneously activating the Erk1/2 and Akt signaling pathways at a low dose level; this can result in the inhibition of tumor cell proliferation, promotion of induced apoptosis, suppression of angiogenesis by the inhibition of migration, proliferation and tube formation, and slight induction of apoptosis for the vascular endothelial cells. Moreover, they induce the G-rich sequence of VEGF DNA to form mixed parallel/antiparallel G-quadruplex structures and stabilize these G-quadruplex structures to repress the expression of the VEGF gene, which can further suppress angiogenesis. In addition, we found that the other two mixed-ligand copper(II)-based complexes [Cu(C17H14NO4Br)(C12H8N2)]2·2CH3OH·H2O and [Cu(C17H14NO4Br)(C10H8N2)]·2CH3OH inhibited angiogenesis and suppressed the growth of cervical cancer by down-regulating the expressions of important proteins such as FAK, Akt and Erk1/2 or their phosphorylated proteins p-FAK, p-Akt, and p-Erk1/2, respectively, downstream of the VEGF/VEGFR2 signaling pathway.26 The Penumaka Nagababu team27 reported that the copper(II) polypyridyl complexes exhibit significant anti-cancer and anti-angiogenic potentials by generating intracellular ROS and upregulating the level of the proapoptotic protein BAX. Studies have shown that copper-based complexes have potential applications as the inhibitors of angiogenesis and tumorigenesis.
Taurine (2-aminoethanesulfonic acid) is a special β-amino acid in the human body. It has been reported that a copper-based complex with a Schiff base ligand derived from taurine exhibits good anti-cancer biological activity.28 In the present study, we synthesized three novel metal-based complexes Cu-1, Cu-2, and Co-1 by using a copper(II) or cobalt(II) salt coordinated with a Schiff base ligand derived from taurine. The synthesis routes are shown in Scheme 1. Their structures were characterized. The anti-cervical cancer and anti-angiogenic potentials of these three complexes were investigated in vitro by a cell viability study, scratch wound healing assay, tube formation assay in HUVECs, angiogenesis assay in vivo chick embryo, and apoptosis analysis by flow cytometry. Cu-1 significantly inhibited cervical cancer and cisplatin-resistant NSCLC growth in C33A and A549/DDP xenograft nude mice, respectively. The molecular mechanisms of anti-cancer and anti-angiogenesis were manifested by investigating the expressions of the apoptosis-related proteins Bcl-2, Bax, and caspase-9 as well as the expressions of the important proteins FAK, Akt and Erk1/2 or their phosphorylated proteins p-FAK, p-Akt, and p-Erk1/2, respectively, downstream of the VEGF/VEGFR2 signaling pathway. Additionally, Cu-1 inhibited angiogenesis in vivo, as manifested by immunohistochemical staining assays using CD31 to label the endothelial cells in tumor tissues.
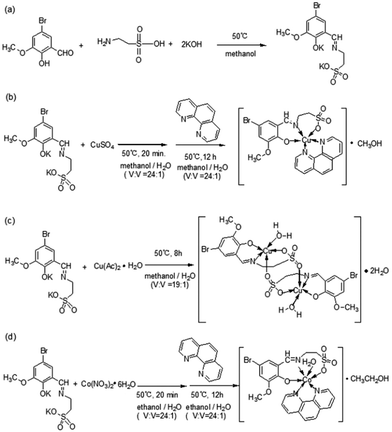 |
| Scheme 1 Synthesis routes for the metal-based complexes Cu-1, Cu-2, and Co-1. (a) The synthesis route for ligand 2-[(3-methoxy-5-bromine-2-hydroxyl-benzylidene)-amino]-ethanesulfonic acid potassium (K2C10H10NO5SBr). (b) The synthesis route for Cu-1. (c) The synthesis route for Cu-2. (d) The synthesis route for Co-1. | |
Results and discussion experimental
Syntheses and crystal structures of complexes
Cu-1.
In a mixed solvent containing methanol and water (V
:
V = 24
:
1), green crystals of Cu-1 (Fig. 1(a) and (b)) were obtained by the reaction of copper sulfate with 1,10-phenanthroline and the Schiff base potassium K2C10H10NO5SBr, which was synthesized with taurine, 5-bromo-3-methoxy-salicylaldehyde, and potassium hydroxide in anhydrous methanol. The crystallographic structural analysis revealed that Cu-1 crystallized in the monoclinic crystal system with the space group P2(1)/c and the following cell parameters: a = 15.516 (8) Å, b = 13.3471 (7) Å, c = 11.7999 (9) Å, β = 100.625 (19)°; V = 2401.8 (12) Å3, Z = 4, Dc = 1.692 g cm−3, F(000) = 1236, μ = 2.705 mm−1, S = 1.032, R = 0.0647, wR (for all) = 0.1820, formula = C23H22BrCuN3O6S, Mr = 611.95. The other crystal data are given in Table S1 (ESI†), and selected bond lengths and angles are listed in Table S3 (ESI†). The atomic coordinates and other parameters have been deposited in the Cambridge Crystallographic Data Center (1566125†). As shown in Fig. 1(b), Cu-1 has irregular hexagonal-block morphology. Cu-1 was a mononuclear copper(II)-based coordination compound consisting of one Cu(II) cation, one bivalent Schiff base ligand anion (C10H10NO5SBr)2−, one neutral ligand Phen, and one methanol solvent molecule, [Cu(C10H10NO5SBr)(Phen)]·CH3OH, namely, [2-({[5-bromo-2-(hydroxy)-3-methoxyphenyl]methylidene}amino)ethane-1-sulfonato]-(1,10-phenanthroline)-copper methanol solvate (Fig. 1(a)). In the structure of Cu-1, the Cu(II) cation was five coordinated with two O atoms (O(2), O(3)) and one N atom (N(1)) of the Schiff base ligand anion and two N atoms (N(2), N(3)) of Phen to form polyhedral geometry between square-pyramidal and trigonal-bipyramidal, with a tau parameter (176.34–147.98)/60 = 0.472,29 which was different from that of a previously reported copper-based complex with a similar ligand.26 Two N atoms [N(1) and N(3)] were at the apical position with a bond angle N(1)Cu(1)N(3) = 176.34(18)° in the polyhedral geometry arrangement. The coordinated bond lengths [1.913(4)–2.202(5) Å] were in the normal range. Cu-1 formed a two-dimensional layer structure in the ac plane (Fig. S1(c), ESI†) and ab plane (Fig. S1(d), ESI†) by van der Waals forces and π⋯π stacking interactions, with the center distances of 3.834 Å between the heterocycle centre and benzene centre, 3.691 Å between the heterocycle centre and heterocycle centre (symmetry codes: (i) −x, −y + 1, −z + 2) (Fig. S1(e), ESI†), and 3.832 Å between the heterocycle centre and heterocycle centre (symmetry codes: (ii) x, −y + 3/2, z + 1/2), thus highlighting the indispensable contribution to the stability of the Cu-1 frameworks.
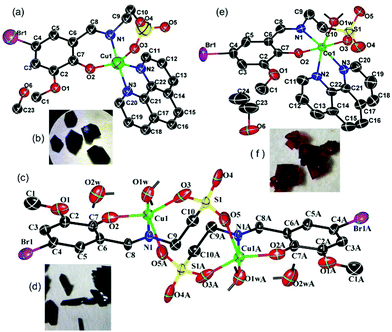 |
| Fig. 1 (a, c and e) Molecular views of Cu-1, Cu-2, and Co-1 using an atom labelling scheme, respectively, and the ellipsoids were drawn at the 50% probability level [in (c); symmetry codes: −x, −y, −z + 1]. (b, d and f) The single crystal morphologies of Cu-1, Cu-2, and Co-1, respectively. Some H atoms were omitted for clarity. | |
Cu-2.
The green columnar crystals of Cu-2 (Fig. 1(c) and (d)) were obtained by the reaction of copper acetate hydrate and the Schiff base potassium K2C10H10NO5SBr in a mixed solvent containing methanol and water (V
:
V = 19
:
1). Cu-2 crystallized in the monoclinic crystal system with the space group P2(1)/c and the following cell parameters: a = 12.248(3) Å, b = 7.6422(18) Å, c = 15.829 (4) Å, β = 104.923 (4)°, V = 1413.7 (6) Å3, Z = 2, Dc = 2.022 g cm−3, F(000) = 868, μ = 4.493 mm−1, S = 1.059, R = 0.0332, wR (for all) = 0.0896, formula = C20H28Br2Cu2N2O14S2, Mr = 871.46. The other crystal data are given in Table S2 (ESI†); selected bond lengths and angles are listed in Table S3 (ESI†), and hydrogen bond lengths and angles are listed in Table S4 (ESI†). The atomic coordinates and other parameters have been deposited in the Cambridge Crystallographic Data Center (1565798†). As shown in Fig. 1(c), Cu-2 is a binuclear and centrosymmetric copper(II)-based coordination compound consisting of two Cu(II) cations, two bivalent Schiff base ligand anions (C10H10NO5SBr)2−, two coordinated water molecules, and two solvent water molecules, [Cu2(C10H10NO5SBr)2(H2O)2]·2H2O, namely, diaqua-{μ-[2-({[5-bromo-2-(hydroxy)-3-methoxyphenyl]methylidene}amino)ethane-1-sulfonato]}-{μ-[2-({[5-bromo-2-(hydroxy)-3-methoxyphenyl]methylidene}amino)ethane-1-sulfonato]}-di-copper dihydrate. In the crystal structure of Cu-2, each Cu1(II) cation was five coordinated with two O atoms (O(2), O(3)) and one N atom (N(1)) of the Schiff base ligand anion, one O atom (O(5A)) of the other Schiff base ligand anion, and one O1W atom of the water molecule to form polyhedral geometry between square-pyramidal and trigonal-bipyramidal, with a tau parameter = 0.3635.29 The N atom (N(1)) and O atom (O(1W)) were at the apical position with a bond angle N(1)Cu(1)O(1W) = 177.23(7)°in the polyhedral geometry arrangement. In this binuclear copper(II)-based coordination compound, two Cu(II) cations were bridged by sulfo groups. The coordinated bond lengths [1.982(2)–2.333(3) Å] were in the normal range. Cu-2 formed a stratified two-dimensional structure in the ab plane (Fig. S2(c), ESI†) via abundant hydrogen bonding interactions (Table S4, ESI†).
Co-1.
The red crystals of Co-1 (Fig. 1(e) and (f)) were obtained by the reaction of cobalt nitrate hexahydrate with Phen and the Schiff base as a co-ligand in a mixed solvent containing ethanol and water (V
:
V = 24
:
1). Co-1 crystallized in the monoclinic crystal system with the space group I2/a and the following cell parameters: a = 15.5234 (7) Å, b = 19.2687 (5) Å, c = 19.1761 (8) Å, β = 112.933 (5)°; V = 5282.5 (3) Å3, Z = 8, Dc = 1.608 g cm−3, F(000) = 2600, μ = 2.290 mm−1, S = 1.012, R = 0.0428, wR (for all) = 0.1103, formula = C24H26BrCoN3O7S, Mr = 639.38. The crystal data are given in Table S1 (ESI†); selected bond lengths and angles are listed in Table S3 (ESI†), and hydrogen bond lengths and angles are listed in Table S4 (ESI†). The atomic coordinates and other parameters have been deposited in the Cambridge Crystallographic Data Center (1565799†). Co-1 was red with rhombic morphology (Fig. 1(f)). As shown in Fig. 1(e), Co-1 is a mononuclear cobalt(II)-based coordination compound consisting of one Co(II) cation, one bivalent Schiff base ligand anion (C10H10NO5SBr)2−, one Phen molecule, one coordinated water molecule, and one ethanol solvent molecule, [Co(C10H10NO5SBr)(Phen)(H2O)]·CH3CH2OH, namely, aqua-[2-({[5-bromo-2-(hydroxy)-3-methoxyphenyl]methylidene}amino)ethane-1-sulfonato]-(1,10-phenanthroline)-cobalt ethanol solvate. In the structure of Co-1, the Co(II) cation was six coordinated with two O atoms (O(2),O(3)) and one N atom (N(1)) of the Schiff base ligand anion, two N atoms (N(2), N(3)) of Phen, and one O atom (O(1w)) to form a slightly distorted octahedral arrangement. The coordinated bond lengths [1.991(2)–2.178(2) Å] were in the normal range. Co-1 formed a two-dimensional network structure by hydrogen bond interactions and van der Waals forces in the bc plane (Fig. S3(c), ESI†); it formed “butterfly”-shaped molecular aggregates and further formed a two-dimensional network structure by hydrogen bond interactions and van der Waals forces in the ab plane (Fig. S3(d), ESI†).
Cytotoxicity assay
To evaluate the anti-cervical cancer and anti-angiogenesis efficacy, we investigated the cytotoxicity of the three complexes Cu-1, Cu-2, and Co-1 against cervical cancer cell lines (C33A and HeLa) and non-cancerous human umbilical vein endothelial cells (HUVECs). As shown in Fig. 2(a), at a concentration of 3.0 μM, Cu-1 inhibits HUVECs by (23.28 ± 2.74)%, HeLa cells by (100.00 ± 0.53)%, and C33A cells by (68.92 ± 1.42)%; Cu-1 (6.5 μM) inhibited C33A cells by (83.00 ± 4.30)%, indicating that Cu-1 exhibited significant cytotoxicity in a dose-dependent manner. Compared with cisplatin, Cu-1 exhibited stronger cytotoxicity because the IC50 values for Cu-1 were (5.25 ± 0.034), (2.99 ± 0.28), and (1.96 ± 0.01) μM for HUVECs, C33A, and HeLa, respectively; the IC50 values for cisplatin were (6.82 ± 0.34), (21.87 ± 0.46), and (5.72 ± 0.18) μM for HUVECs, C33A, and HeLa, respectively. Suramin acted as a positive control for angiogenesis inhibitors with IC50 of (4.96 ± 0.27) μM for HUVECs, which was close to that of Cu-1; this showed that Cu-1 significantly inhibited the survival of C33A, HeLa, and HUVECs, revealing its anti-cervical cancer and anti-angiogenic nature at a lower concentration. As shown in Fig. 2(b), Cu-2 exhibits weaker cytotoxic responses than Cu-1. Cu-2 (46 μM) inhibited HUVECs by (20.75 ± 2.78)%, HeLa by (84.75 ± 3.07)%, and C33A by (27.33 ± 4.26)%; Cu-2 (69 μM) inhibited C33A by (52.00 ± 1.20)%, with the IC50 values of (72.19 ± 1.66), (71.54 ± 3.74), and (32.21 ± 2.99) μM for HUVECs, C33A, and HeLa (Fig. 2(d)), respectively, thus suggesting a preference for HeLa cells. Among the three complexes, Co-1 exhibited the weakest cytotoxic responses against cervical cancer cell lines. As shown in Fig. 2(c), Co-1 (60 μM) inhibits HUVECs by (61.11 ± 2.94)%, HeLa by (54.71 ± 3.01)%, and C33A by (26.76 ± 2.00)%; Co-1 (95 μM) inhibited C33A by (40.85 ± 2.23)%, with the IC50 values of (41.97 ± 0.81), (125.20 ± 7.87), and (62.65 ± 3.39) μM for HUVECs, C33A, and HeLa (Fig. 2(d)), respectively, thus suggesting a preference for HUVECs. These results revealed that Cu-1 exhibited better cytotoxic responses than Cu-2 and Co-1, suggesting its anti-cervical cancer and anti-angiogenic nature; also, the copper-based complex exhibited a stronger cytotoxic response than the cobalt-based complex for the same ligands. Moreover, the mixed-ligand Phen enhanced the cytotoxicity of the copper-based complex for the same ligands and similar coordination patterns.
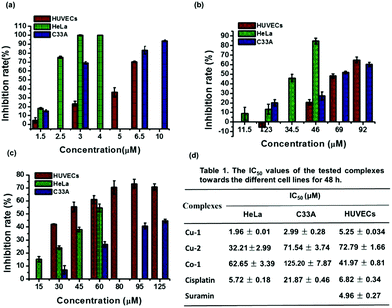 |
| Fig. 2 Cell inhibition rate assays of HUVECs, HeLa, and C33A cell lines treated with various concentrations of Cu-1, Cu-2, and Co-1 for 48 h using the MTT method, respectively. (a) Cu-1; (b) Cu-2; (c) Co-1. (d) The table of the IC50 values of the tested complexes towards different cell lines for 48 h treatment. Values are mean ± SD of three independent experiments. | |
Moreover, we investigated the cytotoxicity of Cu-1 against a non-small cell lung cancer (NSCLC) cell line (A549/DDP), which is resistant to cisplatin. After treatment with Cu-1 or DDP for 24 h, the IC50 values of the A549/DDP cells were (5.76 ± 0.19) and (486.53 ± 35.92) μM, respectively, showing that Cu-1 served as an effective cytotoxic agent to the A549/DDP cells.
Analysis of apoptosis by flow cytometry
In order to investigate the abilities of apoptosis and injury induced by Cu-1, Cu-2, and Co-1, the treated C33A cells, HeLa cells, and HUVECs were double stained with Annexin V/PI and then subjected to flow cytometric analysis. As shown in Fig. 3 and Fig. S4 (ESI†), after the cells were incubated with various concentrations of Cu-1, Cu-2, and Co-1 for 20 h and 40 h, respectively, the rates of apoptosis and injury significantly increased in dose- and time-dependent manners. After the cells were incubated with Cu-1 for 40 h, Cu-1 (4.9 μM) induced efficient apoptosis by 57.1% and injury by 10.9% in the C33A cells; Cu-1 (4.1 μM) induced efficient apoptosis by 90.2% and cellular injury by 7.3% in HeLa cells, and Cu-1 (6.5 μM) induced indistinctive apoptosis only by 13.4% and injury by 1.6% in HUVECs, indicating that Cu-1 induced apoptosis of cervical cancer cells (HeLa and C33A) to a greater extent than that for HUVECs. This significant effect could be attributed to the higher cytotoxic effect of Cu-1 on cancer cells than on non-cancerous endothelial cell lines. Moreover, the apoptotic rate of the C33A cells incubated with Cu-2 (68.8 μM) for 40 h was 46.6%; the apoptotic rate of the HeLa cells incubated with Cu-2 (45.9 μM) for 40 h was 89.5%, and the apoptotic rate of HUVECs incubated with Cu-2 (68.8 μM) for 40 h was only 7.8%, thus showing that Cu-2 induced apoptosis in the cervical cancer cells at a higher concentration compared with Cu-1. This indicated that in the structure of Cu-1, the ligand Phen strengthened the cytotoxicity of Cu-1. Cu-1 and Co-1 had the same ligands and similar coordination patterns. However, among these three complexes, Co-1 showed the weakest ability to induce apoptosis by 16.8% and injury by 7.7% for the C33A cells; it showed almost no apoptosis and damage effects on HUVECs after the treatment with Co-1 (93.8 μM) for 40 h, indicating that the choice of metal ions primarily influenced the cytotoxicity of the coordination complexes. The copper ions showed better cytotoxic effects than cobalt ions. These results supported the anti-cancer activity of Cu-1 and Cu-2 through the induction of the apoptotic pathway.
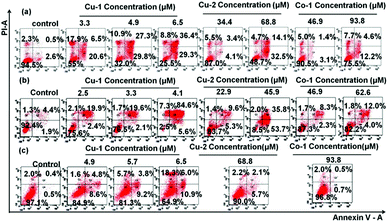 |
| Fig. 3 C33A cells, HeLa cells, and HUVECs were double stained with annexin V/PI, respectively. The induced apoptosis of the C33A cells (a), HeLa cells (b), and HUVECs (c) was analysed by flow cytometry after the cells were treated with different concentrations of Cu-1, Cu-2, and Co-1 for 40 h. These figures show one of the three independent experiments. | |
Analyses of inhibiting VEGF-induced migration, tube formation of HUVECs, and chick embryo angiogenesis
The inhibition of angiogenesis has become a potential therapeutic strategy for cancer treatment. To investigate the anti-angiogenic properties of Cu-1, Cu-2, and Co-1, we performed a VEGF-induced HUVEC migration assay, tube formation assay in vitro, and chick embryo angiogenesis assay. HUVECs have a natural tendency for migration, which is a vital process in angiogenesis. The results revealed that VEGF (20 ng mL−1) strongly stimulated migration (Fig. 4(a) and Fig. S5, ESI†) and tube formation of inactivated HUVECs (Fig. 4(b) and Fig. S6, ESI†). VEGF-induced cell migration and tube formation were far greater than that of the untreated control group. However, Cu-1, Cu-2, and Co-1 effectively inhibited the migration and tube formation of inactivated HUVECs in a dose-dependent manner after VEGF stimulation with less cell migration and capillary structure formation. In particular, Cu-1 (4.9 μM) was more advantageous than suramin (5.0 μM), which was a positive control, in terms of inhibiting VEGF-induced migration and capillary structure formation of HUVECs. Cu-2 (46.0 μM) exhibited a stronger inhibition effect than Co-1 (62.0 μM) and suramin (5.0 μM). Moreover, Fig. 4(c) and Fig. S7 (ESI†) reveal that VEGF (100 ng per egg) strongly stimulates the germination of arterious branches in the chick embryo, whereas low concentrations of Cu-1 (2.5–10.0 μg per egg) inhibit the germination of arterious branches. The inhibition effect of Cu-1 (5.0–10 μg per egg) was stronger than that of suramin (10.0 μg per egg) after VEGF stimulation in the chick embryo. Cu-2 (40.0 μg per egg) exhibited a stronger inhibition effect than Co-1 (40.0 μg per egg), which was close to that of suramin (10.0 μg per egg). Thus, our findings suggested that Cu-1 blocked tumour vasculogenesis better than Cu-2 and Co-1 by inhibiting cancer-derived endothelial cell migration and tube formation as well as by partly inducing apoptosis to prevent the endothelial cells from self-vasculature. In contrast, Co-1 was inferior to Cu-2 in terms of inhibiting angiogenesis and inducing apoptosis. These findings indicated that the choices of the metal ion and different ligands affected the anti-cancer functions of the metal-based complexes and prompted us to investigate the anti-cancer effect of Cu-1 in vivo as well as the origin of such a behaviour.
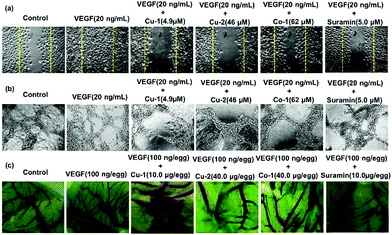 |
| Fig. 4 (a) Effects of Cu-1, Cu-2, Co-1, and suramin on the HUVEC migration in wound migration assays in the presence of VEGF stimulation. (b) Effects of Cu-1, Cu-2, Co-1, and suramin on the HUVEC tube formations in the presence of VEGF stimulation. (c) Angiogenic development of the arterial endpoint in the chick embryo was stimulated by VFGF and was inhibited by Cu-1, Cu-2, Co-1, and suramin. | |
In vivo antitumor efficacy in C33A xenograft nude mice
To investigate the anti-tumor activity of Cu-1 in vivo, we used Cu-1 to treat the C33A xenograft nude mice by intraperitoneal injections with the administration of 100 μg kg−1 Cu-1 every 3 days in the first 27 days and then every 2 days during the following 15 days. As shown in Fig. 5(e), after 42 days, we found that there were no substantial body weight changes observed after the administration of Cu-1. The average weight of the nude mice in the control group slightly increased from (23.81 ± 1.04) to (24.40 ± 0.61) g, whereas the average weight of the Cu-1-treated nude mice slightly increased from (23.78 ± 1.12) to (25.79 ± 0.50) g, without a vomiting phenomenon, thus showing that Cu-1 was well-tolerated at the tested dose level. However, Cu-1 significantly suppressed the tumor volume (Fig. 5(a), (b) and (d)) and reduced the tumor weight (Fig. 5(c)). The average tumor volume in the control mice increased from (61.43 ± 5.13) to (1759.82 ± 357.98) mm3 after 42 days, whereas the average tumor volume in the Cu-1-treated mice increased from (57.42 ± 1.87) to (153.31 ± 40.07) mm3. The average tumor weight in the control group was (1.76 ± 0.24) g, whereas the average tumor weight in the Cu-1-treated group was only (0.24 ± 0.042) g, which demonstrated significant inhibition in the tumor growth. These results showed that Cu-1 could significantly suppress the development of cervical cancer in vivo.
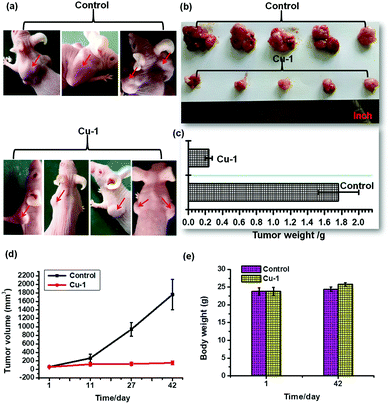 |
| Fig. 5 Cu-1 inhibited tumor growth in vivo. The C33A cells were injected subcutaneously into 5–6 week old SCID female mice. After the tumors were established (∼55 mm3), the mice were randomly assigned to two groups that received different therapies. (a) The images of the tumors before being dislodged from the nude mice. (b) The images of the dislodged tumors from the two mice groups after the last intraperitoneal injection. (c) The weights of the dislodged tumors from the two mice groups after the last intraperitoneal injection. (d) Tumor volumes at different times after tumor inoculation. The tumor volumes were calculated by V = lw2/2 (mean SD, n = 4 animals per group). (e) The average mouse body weight was monitored with an electronic balance on the first and last day of treatment. The photographs were taken by a mobile phone (OPPO R9t). | |
In vivo cisplatin-resistant NSCLC growth inhibition efficacy
We evaluated the anti-cancer and anti-angiogenesis efficacy in human cancers resistant to cisplatin. The A549/DDP xenograft model of nude mice was established, and the nude mice were treated with cisplatin and Cu-1 intraperitoneally with a dose of 150 μg kg−1 (every 2 days) for 30 days, and the physiological saline-treated group served as the control. The average tumor weights in the saline- and cisplatin-treated groups were significantly higher than that of the Cu-1-treated group, with (1.567 ± 0.241), (1.349 ± 0.311), and (0.317 ± 0.019) g for the saline-treated group, cisplatin-treated group, and Cu-1-treated group, respectively, thus showing that Cu-1 significantly inhibited the cisplatin-resistant NSCLC growth in vivo. The tumor tissues were used for immunohistochemical analysis.
Immunohistochemical analysis
Angiogenesis is a critical step in tumor development and metastasis formation. Inhibiting angiogenesis can delay tumor growth. We further investigated the histological change and decrease in vascular endothelial cell density in tumors by HE staining and CD31 immumohistochemical staining assays, respectively. As shown in Fig. 6, compared with the control- and DDP-treated groups, in the Cu-1-treated group, the number of cells is significantly reduced and higher levels of apoptosis with chromatin condensation or nuclei fragmentation can be observed. Eosin staining was enhanced (Fig. 6(a)), and the results of the CD31 staining showed that the density of vascular endothelial cells dramatically decreased in the Cu-1-treated group (Fig. 6(b)). These results verified that Cu-1 remarkably inhibited NSCLC tumor growth and angiogenesis in vivo.
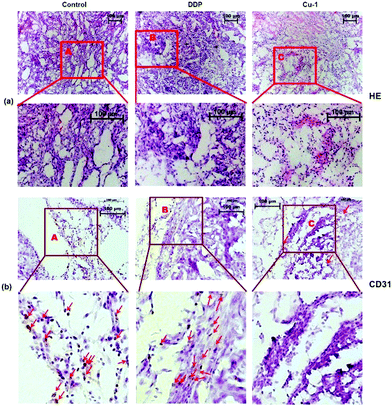 |
| Fig. 6 (a) The morphological changes of the A549/DDP tumor tissues were detected by HE staining after treatment with physiological saline, cisplatin, and Cu-1. (b) Representative photographs of the CD31 immumohistochemical staining of vascular endothelial cells with the CD31 antibody in A549/DDP tumor tissues after treatment with physiological saline, cisplatin, and Cu-1. The brown cells are vascular endothelial cells labeled by CD31. The control group was physiological saline. (HE staining images: 10 × 10 magnification; CD31 staining images: 10 × 20 magnification). | |
Bcl-2 is an anti-apoptotic gene, and Bax is a pro-apoptotic gene. If down-regulated, Bcl-2 can induce apoptosis. The overexpression of Bcl-2 is often the expression of drug resistance. The upregulation of Bax can promote apoptosis. Moreover, Bax can bind to Bcl-2 to form a dimer to weaken the anti-apoptotic ability of Bcl-2. When Bcl-2 is dominant, the cells will survive. However, when Bax is dominant, it causes apoptosis. In addition, caspase-9 is one of the members of the caspase family, and the upregulation of the pro-apoptotic gene caspase-9 will promote apoptosis. To further evaluate whether Cu-1 can eliminate the drug resistance caused by cisplatin and induce tumor cells apoptosis, the tumor tissues treated with saline, cisplatin, and Cu-1 were used to investigate the expressions of the proteins caspase-9, Bcl-2, and Bax by immunohistochemical analysis. As shown in Fig. 7, the expressions of caspase-9 and Bax are obviously up-regulated in the Cu-1-treated group compared with that in the saline- and cisplatin- treated groups, whereas the expression of Bcl-2 is significantly reduced in the Cu-1-treated group. There was no significant difference in the saline- and cisplatin-treated groups because of the drug resistance caused by cisplatin, showing that Cu-1 removed the drug resistance caused by cisplatin and promoted apoptosis by down-regulating the expression of Bcl-2 and up-regulating the expressions of caspase-9 and Bax in the A549/DDP xenograft tumors.
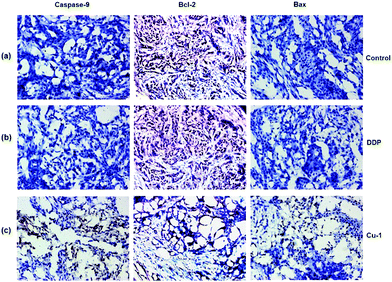 |
| Fig. 7 The expressions of caspase-9, Bcl-2, and Bax in A549/DDP tumor tissues were detected by immunohistochemistry. (a–c) Represent the saline-, DDP-, and Cu-1-treated groups, respectively. Scale bar: 200 μm and 10 × 20 magnification. | |
Western blotting assay in C33A cells, HeLa cells, and HUVECs
The VEGF/VEGFR2 signal transduction pathway plays a key role in regulating and controlling the survival, proliferation, and migration of tumor cells and tumor angiogenesis. As shown in Fig. 8(d), the proteins VEGFR2, Akt, Erk1/2, and FAK as well as their corresponding phosphorylated proteins p-VEGFR2, p-Akt, p-Erk1/2, and p-FAK are important signal molecules. Cu-1, Cu-2, and Co-1 were investigated for their effects on the expressions of these proteins by subjecting the C33A cells, HeLa cells, and HUVECs to Western blot analysis. Fig. 8(a) shows that the expressions of the proteins VEGFR2/p-VEGFR2, FAK/p-FAK, AKT, and Erk1/2 significantly decrease in a dose-dependent manner after the C33A cells are treated with Cu-1 (1.6–4.1 μM, 1.0–2.5 μg mL−1) for 24 h, indicating that Cu-1 suppressed the development of the C33A cell cervical cancer by down-regulating the expressions of these proteins to inhibit proliferation, induce apoptosis, and antagonize invasion and metastasis as well as inhibit tumor angiogenesis, resulting in an effective anti-cancer function. To our surprise, repeated experiments demonstrated that Cu-1 over-expressed the levels of p-AKT and p-Erk1/2 after treatment with Cu-1 when the concentration was greater than 3.3 μM, but it significantly down-regulated the expressions of AKT and Erk1/2. These results were similar to previous findings26 but differed from our previous results, where it down-regulated the expressions of p-AKT and p-Erk1/2 in a dose-dependent manner.24,25 According to the results of apoptosis in the flow cytometry analysis, we speculated that the over-expressions of p-Akt and p-Erk1/2 might promote apoptosis, and suggest that apoptosis also might be related to other factors such as the caspase family, Bcl-2, and Bax, as well as injury or death factors, etc. Compared with Cu-1, the treatment with Cu-2 (23–69 μM) and Co-1 (31–125 μM) at higher concentrations did not show an obvious effect on the expressions of total proteins Akt, Erk1/2, and FAK. However, Cu-2 markedly inhibited the expressions of the proteins VEGFR2/p-VEGFR2, p-FAK, p-Akt, and p-Erk1/2; Co-1 markedly inhibited the expressions of the proteins VEGFR2/p-VEGFR2, p-FAK, and p-Akt to suppress the growth of the C33A cell cervical cancer. Fig. 8(b) shows that the expressions of VEGFR2 and p-VEGFR2 are not significantly affected in the HeLa cells treated with Cu-1 (1.6–4.9 μM, 1.0–3.0 μg mL−1) for 24 h. However, the expressions of the FAK and p-FAK proteins were significantly inhibited when the concentration increased, and the expressions of AKT and Erk1/2 were slightly inhibited in a dose-dependent manner. Similarly, Cu-1 (4.9 μM) intensively increased the expressions of the proteins p-AKT and p-Erk1/2 after treatment in the HeLa cells, which was the same as those in the C33A cells, suggesting that p-AKT and p-Erk1/2 were not the only key factors for Cu-1 to induce apoptosis in the HeLa cells. Higher concentrations of Cu-2 (23.0–69.0 μM) and Co-1 (31.0–94.0 μM) inhibited the expressions of the proteins p-VEGFR2 and p-FAK to suppress the growth of HeLa cells; also, p-Akt and p-Erk1/2 were over-expressed. In particular, Cu-1, Cu-2, and Co-1 showed their respective characteristics in regulating and controlling the expressions of the proteins VEGFR2/p-VEGFR2, Akt/p-Akt, Erk1/2/p-Erk1/2, and FAK/p-FAK in non-cancerous HUVECs and in the cancerous C33A and HeLa cells. As shown in Fig. 8(c), Cu-1, Cu-2, and Co-1 do not affect the expressions of the proteins VEGFR2, Akt, Erk1/2, and FAK after HUVECs are treated with varied concentrations of Cu-1, Cu-2, and Co-1 for 24 h; in contrast, the expressions of the phosphorylated proteins p-VEGFR2, p-FAK, p-Akt, and p-Erk1/2 are dramatically inhibited in a dose-dependent manner without an overexpressed phenomenon, indicating that Cu-1, Cu-2, and Co-1 down-regulated the expressions of the phosphorylated proteins p-VEGFR2, p-FAK, p-AKT, and p-Erk1/2 to inhibit angiogenesis, which was similar to our previous results.24,25 The results suggested that non-cancerous and cancerous cells had incompletely identical responses to metal-based complexes. Cu-1 was superior to Cu-2 and Co-1 to inhibit C33A cervical cancer by down-regulating the expressions of the proteins VEGFR2/p-VEGFR2, FAK/p-FAK, AKT, and Erk1/2 in the C33A cells and to inhibit HeLa cervical cancer by down-regulating the expressions of the proteins FAK/p-FAK, AKT, and Erk1/2 in HeLa cells. These three metal-based complexes suppressed angiogenesis to enhance the anti-cancer effect by down-regulating the expressions of the phosphorylated proteins p-VEGFR2, p-FAK, p-AKT, and p-Erk1/2 in vascular endothelial cells.
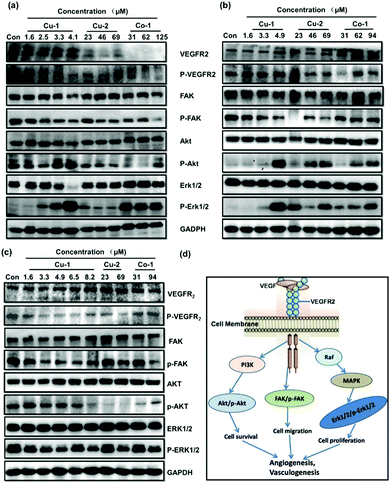 |
| Fig. 8 Effects of Cu-1, Cu-2, and Co-1 on the intracellular signals in cells. Effect of Cu-1, Cu-2, and Co-1 on the expressions of VEGFR2, p-VEGFR2, FAK, p-FAK, Akt, p-Akt, Erk1/2, p-Erk1/2, and GAPDH in C33A cells (a), HeLa cells (b), and HUVECs (c) after treatment for 24 h. GAPDH was detected as a loading control. (d) Three important signaling pathways that are associated with angiogenesis, apoptosis, tumor metastasis, and cell proliferation were downstream of VEGF/VEGFR2. | |
Western blotting assay In A549/DDP cells
To investigate the expressions of the proteins VEGFR2, Akt, Erk1/2, and FAK and their corresponding phosphorylated proteins p-VEGFR2, p-Akt, p-Erk1/2, and p-FAK in the A549/DDP cells, Cu-1 and DDP were subjected to the A549/DDP cells for Western blot analysis. Fig. 9 shows that DDP does not significantly change the expressions of the proteins VEGFR2, FAK, AKT, and Erk1/2 and their corresponding phosphorylated proteins p-VEGFR2, p-FAK, p-AKT, and p-Erk1/2 in the A549/DDP cells in the range of 15.0–25.0 μM. However, Cu-1 (4.1–12.2 μM) obviously down-regulated the expressions of the proteins VEGFR2, FAK, AKT, and Erk1/2 and their phosphorylation proteins p-VEGFR2, p-FAK, and p-AKT in the A549/DDP cells, thus indicating that Cu-1 inhibited angiogenesis and delayed A549/DDP tumor growth by down-regulating the expressions of these proteins. To our surprise, according to the results of repeated experiments, we found that the expressions of Erk1/2 and p-Erk1/2 were weaker in the A549/DDP cells, whereas a low concentration of Cu-1 (4.1 μM) significantly changed and enhanced the expressions of the proteins Erk1/2 and p-Erk1/2. Then, Cu-1 down-regulated the expressions of Erk1/2 and p-Erk1/2 with the increase in concentration, indicating that Cu-1 and DDP had different molecular mechanisms in inhibiting the A549/DDP tumor growth.
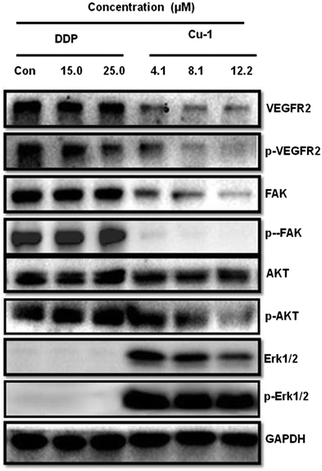 |
| Fig. 9 Effects of Cu-1 and DDP on the intracellular signals from the A549/DDP cells after treatment with DDP and Cu-1 for 30 h, respectively. GAPDH was detected as a loading control. | |
Experimental
Instruments and materials (please see ESI†)
Synthesis of ligand (K2C10H10NO5SBr).
According to the ref. 30, 5-bromo-2-hydroxy-3-methoxy-benzaldehyde (2.3104 g, 10.0 mmol) was dissolved in absolute methanol (15 mL) and then added to an absolute methanol solution (25 mL) containing taurine (1.2514 g, 10.0 mmol) and KOH (1.1220 g, 20.0 mmol) to form a yellow solution at 50 °C. This yellow reaction mixture was stirred at 50 °C for 3 h. Subsequently, a large proportion of methanol was evaporated from the yellow solution. A yellow solid was precipitated and then cooled for filtration. The yellow product was washed using absolute methanol and ether, respectively. The yellow solid (yield: 80.5%, based on taurine) was collected and dried under vacuum for 2 days for further use. IR(cm−1): 3462.22(–OH), 2951.09(–C–H), 2904.80(–C–H), 2831.50(–C–H), 1639.49(s; vs(–CH
N–)), 1622.13, 1508.33, 1471.69, 1438.90, 1396.46, 1247.94(–SO3), 1217.08(–SO3), 1205.51(–SO3), 1174.65(–SO3), 1099.43, 1043.49(–SO3), 989.48, 866.04, 742.59, 677.01, 576.72, 530.42, 495.71, 412.77 cm−1 (Fig. S9, ESI†).
Synthesis of Cu-1.
A mixed solution containing K2C10H10NO5SBr (0.0824 g, ∼0.20 mmol) and anhydrous CuSO4 (0.0559 g, 0.35 mmol) was stirred at 50 °C for 20 minutes in a 25 mL mixed solvent containing methanol and water (V
:
V = 24
:
1). Then, Phen (0.0402 g, 0.2 mmol) was added into the mixed solution under continuous stirring at 50 °C for 8 h. Subsequently, the resulting mixed reagent was filtrated after cooling, and the bright green filtrate was left at room temperature. Some bottle-green crystals were obtained after some days, giving hexagonal-block morphology single crystals that were suitable for X-ray diffraction. For C23H22BrCuN3O6S, namely, [Cu(C10H10NO5SBr)(C12H8N2)]·CH3OH, Mr = 611.95, anal. calcd, %: C, 45.14; H, 3.62; N, 6.87; found, %: C, 45.10; H, 3.61; N, 6.86. IR (KBr, ν, cm−1): 3643.53, 3387.00, 3064.89, 2929.87, 2831.50, 1625.99 (s; vs (–CH
N–)), 1587.42, 1519.91, 1456.26, 1433.11, 1323.17, 1236.37, 1211.30, 1157.22, 1103.28, 1070.49, 1035.77, 981.77, 848.68, 785.03, 729.09, 680.87, 644.22, 594.08, 522.71, 499.56, 468.78 cm−1 (Fig. S10, ESI†). The single crystal productivity was 69.52% based on Phen. The X-ray powder diffractogram of Cu-1 was identical to the simulated diffractogram generated from the single crystal X-ray diffraction data (Fig. S13(a), ESI†). Liquid chromatography-mass spectrometry (DMSO/CH3OH): m/z 579.94 [M − CH3OH] +, m/z 580.95 [M − CH3OH +1] +, m/z 582.95 [M − CH3OH + 2] +, m/z 578.95 [M − CH3OH − 1] +, and m/z 577.95 [M − CH3OH − 2]+ (Fig. S14, ESI†).
Synthesis of Cu-2.
A mixed solution containing K2C10H10NO5SBr (0.0817 g, ∼0.20 mmol) and Cu(Ac)2·H2O (0.0509 g, 0.25 mmol) in 20 mL mixed solvent containing methanol and water (V
:
V = 19
:
1) was stirred at 50 °C for 8 h. Subsequently, the cooled resulting reagent was filtrated, and the yellow-green filtrate was left at room temperature. Many bottle-green crystals were obtained after some days, giving prismatic morphology single crystals that were suitable for X-ray diffraction. For C20H28Br2Cu2N2O14S2, namely, [Cu2(C10H10NO5SBr)2(H2O)2]·2H2O, Mr = 871.46, anal. calcd, %: C, 27.56; H, 3.24; N, 3.21. Found, %: C, 27.53; H, 3.25; N, 3.20. IR (KBr, ν, cm−1): 3398.57, 3116.97, 3018.60, 2947.23, 1629.85 (s; vs(–CH
N–)), 1587.42, 1541.12, 1475.54, 1456.26, 1442.75, 1334.74, 1246.02, 1230.58, 1211.30, 1178.51, 1159.22, 1078.21, 1041.56, 758.02, 682.80, 594.08, 549.71, 497.63, 443.63 cm−1 (Fig. S11, ESI†). The single crystal productivity was 74.08% based on the ligand K2C10H10NO5SBr. The X-ray powder diffractogram of Cu-2 was identical to the simulated diffractogram generated from the single crystal X-ray diffraction data (Fig. S13(b), ESI†). Liquid chromatography-mass spectrometry (DMSO/CH3OH): m/z 399.87 [Cu(C10H10NO5SBr)]2−: 1/2 [M − 4H2O]2−; m/z 397.88 [Cu(C10H10NO5SBr) − 2]2−: 1/2 [M − 4H2O − 2]2−; m/z 335.95 [(C10H10NO5SBr) − 1]2−: 1/2 [M − 4H2O − 2Cu]2−; m/z 337.95 [(C10H10NO5SBr) + 1]2−: 1/2 [M − 4H2O − 2Cu]2− (Fig. S15, ESI†).
Synthesis of Co-1.
A mixed solution containing K2C10H10NO5SBr (0.0828 g, ∼0.20 mmol) and Co(NO3)2.6H2O (0.0733 g, 0.25 mmol) in 25 mL mixed solvent containing ethanol and water (V
:
V = 24
:
1) was stirred at 50 °C for 20 minutes to obtain a red solution. Then, Phen (0.0394 g, 0.2 mmol) was added into the aforementioned solution to react together for 12 h at a 50 °C constant temperature. The resulting mixed reagent was cooled and filtrated, and the red filtrate was left at room temperature. Many red crystals were obtained after some days, giving rhombic morphology single crystals that were suitable for X-ray diffraction. For C24H26BrCoN3O7S, namely, [Co(C10H10NO5SBr)(C12H8N2)(H2O)]·CH3CH2OH, Mr = 639.38, anal. calcd, %: C, 45.08; H, 4.10; N, 6.57. Found, %: C, 45.07; H, 4.11; N, 6.55. IR (KBr, ν, cm−1): 3314.00, 3404.36, 1625.99 (s; vs(–CH
N–)), 1516.05, 1448.54, 1423.47, 1315.4, 1234.44, 1174.65, 1147.65, 1097.50, 1039.63, 852.54, 750.31, 729.09, 636.51, 548.85, 495.71, 426.27 cm−1 (Fig. S12, ESI†). The single crystal productivity was 79.17% based on Phen. The X-ray powder diffractogram of Co-1 was identical to the simulated diffractogram generated from the single crystal X-ray diffraction data (Fig. S13(c), ESI†). Liquid chromatography-mass spectrometry (DMSO/CH3OH): m/z 574.89: [M − CH3CH2OH–H2O]+; m/z 572.90: [M − CH3CH2OH–H2O − 2]+; m/z 590.86: [M − CH3CH2OH–H2O − 2]+ + NH4+; and m/z 596.60: [M − CH3CH2OH–H2O − 1]+ + Na+ (Fig. S16, ESI†).
X-ray crystallography.
A suitable dimension single crystal of Cu-1, Cu-2, and Co-1 was selected for the measurement. The data were collected on a four-circle CCD diffractometer equipped with mirror-monochromatized Mo Kα radiation (λ = 0.71073 Å) in the omega scan mode. For data collection, cell refinement, and data reduction, computations were performed with CrysAlisPro, Agilent Technologies, Version 1.171.37.31. The structures of Cu-1, Cu-2, and Co-1 were solved by direct methods using SHELXL-97 and refined by full matrix least-squares on F2 using the SHELXL 97 program. The non-hydrogen atoms were assigned by anisotropic displacement parameters in the refinement. The hydroxyl groups were geometrically positioned and refined using a mixed model. All H atoms bonded to C atoms and hydroxyl groups were calculated hydrogens, and other H atoms bonded to O atoms from water molecules were geometrically positioned and refined using a riding model, with C–H = 0.9300 Å for aromatic [Uiso(H) = 1.2 Ueq(C)], C–H = 0.9700 Å for secondary methylene [Uiso(H) = 1.2 Ueq(C)], C–H = 0.9600 Å for methyl [Uiso(H) = 1.5 Ueq(C)], O–H = 0.8200 Å for hydroxyl [Uiso(H) = 1.5 Ueq(O)], and O–H = 0.846(9)–0.8507 Å for water [Uiso(H) = 1.5 Ueq(O)]. All of the crystal data were deposited in the Cambridge Crystallographic Data Center.†
Cell viability assays.
Cell viability was determined by the MTT method. At the exponential growth phase, cells were diluted to (7–8) × 104 cells per mL with the appropriate medium, respectively, and were seeded in 96-well plates at a volume of 100 μL per well with six duplicates. The other empty wells were filled with 1 × PBS. The cells were incubated at 37 °C in 5% CO2 for 12 h and were treated with various concentrations of the complex (100 μL per well) diluted with the appropriate medium without FBS. The medium and drug-free control samples were prepared simultaneously. After incubation of the cells for up to 48 h, the MTT (20 μL, 5 mg mL−1) solution was added into each well. After further incubation for 4 h at 37 °C in 5% CO2, the medium was removed with a microsyringe. DMSO (200 μL) was added to each well. The plates were oscillated for 10 minutes, and the values for OD were analyzed by a microplate reader at a wavelength of 490 nm. The percentage growth inhibitory rate for the treated cells was calculated by (ODtested − ODmedia
control)/(ODdrug-free
control − ODmedia
control) × 100%, where OD is the mean value calculated using the data from six replicate tests. The IC50 values were determined by plotting the percentage viability versus the concentration on a logarithmic graph and by reading the concentration at which 50% of the cells were viable relative to the control.
Cell morphology assays.
At the exponential growth phase, the cells (1.0 × 105 per well) were seeded in 24-well plates and incubated for 24h. They were then treated with various concentrations of the complex for 48 h. The cell morphologies were observed by an inverted photomicroscope.
Apoptosis assays.
Apoptosis was detected with an Annexin V/PI kit purchased from BD Pharmingen (BD Bioscience) according to the manufacturer's instructions. The cells (2.0 × 105 per well) were seeded in 12-well plates and incubated for 12 h. They were then treated with various concentrations of the complex for 20 h or 40 h. To detect early and late apoptosis, both floating and adherent cells were harvested together and washed twice with 1 × PBS, centrifuged at 1000 rpm for 5 min to remove the medium, and resuspended in Binding Buffer (100 μL). Subsequently, 5 μL of Annexin V was used to stain the cells. After suspended, 5 μL of PI (propidium iodide) were used to stain cells. The suspended cells in the binding buffer were incubated for 15 min at 37 °C in the dark. Afterwards, the binding buffer (400 μL) was used to resuspend the cells again; then, the cells were analyzed by FACS Calibur (BD Bioscience).
HUVEC migration assays.
HUVECs were allowed to grow into full confluence in 6-well plates precoated with 0.1% gelatin and then were incubated with 10 μg mL−1 of mitomycin C at 37 °C and 5% CO2 for 12 h to inactivate HUVECs. The monolayer inactivated HUVECs were wounded by scratching with a 200 μL pipette tip. Fresh medium was added with various concentrations of Cu-1 (3.3–6.5 μM), Cu-2 (23.0–46.0 μM), Co-1 (31.0–62.0 μM), and suramin (6.5 μM) in the presence of VEGF (20 ng mL−1) stimulation. After 24 h incubation at 37 °C and 5% CO2, images were taken by an inverted photomicroscope with the same magnification. The numbers of cells that migrated were quantified by a manual measurement, and percentage migration was expressed using untreated wells at 100%. At least three independent experiments were performed.
HUVEC tube formation assays.
Matrigel (BD) was dissolved at 4 °C overnight according to the product description. Each well in the prechilled 96-well plates was coated with 60 μL of matrigel and then incubated at 37 °C for 30 minutes. After HUVECs were pretreated by various concentrations of Cu-1 (3.3–6.5 μM), Cu-2 (23.0–46.0 μM), Co-1 (31.0–62.0 μM), and suramin (6.5 μM) in the presence of VEGF (20 ng mL−1) stimulation for 10 h, HUVECs (4.0 × 104 cells) were seeded into each well with 200 μL of quondam medium. The VEGF control samples were tested simultaneously. After 12 h of incubation at 37 °C and 5% CO2, the HUVEC tube formations were assessed with an inverted microscope. The effect of the inhibition of tube formation was a qualitative judgment from the integrity of the tubular structure and the number of tubes formed. Tube formation percentage was expressed using untreated wells at 100%.
Chick embryo angiogenesis assays.
The chick embryo angiogenesis was used as a model system for chemically inducing and suppressing the tumor angiogenesis assay. Fertilized chicken eggs (5 eggs per group) were incubated at 37 °C and 80% humidity. On the sixth day of incubation, a square window was opened in the shell, and the chick embryo was injected with various concentrations of Cu-1 (2.5–10.0 μg per egg), Cu-2 (40.0 μg per egg), Co-1 (40.0 μg per egg), and suramin (10.0 μg per egg) in the presence of VEGF (100 ng per egg) stimulation by a micro-syringe. The VEGF (100 ng per egg) control samples were tested simultaneously. The window was sealed with a transport tape after the injection, and the eggs were returned to the incubator. After 4 days of incubation, images of the chick embryo arterious branches in each treatment group were photographed using a mobile phone (OPPO R9t). The anti-angiogenic effect of the test complexes was presented as the relative number of arterious branches, and the control group was set at 100%.
C33A xenograft mouse model.
The animal experiments were approved by the Institutional Animal Care and Use Committee of Guilin Medical University. First, 5 to 6 week-old SCID female mice were divided into four mice per group. C33A cells (5 × 106) were injected subcutaneously into the forelimb of the nude mice. After the tumors were established (∼50 mm3), Cu-1 and saline were used to treat the C33A xenograft nude mice by intraperitoneal injection with the administration of 100 μg kg−1 every 3 days for the first 27 days and every 2 days for the following 15 days. The body weight and tumor sizes of the mice were recorded at specific time intervals and the tumor sizes were determined by vernier caliper measurements and calculated as length × (width)2/2 (V = lw2/2). After 42 d, the mice with tumors were sacrificed.
A549/DDP xenograft mouse model.
The animal experiments were approved by the Animal Health and Utilization Committee of Guilin Medical College. Twenty 5 to 6-week-old severe combined immunodeficient mice were purchased from and fed at the Guilin Medical University Experimental Animal Center SPF Experimental Animal Lab. A549/DDP cells (1.0 × 107) were injected subcutaneously into the axillary arm. The male and female nude mice were fed in different cages. The A549/DDP xenograft mouse model was established after tumor formation (∼50 mm3). The nude mice were randomly divided into 3 groups and were intraperitoneally treated with saline, cisplatin, and copper-based complex (Cu-1) with a 150 μg kg−1 dose once every 2 days for 30 days. The tumor-bearing mice were sacrificed after anesthesia. After the tumors were quickly weighed, they were immediately fixed for HE staining and immunohistochemical analysis.
HE staining and immunohistochemical analysis.
Fresh tissues were fixed with a 4% paraformaldehyde solution for 24 h, dehydrated by sucrose, embedded in an optimum cutting temperature compound, and frozen rapidly. The embedding blocks were cut into 5 μm thick sections. Before staining, the frozen sections were soaked into pre-cooled acetone for 20 minutes and stained according to the Hematoxylin-Eosin (HE) Staining Kit. After treatment with 3% hydrogen peroxide in a PBS solution for 30 minutes at room temperature to quench endogenous peroxidase, 10% goat serum was used to block for 30 min. Then, the sections were incubated with primary antibodies (anti-CD31, anti-caspase-9, anti-bcl-2, and anti-bax) overnight at 4 °C and horseradish peroxidase-labeled secondary antibody at room temperature for 30 minutes. The slices were stained with the DAB Horseradish Peroxidase Color Development Kit and hematoxylin; then, photos were taken under a biomicroscope.
Western blot assays for C33A cells, HeLa cells, and HUVECs.
C33A cells, HeLa cells, and HUVECs were seeded into 100 mm plates (7.5 × 105 cells per plate) on the day before treatment. The C33A cells were exposed to various concentrations of Cu-1 (1.6–4.1 μM), Cu-2 (23.0–69.0 μM), and Co-1 (31.0–125.0 μM) for 24 h. The HeLa cells were exposed to various concentrations of Cu-1 (1.6–4.9 μM), Cu-2 (23.0–69.0 μM), and Co-1 (31.0–94.0 μM) for 24 h. The HUVECs were exposed to various concentrations of Cu-1 (1.6–8.2 μM), Cu-2 (23.0–69.0 μM), and Co-1 (31.0–94.0 μg mL−1) for 24 h Whole cell extracts were prepared using RIPA buffer supplemented with proteinase inhibitors. Total proteins were extracted from the cells with RIPA buffer and the protein concentrations were determined by a BCA assay. The whole collections were boiled for 10 min in 1 × SDS loading buffer, subsequently subjected to 5% and 10% SDS-PAGE, and transferred to a polyvinylidene difluoride (PVDF) membrane. The membranes were blocked in blocking buffer (5% nonfat drymilk/1% Tween-20 in TBS) for 2 h at room temperature and then incubated with primary antibodies (anti-GAPDH (1
:
2000), anti-Erk1/2, anti-phospho-Erk1/2, anti-Akt, anti-phospho-Akt, anti-FAK, anti-phospho-FAK, anti-VEGFR2, and anti-phospho-VEGFR2, 1
:
1000) in blocking buffer for 2 h at room temperature. The bands were then visualized using horseradish peroxidase-conjugated secondary antibodies (1
:
1000) for 1 h at room temperature, followed by an enhanced chemiluminescence system kit (Pierce Biotech, Rockford, IL) according to the manufacturer's instructions.
Western blotting analysis for A549/DDP cells.
The A549/DDP cells were inoculated for 12 h and exposed to cisplatin or Cu-1 for 30 hours. The negative control was complete media. After the cells were washed with pre-chilled PBS, the cells were lysed on ice with Ripa lysate containing 1% PMSF and 1% protein phosphatase inhibitor for 30 min, quickly scraped to one side with cell scrapers, and fully vortexed. Then, the mixture was centrifuged for 30 min at 4 °C in 13
000 rmp and the supernatant was carefully collected. The protein concentrations were determined by a BCA assay. The proteins were boiled for 10 min in 1 × SDS loading buffer for degeneration, subsequently subjected to 5% and 10% SDS-PAGE, and transferred to a PVDF membrane. The PVDF membranes were blocked with 5% BSA and 1% Tween-20 in TBS for 2 h. Primary antibodies, including anti-GAPDH, anti-Erk1/2, anti-phospho-Erk1/2, anti-Akt, anti-phospho-Akt, anti-FAK, anti-phospho- FAK, anti-VEGFR2, and anti-phospho-VEGFR2 were treated in BSA blocking buffer at room temperature for 2 h. Horseradish peroxidase conjugated secondary antibodies were then used to react for 1 h at room temperature. The bands were then visualized using Super Signal West Pico for luminescence development.
Conclusions
In summary, we synthesized three novel metal-based complexes Cu-1, Cu-2, and Co-1 and characterized their structures. In a low concentration range, Cu-1 was found to have the most significant anti-cervical cancer and anti-angiogenic efficacy, as observed by several assays in vitro. Cu-2 was inferior to Cu-1 and superior to Co-1 in exhibiting anti-cervical cancer and anti-angiogenesis potentials at higher concentrations. The remarkable anti-cervical cancer effect of Cu-1 was confirmed in xenograft nude mice models. Cu-1 compared to Cu-2 and Co-1 significantly down-regulated the expressions of the proteins VEGFR2, FAK, Akt, and Erk1/2 and their phosphorylated proteins p-VEGFR2 and p-FAK in the C33A cells; it also down-regulated the expressions of the proteins Akt, Erk1/2, FAK, and p-FAK in HeLa cells, exhibiting an anti-cervical cancer function. Simultaneously, Cu-1, Cu-2, and Co-1 remarkably down-regulated the expressions of the p-VEGFR2, p-Akt, p-Erk1/2, and p-FAK proteins in vascular endothelial cells to inhibit tumor angiogenesis and enhance the anti-cancer function, thus showing that non-cancerous and cancerous cells had incompletely identical responses to metal-based complexes. In particular, Cu-1 regulated the expressions of the phosphorylated proteins p-Akt and p-Erk1/2 in an incomplete dose-dependent manner and even overexpression in cervical cancer cells, thus suggesting that the Cu-1-induced apoptotic cervical cancer cells should be associated with other apoptotic factors such as the caspase family, Bcl-2, and Bax and injury or death factors as well as Akt/p-Akt, ERK/p-Erk1/2, etc. In addition, Cu-1 significantly exhibited cisplatin-resistant NSCLC by inhibiting the expression of Bcl-2 and up-regulating the expressions of caspase-9 and Bax, simultaneously down-regulating the expressions of the proteins VEGFR2, FAK, AKT, and Erk1/2 and their phosphorylation proteins p-VEGFR2, p-FAK, and p-AKT in the A549/DDP cells to induce the apoptosis of tumor cells and inhibit tumor angiogenesis. Moreover, the anti-cancer activity of the metal-based complexes was closely related to the choices of ligands, metal ions, and coordination patterns. The copper-based complexes were more cytotoxic than the cobalt-containing complexes. The ligand Phen was coordinated with copper ions, which could enhance the anti-cancer and anti-angiogenesis activities of the copper-based complexes. Cu-1 is a potential anti-cancer and anti-angiogenesis copper-containing drug, and it is even effective for human cancers that have become resistant to DDP. The studies revealed that the bioactivity of the metal-based complexes was relevant to the selection of ligands and metal ions. The active copper-based complexes were effective in resisting tumor growth with cisplatin resistance.
Conflicts of interest
The authors declared no competing financial interests.
Acknowledgements
This work was supported by the National Natural Science Foundation of China (No. 21661011 and 21861014), Guangxi Natural Science Foundation (No. 2016GXNSFAA380292), Guangxi Key Laboratory of Early Prevention and Treatment for Regional High Frequency Tumor, Guangxi Medical University (No. GK2014-TKF05), and the Scientific Experiment Center of Guilin Medical College, Guangxi, China.
References
- R.-V. Lucia, P. Roberto, B. Mauro, T. Matilde, I. Gloria, C. Tonia, M. Giulio, A. P. Eugenio, S. Giorgio, M. L. Luigi and D. M. Ruggero, Nature, 2010, 468, 824 CrossRef.
- W. Rong, C. Kalyani, W. Jennifer, K. Urszula, E. H. Koos, G. Adam, F. Boris, L. Margaret, B. Cameron and T. Viviane, Nature, 2010, 468, 829 CrossRef.
- L. Coultas, K. Chawengsaksophak and J. Rossant, Nature, 2005, 438, 937 CrossRef CAS.
- R. K. Jain, Nat. Med., 2003, 9, 685 CrossRef CAS.
- L. D. Ke, Y. X. Shi, S. A. Im, X. S. Chen and W. K. A. Yung, Clin. Cancer Res., 2000, 6, 2562 CAS.
- M. Grunewald, I. Avraham, Y. Dor, E. Bachar-Lustig, A. Itin, S. Jung, S. Chimenti, L. Landsman, R. Abramovitch and E. Keshet, Cell, 2006, 124, 175 CrossRef CAS.
- L. Finney, S. Vogt, T. Fukai and D. Glesne, Clin. Exp. Pharmacol. Physiol., 2009, 36, 88 CrossRef CAS.
- G.-F. Hu, J. Cell. Biochem., 1998, 69, 326 CrossRef CAS.
- C. K. Sen, S. Khanna, M. Venojarvi, P. Trikha, E. C. Ellison, T. K. Hunt and S. Roy, Am. J. Physiol., 2002, 282, H1821 CAS.
- A. Nasulewicz, A. Mazur and A. Opolski, J. Trace Elem. Med. Biol., 2004, 18, 1 CrossRef CAS.
- M. Moriguchi, T. Nakajima, H. Kimura, T. Watanabe, H. Takashima, Y. Mitsumoto, T. Katagishi, T. Okanoue and K. Kagawa, Int. J. Cancer, 2002, 102, 445 CrossRef CAS.
- C. Cox, T. N. Teknos, M. Barrios, G. J. Brewer, R. D. Dick and S. D. Merajver, Laryngoscope, 2001, 111, 696 CrossRef CAS.
- H. Yoshiji, S. Kuriyama, J. Yoshii, Y. Ikenaka, R. Noguchi, K. Yanase, T. Namisaki, M. Yamazaki, H. Tsujinoue, H. Imazu and H. Fukui, Int. J. Cancer, 2001, 94, 768 CrossRef.
- Q. Pan, C. G. Kleer, K. L. van Golen, J. Irani, K. M. Bottema, C. Bias, M. De Carvalho, E. A. Mesri, D. M. Robins, R. D. Dick, G. J. Brewer and S. D. Merajver, Cancer Res., 2002, 62, 4854 CAS.
- M. K. Khan, M. W. Miller, J. Taylor, N. K. Gill, R. D. Dick, K. Van Golen, G. J. Brewer and S. D. Merajver, Neoplasia, 2002, 4, 164 CrossRef CAS.
- K. M. Acevedo, D. J. Hayne, L. E. McInnes, A. Noor, C. Duncan, D. Moujalled, I. Volitakis, A. Rigopoulos, K. J. Barnham, V. L. Villemagne, A. R. White and P. S. Donnelly, J. Med. Chem., 2018, 61, 711 CrossRef CAS.
- A. E. Stacy, D. Palanimuthu, P. V. Bernhardt, D. S. Kalinowski, P. J. Jansson and D. R. Richardson, J. Med. Chem., 2016, 59, 4965 CrossRef CAS.
- J. Qi, Y. Zhang, Y. Gou, Z. Zhang, Z. Zhou, X. Wu, F. Yang and H. Liang, Mol. Pharmaceutics, 2016, 13, 1501 CrossRef CAS.
- J. Wang, Y. Gou, Z. Zhang, P. Yu, J. Qi, Q. Qin, H. Sun, X. Wu, H. Liang and F. Yang, Mol. Pharmaceutics, 2018, 15, 2180 CrossRef CAS.
- H.-Y. Zhang, W. Wang, H. Chen, S.-H. Zhang and Y. Li, Inorg. Chim. Acta, 2016, 453, 507 CrossRef CAS; Q. P. Huang, S. N. Zhang, S. H. Zhang, K. Wang and Y. Xiao, Molecules, 2017, 22, 1813 CrossRef.
- D. Griffith, J. P. Parker and C. J. Marmion, Med. Chem., 2010, 10, 354 CAS.
- N. P. E. Barrya and P. J. Sadler, Chem. Commun., 2013, 49, 5106 RSC.
- F. Tisato, C. Marzano, M. Porchia, M. Pellei and C. Santini, Med. Res. Rev., 2010, 30, 708 CAS.
- X.-Y. Qin, L.-C. Yang, F.-L. Le, Q.-Q. Yu, D.-D. Sun, Y.-N. Liu and J. Liu, Dalton Trans., 2013, 42, 14681 RSC.
- X.-Y. Qin, Y.-N. Liu, Q.-Q. Yu, L.-C. Yang, Y. Liu, Y.-H. Zhou and J. Liu, ChemMedChem, 2014, 9, 1665 CAS.
- X.-Y. Qin, Y.-N. Wang, X.-P. Yang, J.-J. Liang, J.-L. Liu and Z.-H. Luo, Dalton Trans., 2017, 46, 16446 RSC.
- P. Nagababu, A. K. Barui, B. Thulasiram, C. S. Devi, S. Satyanarayana, C. R. Patra and B. Sreedhar, J. Med. Chem., 2015, 58, 5226 CrossRef CAS.
- Y.-M. Jiang, S.-H. Zhang, Q. Xu and Y. Xiao, Acta Chim. Sin., 2003, 61(4), 573 CAS.
- A. W. Addison, T. N. Rao, J. Reedijk, J. van Rijn and G. C. Verschoor, J. Chem. Soc., Dalton Trans., 1984, 1349 RSC.
- X.-Y. Qin, S.-H. Zhang, Y.-M. Jiang, J.-C. Liu and J.-H. Qin, J. Coord. Chem., 2009, 62(3), 427 CrossRef CAS.
|
This journal is © The Royal Society of Chemistry 2020 |
Click here to see how this site uses Cookies. View our privacy policy here.