DOI:
10.1039/C9MO00068B
(Research Article)
Mol. Omics, 2020,
16, 73-82
Proteomics and molecular network analyses reveal that the interaction between the TAT–DCF1 peptide and TAF6 induces an antitumor effect in glioma cells†
Received
7th April 2019
, Accepted 2nd October 2019
First published on 3rd January 2020
Abstract
Glioblastoma is the most lethal brain cancer in adults. Despite advances in surgical techniques, radiotherapy, and chemotherapy, their therapeutic effect is far from significant, since the detailed underlying pathological mechanism of this cancer is unclear. The establishment of molecular interaction networks has laid the foundation for the exploration of these mechanisms with a view to improving therapy for glioblastoma. In the present study, to further explore the cellular role of DCF1 (dendritic cell-derived factor 1), the proteins bound to TAT–DCF1 (transactivator of transcription–dendritic cell-derived factor 1) were identified, and biosystem analysis was employed. Functional enrichment analyses indicate that TAT–DCF1 induced important biological changes in U251 cells. Furthermore, the established molecular interaction networks indicated that TAT–DCF1 directly interacted with TAF6 in glioma cells and with UBC in HEK293T (human embryonic kidney 293T) cells. In addition, further biological experiments demonstrate that TAT–DCF1 induced the activation of the RPS27A/TOP2A/HMGB2/BCL-2 signaling pathway via interaction with TAF6 in U251 cells. Taken together, these findings suggest that the TAT–DCF1 peptide possesses great potential for the development of glioblastoma therapy through the interaction with TAF6-related pathways and provides further theoretic evidence for the mechanisms underlying the antitumor effects of TAT–DCF1.
1. Introduction
GBM (glioblastoma) is the most common and most lethal brain malignancy in adults;1,2 therefore, it is very important to develop effective treatment methods. Although gene therapy is promising, there remain some problems in developing safe and efficient gene delivery systems.3
DCF1 (dendritic cell factor 1), also known as TMEM59, is a membrane protein that was first discovered to be involved in neural stem cell differentiation in our research.4,5 Knockout of Dcf1 leads to a decrease in dendritic complexity and dendritic spine density; in contrast, overexpression of Dcf1 can repair the function and structure of dendritic spines.6 DCF1 is widely expressed in different organelles, including lysosomes, Golgi apparatus, endoplasmic reticulum, endosomes, and mitochondria.7 Our recent data indicate that immune cell components, in particular white blood cells and monocytes, in Dcf1-knockout (KO) mice are lower than those in wild type (WT) mice (paper under revision), strongly implying that DCF1 may contribute to the normal immune response. Considering the close relationship between the immune system and tumors, the function of DCF1 was further explored from a novel angle as a potential therapy for GBM. Our previous research has shown that the full-length TAT–DCF1 peptide decreases the viability, inhibits the migration, and promotes the apoptosis of U251 cells;8 however, the underlying mechanism is still unclear, and the present paper attempted to further explore this mechanism. Thus, TAT–DCF1 peptide complexes in glioma cells were purified to explore the underlying mechanisms through proteomics and molecular network analysis.
To elucidate the mechanism, IP-mass (immunoprecipitation-mass spectrometry) was employed to detect the proteins bound to TAT–DCF1 in tumor (U251) and normal (HEK293T) cells. Moreover, gene ontology and KEGG (Kyoto Encyclopedia of Genes and Genomes) pathway enrichment of these proteins were carried out. In addition, PINs (protein interaction networks) were established, and key candidate proteins were identified. Further, the interactions between TAT–DCF1 and its partner proteins were evaluated using biological experiments. In conclusion, the present study provides a more comprehensive analysis of the related molecular mechanism underlying the antitumor effect of DCF1 in glioma cells.
2. Materials and methods
2.1 TAT
In the past 20 years, a series of cell-penetrating peptides have been carefully studied by researchers. TAT, a C-terminal peptide derived from human immunodeficiency virus HIV1, is rich in positively charged arginine, and its N-terminal peptide, GRKKR, can act as a nuclear localization signal. The sequence of TAT is TATGGCAGGAAGAAGCGGAGACAGCGACGAAGA. TAT can transfer the cargo it carries not only into the cell membrane, but also into mitochondria, and can efficiently transfer the fused protein into cultured cells, showing corresponding biological activity.
2.2 Cell culture
HEK293T cells and U251 cells were purchased from Cell Bank of Type Culture Collection of Chinese Academy of Sciences, Shanghai Institute of Cell Biology, Chinese Academy of Sciences. The cells were cultured in DMEM medium (Invitrogen, USA) supplemented with 10% FBS (Invitrogen, USA), 1% penicillin and 1% streptomycin (Invitrogen, USA). At approximately 80% confluence, the cells were digested using 0.25% trypsin solution at 37 °C for 2–5 min, and the trypsin digestion was stopped with the addition of DMEM medium with 10% FBS. The cells were split into two dishes and subsequently incubated overnight.
2.3 Cell immunofluorescence
U251 cells were seeded on a 24-well plate at a density of 1 × 105 cells per well and incubated at 37 °C with 5% CO2 for 24 h. After the old medium was discarded, fresh medium containing 175 mg L−1 TAT–DCF1 peptide was directly added to each well and the cells were cultured for a further 12 h. Subsequently, cells were rinsed with PBS, fixed in 4% paraformaldehyde for 10 min at RT (room temperature), and permeabilized with 0.1% Triton X-100 for 10 min. The cells were then blocked with 2% BSA in PBS at RT for 1 h and incubated with primary antibodies (mouse anti-(His)6 monoclonal antibody 1
:
500, Abcam, USA; rabbit anti-TAF6 monoclonal antibody, 1
:
500, Bioss, China) at 4 °C overnight. Subsequently, cells were washed 3 times with PBS, and incubated with a goat anti-mouse (1
:
500, Zemed, USA) or goat anti-rabbit (1
:
500, Zemed, USA) secondary antibody for 1 h at RT, and with DAPI (4′,6-diamidino-2-phenylindole) for 5 min at RT. Fluorescence signals at 488 nm or 594 nm were detected using a Zeiss LSM710 fluorescence microscope.
2.4 Immunoprecipitation
U251 or HEK293T cells were cultured on a 10 cm dish at 37 °C with 5% CO2 for 24 h. Cells were treated with the TAT–DCF1 peptide at a final concentration of 175 mg L−1 in fresh medium for 12 h. After the treatment, cells were washed twice with PBS and cultured with fresh medium at 37 °C with 5% CO2 for a further 48 h. The untreated control U251 or HEK293T cells were cultured in parallel. The membrane proteins were extracted using a membrane protein extraction kit (Beyotime, China), according to the manufacturer's protocol. An anti-DCF1 antibody (made in our lab) was coupled to protein A/G beads (Gibco, USA) and used to probe a membrane protein lysate. In brief, the membrane protein lysate was incubated with the anti-DCF1 antibody at 4 °C for 1 h, then the beads were added to the antigen–antibody complex and incubated at 4 °C for a further 1 h. Subsequently, the bead–antibody–antigen complex was washed three times with PBS and eluted with 2% SDS–PBS buffer at 70 °C for 10 min. The inputs, beads with no antibody, eluted samples, and HEK293T-blank and U251-blank groups without treatment of TAT–DCF1 were subjected to Western blotting using a rabbit anti-DCF1 polyclonal antibody. Meanwhile, the inputs, beads with no antibody, eluted samples, and HEK293T-TAT–DCF1 and U251-TAT–DCF1 groups treated with TAT–DCF1 were probed with a mouse anti-(His)6 antibody. The eluted proteins were analyzed by mass spectrometry at Shanghai Marjorbio Co., Ltd.
2.5 Mass spectrometry
A total of 50 μg each protein sample was resuspended in tetraethylammonium bromide (TEAB; 100 mM final concentration). The mixture was reduced with Tris(2-carboxyethyl)phosphine (TCEP; 10 mM final concentration) at 37 °C for 60 min and alkylated with iodoacetamide (IAM; 40 mM final concentration) at room temperature for 40 min in the dark. A six-fold volume of cold acetone was added to precipitate the protein at −20 °C for 4 h. After centrifugation at 10
000 × g for 20 min at 4 °C, the pellet was rinsed with 90% acetone, and trypsin was added at 1
:
50 trypsin-to-protein mass ratio and incubated at 37 °C overnight. Digested peptides were desalted with HLB and vacuum dried prior to being analyzed by nano-flow liquid chromatography tandem mass spectrometry performed on an EASY-nLC system (Thermo, USA) connected to a Q Exactive HF-X quadrupole orbitrap mass spectrometer (Thermo, USA) using a nanoelectrospray ion source. The parameters are shown in Table S1 (ESI†). The database used in the present study was Uniprot (http://www.uniprot.org), and the RAW data files were analyzed using ProteomeDiscoverer (Thermo Scientific, Version 2.1). When searching the database, the RAW file was submitted to the ProteomeDiscoverer server, the database that had been created was selected, and the database search was performed. The relevant parameters are shown in Table 1.
Table 1 Proteome Discoverer search parameters
Item |
Value |
Protein discoverer version |
2.1 |
Protein database |
Uniprot-mouse-79954-20160909.fasta |
Cys alkylation |
Carbamidomethyl |
Enzyme name |
Trypsin (full) |
Max. missed cleavage sites |
2 |
Precursor mass tolerance |
10 ppm |
Fragment mass tolerance |
0.05 Da |
2.6 Construction of the molecular signaling networks involved in the DCF1 or TAT–DCF1 complexes
All proteins were from the mass spectrometry results of the different experimental groups: HEK293T-blank, HEK293T-TAT–DCF1, U251-blank, and U251-TAT–DCF1. Biomolecular networks can be represented as graphs in which points and edges denote biomolecules and their connections, respectively. In PINs, the points are the proteins and the edges are their interactions. The object of optimizing the alignment of two networks is to obtain a mapping that best describes the similarity of the points as well as that of their connections. The connected PINs were constructed based on the STRING database,9 which involved 368, 98, 51, and 119 proteins, respectively. The number of common proteins in the compared PIN groups are listed in Fig. S1 (ESI†), among which the D values10 greater than 0.4 were selected and recognized as proteins with significant changes,11 which were 10, 1, 2, and 34, respectively. The D value describes the topology differences of a certain node in different networks. To explore the kernel differences between PINs, we ignored the proteins with D values less than 0.4, with the exception of DCF1 and TAT–DCF1, and the common links between the remaining proteins. The final differential networks that included proteins with obvious changes, and those different links among them, were constructed and visualized.
We downloaded the protein sequences from the UniProt database12 (http://www.uniprot.org/) and compared the above PINs through the Hungarian algorithm and the Greedy algorithm (HGA).13 The Point and Edge Score (PE)13 and EC (Edge Correctness)13 of the PINs were calculated and are shown in Fig. S2 (ESI†). The PE is an indicator of the similarity of biological networks, which considers both biological significance and network topology. EC is another popular indicator that pays more attention to network topology. Furthermore, to evaluate the difference between the PINs, we aligned the networks by HGA13 to calculate the similarity of these networks. The lower the values of EC and PES (point and edge score), the greater the difference between two networks. As seen from Fig. S2 (ESI†), the largest value in the first column (PE: 614.694 and EC: 0.665) indicates that the HEK293T-blank (318) and HEK293T-TAT–DCF1 (48) networks were the most similar, indicating that there was a slight difference between these two networks upon the addition of TAT–DCF1 or blank peptides. In addition, a decrease in the PE (53.122 and 45.069) and EC (0.329 and 0.184) values indicates that there was a large difference between these networks, including U251-blank (37) and U251-TAT–DCF1 (105), and HEK293T-TAT–DCF1 (86) and U251-TAT–DCF1 (107).
2.7 Western blotting
U251 cells were seeded at a density of 1 × 105 cells per well on a 24-well plate and incubated at 37 °C with 5% CO2 for 24 h. Cells were treated with the TAT–DCF1 or TAT–EGFP peptide at a final concentration of 175 mg L−1 in fresh medium for 12 h. After treatment, cells were washed twice with PBS and cultured in fresh medium at 37 °C with 5% CO2 for a further 48 h. Cells were washed twice with ice-old PBS and the total protein was extracted using lysis buffer for Western blotting and IP (Beyotime, China), according to the manufacturer's protocol. The total protein was separated by SDS-PAGE and transferred onto nitrocellulose membranes, which were then blocked with 5% BSA in PBS for 1 h at RT, and incubated with a mouse anti-GAPDH monoclonal antibody (1
:
1000), a rabbit anti-BCL-2 polyclonal antibody (1
:
1000), or a rabbit anti-BAX polyclonal antibody (1
:
1000) overnight at 4 °C. Next, infrared dye 700-conjugated affinity-purified goat anti-mouse IgG and infrared dye 800-labled goat anti-rabbit IgG secondary antibodies were added and incubated for 1 h at RT. Immunoreactive bands were detected and quantified using the LI-COR Odyssey infrared imaging system (simultaneous two-color targeted analysis) and software (LI-COR).
2.8 Total RNA extraction, cDNA synthesis, and qPCR
U251 cells were seeded at a density of 4 × 105 cells per well on 6-well plates. 12 h post-treatment with 175 mg L−1 TAT–DCF1 peptide, cells were washed twice with PBS and cultured in fresh medium at 37 °C with 5% CO2 for a further 48 h. The total RNA was extracted using a Total RNA Extraction Kit (Promega, USA), according to the manufacturer's protocol. The concentration of RNA was determined by measuring the absorbance at 260 nm, and 2 μg of RNA was used for cDNA synthesis using an RT Master Mix (TaKaRa, Japan). qPCR (quantitative real-time PCR) amplification was performed at least in triplicate using a mixture of Top Green qPCR Super Mix (Transgen, China), cDNA samples, and designated primers (Table S2, ESI†). The relative gene expression was calculated by comparing the CT value of the gene of interest with that of GAPDH, which serves as the internal control.
2.9 Animals
All animal procedures were performed in accordance with the Guidelines for Care and Use of Laboratory Animals of Shanghai University and were approved by the Animal Ethics Committee of Shanghai University.
2.10 Statistical analysis
All data were analyzed using the GraphPad Prism software. The protein expression and mRNA expression were analyzed by one-way Analysis of Variance (ANOVA). P < 0.05 was considered statistically significant.
3. Results
3.1 Proteomic analysis of the TAT–DCF1 peptide in HEK293T and U251 cells
To explore the mechanisms underlying the antitumor effects of the TAT–DCF1 peptide, IP-mass was used to detect the proteins bound to TAT–DCF1. To distinguish the bound proteins that were specific to U251 glioma cells, HEK293T cells were used as a control. Immunoprecipitation was performed to purify the TAT–DCF1 peptide complex, DCF1 was detected by Western blotting using an anti-DCF1 antibody in the HEK293T-blank (Fig. 1A) or U251-blank (Fig. 1C) groups, and TAT–DCF1 was detected using an anti-(His)6 antibody in the HEK293T-TAT–DCF1 (Fig. 1B) or U251-TAT–DCF1 (Fig. 1D) groups. The results indicate that the TAT–DCF1 peptide was successfully detected. A database search of the fragmentation spectra resulted in the identification of 384 proteins in the HEK293T-blank group, 107 proteins in the HEK293T-TAT–DCF1 group, 57 proteins in the U251-blank group, and 149 proteins in the U251-TAT–DCF1 group (data not shown). Subsequently, the mass-spectrometry results were analyzed and the biological processes for which the P-value was less than 0.05 were collected from Gene Ontology. As shown in Tables S3–S6 (ESI†), the proteins that interacted with DCF1 in the four groups were involved in the same six biological processes, including SRP-dependent cotranslational proteins – translational initiation, viral transcription, nuclear-transcribed mRNA catabolic process, nonsense-mediated decay, translation, and rRNA processing – implying the basic function of DCF1. There were, however, significant differences between HEK293T and U251 cells. For instance, establishment of protein localization to the plasma membrane, protein stabilization, and mitochondrial organization were the top three enriched biological processes in HEK293T cells. Interestingly, it has been reported that DCF1 inhibits APP (amyloid precursor protein) glycosylation14 and is located in mitochondria,15 suggesting that DCF1 may indeed be associated with protein stabilization and mitochondrial organization. Taken together, these results indicate that DCF1 may participate in these biological processes under normal circumstances. Only one of the same biological processes, namely membrane raft assembly, was enriched in U251 cells (Tables S5 and S6, ESI†), indicating a specific role for DCF1 in U251 tumor cells. However, in the U251-TAT–DCF1 group, TAT–DCF1 was shown to be related to specific biological processes such as DNA topological changes,16 apoptotic DNA fragmentation,17 and establishment of protein localization at the plasma membrane18 in U251 cells, which are all necessary for tumor apoptosis (Table S6, ESI†). In conclusion, these results indicate that TAT–DCF1 influences multiple biological processes in U251 cells that result in apoptosis.
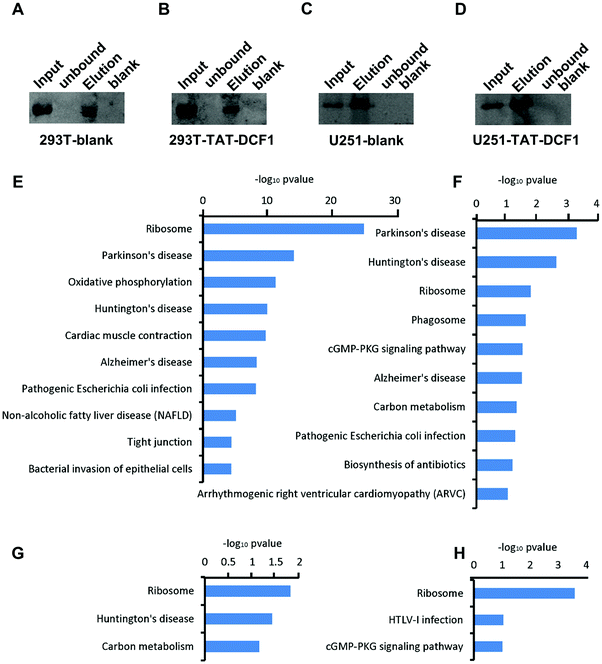 |
| Fig. 1 Immunoprecipitation and KEGG pathway enrichment analysis revealed that DCF1 plays a specific role in U251 cells. The DCF1 complex was extracted using anti-DCF1 antibody beads and detected using an anti-DCF1 antibody in HEK293T (A) or U251 (C) cells without the treatment of the TAT–DCF1 peptide. The TAT–DCF1 complex was extracted using anti-DCF1 antibody beads and detected using an anti-(His)6 antibody in HEK293T (B) or U251 (D) cells with the treatment of the TAT–DCF1 peptide. The input group is total membrane protein lysate, the unbound group is beads without DCF1 antibody, the blank group is beads with the DCF1 IgG isotype, and the elution group is the eluted sample. (E–H) KEGG pathway enrichment analysis of the proteins that interacted with DCF1 in HEK293T-blank, HEK293T-TAT–DCF1, U251-blank, and U251-TAT–DCF1 groups. | |
3.2 KEGG pathway enrichment analysis of TAT–DCF1-interacting proteins
In order to clearly distinguish the pathways that were enriched by these proteins in the four groups (HEK293T-control; HEK293T-TAT–DCF1; U251-control; and U251-TAT–DCF1), DAVID (The Database for Annotation, Visualization and Integrated Discovery) was used to analyze the enrichment of the KEGG (Kyoto Encyclopedia of Genes and Genomes) pathway as shown in Fig. 1E–H. The majority of the proteins that interacted with DCF1 were associated with neurodegenerative disease- (Parkinson's, Alzheimer's (AD) or Huntington's disease) and ribosome-related pathways in HEK293T cells (HEK293T-control and HEK293T-TAT–DCF1). However, it is noteworthy that there were slight differences between the two groups. Most of the proteins in the HEK293T-control group were enriched in ribosome-related pathways, while those in the HEK293T-TAT–DCF1 group were enriched in neurodegenerative disease-related pathways. In U251 cells, the proteins that interacted with DCF1 (U251-control and U251-TAT–DCF1) were also enriched in neurodegenerative disease- and ribosome-related pathways (Fig. 1G and H). Interestingly, the number of proteins enriched in ribosome-related pathways in the U251-TAT–DCF1 group was much more than that in the U251-control group. Furthermore, RPL23, RPL4, RPL28, and RPS27A were enriched in the ribosome-related pathway specifically in the U251-TAT–DCF1 group, implying that TAT–DCF1 may play a crucial role in U251 cells through these protein interactions. For instance, it has been reported that ribosomal protein L23 (RPL23) may be a negative regulator of cell apoptosis,18 suggesting that DCF1 mediated U251 tumor cell apoptosis by regulating the expression of RPL23. Moreover, in addition to ribosome-related pathways, proteins in the human T-lymphotropic virus type I (HTLV-I) infection-related pathway were enriched in the U251-TAT–DCF1 group. Studies have shown that proteins involved in the HTLV-I infection-related pathway are associated with cancer.19 In addition, SLC25A31, which is also enriched in the HTLV-I infection-related pathway (Fig. 1H), can exchange cytosolic ADP for matrix ATP in the mitochondria,20 the site of DCF1 localization.7 Interestingly, overexpression of SLC25A31 has been shown to display an anti-apoptotic phenotype.21 Taken together, these results indicate that the functions of DCF1 and SLC25A31 are similar, and that they may work synergistically to regulate apoptosis in U251 cells. In conclusion, the results of KEGG pathway enrichment analysis show that the mechanism of apoptosis induced by TAT–DCF1 was relatively complex and that the process of apoptosis may be under the regulation of multiple proteins and pathways.
3.3 Protein interaction networks induced by the DCF1 or TAT–DCF1 complexes in U251 or HEK293T cells
To explore the antitumor mechanism of the TAT–DCF1 complex in U251 cells, protein interaction networks mediated by TAT–DCF1 in both U251 and HEK293T cells were constructed using a computational biological systems database (STRING). To further clearly distinguish the proteins that are specifically regulated by TAT–DCF1 in U251 cells, the differentially expressed proteins networks were selected and differential interaction networks were constructed (Fig. 2A–F). As shown in Fig. 2A–C, DCF1 directly interacted with ubiquitin-related proteins in three networks: UBA52 in the HEK293T-blank network (318) and UBC in the HEK293T-TAT–DCF1 (48) and U251-blank (37) networks. It has been previously reported that ubiquitin is a highly conserved nuclear and cytoplasmic protein that plays a major role in targeting cellular proteins for degradation.22 It can be speculated that the interaction of TAT–DCF1 with ubiquitin-related proteins may exert cellular protein degradation functions. However, TAT–DCF1 directly interacted with TAF6 in the U251-TAT–DCF1 network (105) (Fig. 2D), implying an important function of TAF6 and TAT–DCF1 in U251 cells, which may interact with other genes to induce apoptosis in tumor cells.
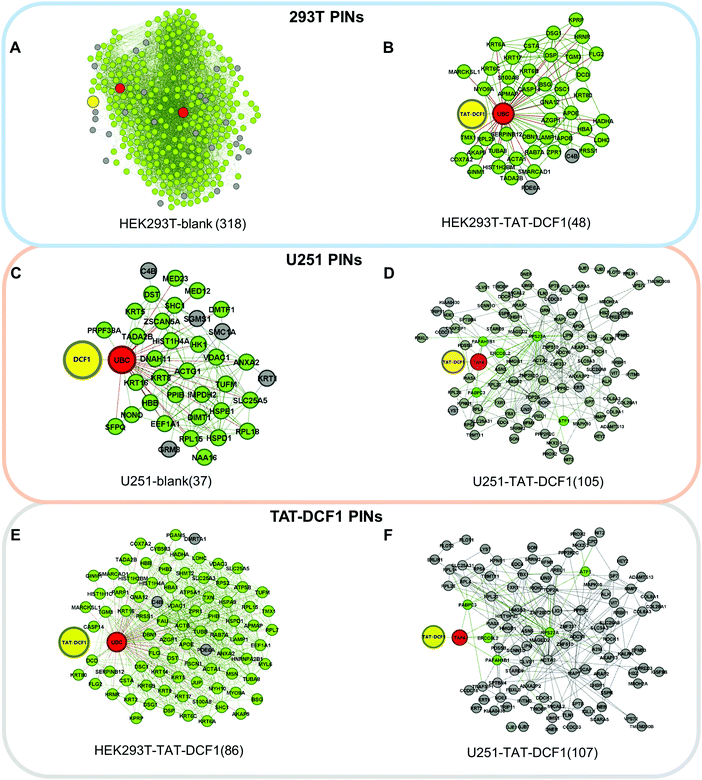 |
| Fig. 2 Protein interaction networks induced by the TAT–DCF1 peptide in U251 and HEK293T cells revealed that TAT–DCF1 interferes with protein interaction in HEK293T and U251 cells. Networks were constructed through pairwise comparison between HEK293T-blank (318) (A) and HEK293T-TAT–DCF1 (48) (B), U251-blank (37) (C) and U251-TAT–DCF1 (105) (D), and HEK293T-TAT–DCF1 (86) (E) and U251-TAT–DCF1 (107) (F). The red nodes denote the genes that directly interact with the yellow nodes, and the green nodes denote the genes that directly interact with the red nodes. | |
Similarly, the proteins that directly interacted with DCF1 were different (UBC in HEK293T-TAT–DCF1 (86) and TAF6 in U251-TAT–DCF1 (107)) (Fig. 2E and F). Furthermore, to evaluate the difference between the PINs, we aligned the networks by HGA13 to calculate the similarity of these networks. The lower the values of EC and PES (Point and Edge Score), the greater the difference between two networks. As seen from Fig. S2 (ESI†), the largest value in the first column (PE: 614.694 and EC: 0.665) indicates that the HEK293T-blank (318) and HEK293T-TAT–DCF1 (48) networks were the most similar, indicating that there was a slight difference between these two networks upon the addition of TAT–DCF1 or blank peptides. In addition, a decrease in the PE (53.122 and 45.069) and EC (0.329 and 0.184) values indicates that there was a large difference between these networks, including U251-blank (37) and U251-TAT–DCF1 (105), and HEK293T-TAT–DCF1 (86) and U251-TAT–DCF1 (107). In conclusion, it is clear that there were many differences between HEK293T and U251 cells upon the addition of TAT–DCF1, suggesting that TAT–DCF1 indeed interferes with protein interaction in HEK293T and U251 cells.
3.4 TAT–DCF1 interacts with TAF6 in U251 cells
TAF6 was a key candidate protein for interaction with TAT–DCF1 in U251 cells (Fig. 2D). To elucidate the interaction between TAT–DCF1 and TAF6, co-immunoprecipitation studies were carried out in U251 cells, and TAT–DCF1 complexes were purified. Elution buffer or 2% SDS buffer (as 100% control) was used to elute TAT–DCF1 complexes from a column containing an antibody against the His-tag on TAT–DCF1. The eluted proteins, including TAT–DCF1 and TAF6, were identified using Western blotting, and the normalized intensity was compared with the 2% SDS elution control. The 2% SDS buffer eluted most of the TAT–DCF1 and TAF6 (Fig. 3A). A mouse anti-(His)6 antibody intensely labeled TAT–DCF1, which copurified with TAF6 (Fig. 3A), indicating that TAT–DCF1 interacts with TAF6. To further verify the interaction between TAT–DCF1 and TAF6 and to ascertain their localization, immunohistochemical analysis was performed in U251 cells (Fig. 3B). As shown in Fig. 3B, colocalization of TAT–DCF1 and TAF6 was observed. The cells were treated with TAT–DCF1 and dual-stained for TAT–DCF1 (with a mouse anti-(His)6 antibody) (green) and TAF6 (with a rabbit anti-TAF6 antibody) (red), and the nuclei were labeled with DAPI (blue). Taken together, the above findings demonstrate that TAT–DCF1 indeed interacted with TAF6.
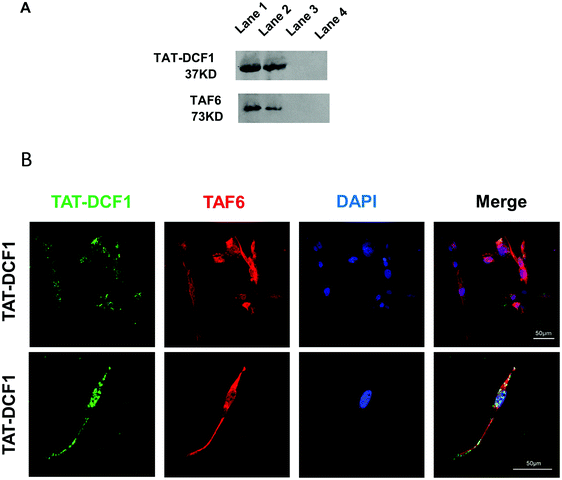 |
| Fig. 3 TAT–DCF1 interacts with TAF6 in U251 cells. U251 cells were incubated with TAT–EGFP or TAT–DCF1 at a concentration of 175 mg L−1 for 12 h. (A) Interaction between TAT–DCF1 and TAF6 was examined by immunoprecipitation and Western blotting. TAT–DCF1 was extracted with an anti-His antibody and detected using DCF1 and TAF6 antibodies. The lines in each panel are in the following order: lane 1, the input group is total membrane protein lysate; lane 2, the elution group is the eluted sample; lane 3, the unbound group is the beads without DCF1 antibody; lane 4, the blank group is the beads with the DCF1 IgG isotype. (B) U251 cells treated with the TAT–DCF1 peptide, with TAF6 displaying colocalization with TAT–DCF1. The TAT–DCF1 peptide was detected by a His-tag marker (green), TAF6 was detected by an anti-TAF6 antibody (red), and the nucleus was counterstained with DAPI (blue). Scale bars: 50 μm. | |
3.5 TAT–DCF1 results in the activation of the RPS27A/TOP2A/HMGB2/BCL-2 signaling pathway via interaction with TAF6
Since TAT–DCF1 interacted with TAF6 (Fig. 3), promoting apoptosis of U251 cells,15 the underlying mechanism remains unclear. To investigate the mechanism by which the TAT–DCF1 and TAF6 interaction initiates an antitumor effect, TRAF6, RPS27A, TOP2A, HMGB1, and HMGB2 (Fig. 4) were selected for expression analysis at the mRNA level, since these proteins were most likely related to cancer.16,23–28 Interestingly, as shown in Fig. 4A, the mRNA levels of TAF6, TOP2A, RPS27A, HMGB1, and HMGB2 in the U251-TAT–DCF1 group were all decreased, whereas the level of TRAF6 was increased, as compared with the U251-control group. Among these genes, TOP2A, RPS27A, and HMGB2 were significantly reduced, but no significant difference in the levels of TAF6, HMGB1, or TRAF6 was observed. In addition, apoptosis-related genes (BCL-2 and BAX) were evaluated by Western blotting following treatment with TAT–DCF1 or TAT-EGFP. As shown in Fig. 4B and C, treatment of U251 cells with TAT–DCF1 dramatically reduced the protein expression of BCL-2 and increased that of BAX, as compared with the U251-Control group. These results suggest that TAT–DCF1 led to the activation of the TOP2A/RPS27A/HMGB2/BCL-2 signaling pathway via interaction with TAF6 revealing the specific functions of DCF1 in U251 cells.
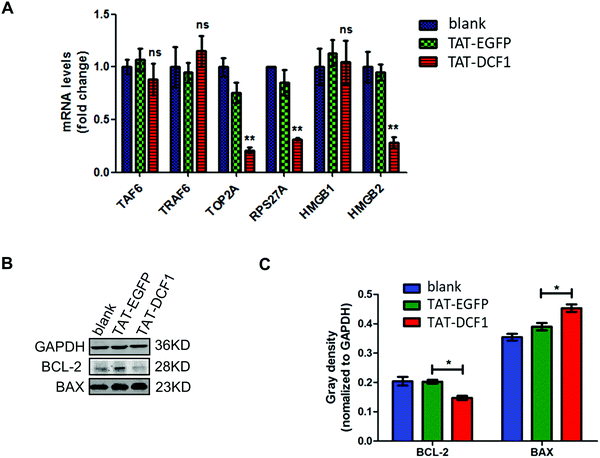 |
| Fig. 4 TAT–DCF1 results in the activation of the TOP2A/RPS27A/HMGB2/BCL-2 signaling pathway via interaction with TAF6 revealing the specific functions of DCF1 in U251 cells. (A) mRNA expression of TAF6, TRAF6, TOP2A, RPS27A, HMGB1 and HMGB2 was assessed by qPCR. An abundance of each mRNA transcript is expressed relative to that of GAPDH, which serves as the internal control. Data are expressed as the mean ± SEM. n = 3. **p < 0.01 vs. TAT–EGFP. (B) The expression of BCL-2 or BAX was analyzed by Western blotting. (C) Statistical analysis of the gray density for the Western blotting of the three groups. GAPDH was used as a loading control. Data are expressed as the mean ± SEM. *p < 0.05. | |
4. Discussion
In the present paper, we show that U251 cells were efficiently transduced with the TAT–DCF1 peptide (Fig. 1A–D). Moreover, IP-Mass was used to detect the proteins bound to TAT–DCF1, and gene ontology and KEGG pathway enrichment analysis show that TAT–DCF1 influences multiple biological processes (Fig. 1E–H and Tables S3–S6, ESI†) including neurodegenerative disease- and ribosome- and HTLV-I infection-related pathways. The process of apoptosis induced by TAT–DCF1 may be under the regulation of multiple proteins and pathways (Fig. 1) in U251 cells. In addition, the established protein interaction networks (Fig. 2) and further biological experiments (Fig. 3) show that TAT–DCF1 directly interacted with TAF6 and plays an important antitumor role via the RPS27A/TOP2A/HMGB2/BCL-2 signaling pathway (Fig. 4).
In order to explore further, the proteins bound to TAT–DCF1 were detected by IP-Mass and the results were analyzed using the DAVID functional annotation tool.29 TAT–DCF1 influenced multiple biological processes in U251 cells (Tables S3–S6, ESI†). Furthermore, certain proteins interacted with DCF1 specifically in U251 cells following treatment with the TAT–DCF1 peptide, and these included HMGB1, HMGB2, and TOP2A, which are associated with DNA topological changes (Table S6, ESI†). HMG (high mobility group) proteins, which are named according to their electrophoretic mobility in polyacrylamide gels, include three superfamilies: HMGB, HMGN, and HMGA.30,31 As shown in Fig. 4A, HMGB2 was significantly downregulated in the U251-TAT–DCF1 group, which is consistent with the previous report that downregulation of HMGB2 promotes apoptosis and inhibits proliferation and invasion of hepatocellular carcinoma cells.32,33 In addition, it has also been reported that overexpression of HMGB2 is associated with tumor aggressiveness and poor prognosis in hepatocellular carcinoma,34 implying that HMGB2 regulated by TAT–DCF1 plays an important role in tumor apoptosis.
To explore the potential signaling pathways in which DCF1 is involved in HEK293T and U251 cells, KEGG pathway enrichment analysis was carried out to elucidate TAT–DCF1-interacting proteins, revealing vital roles of DCF1 in neurodegenerative disease-related pathways (Fig. 1E–H). It has been reported that DCF1 can affect the shedding of amyloid precursor protein (APP) by reducing its access to cellular compartments.14 Moreover, microarray experiments have shown that DCF1 is an important contributing factor to AD.35 Further, DCF1 deficiency leads to dendritic spine dysplasia, which results in the facilitation of memory acquisition.36 These data indicate that DCF1 has an essential role in neurodegenerative disease-related pathways, which is consistent with our study. Most of the proteins that interacted with DCF1 were enriched in ribosome-related pathways in HEK293T cells (Fig. 1E and F). As it is known, ribosomes serve as a complex molecular machinery in protein synthesis,37 and dysregulation of ribosome biogenesis is associated with breast cancer progression,38 indicating that DCF1 is involved in biological processes (including tumor progression) through ribosome-related pathways in U251 and HEK293T cells. As compared with ribosome-related pathways, which were enriched in all four groups (HEK293T-control, HEK293t-TAT–DCF1, U251-control, and U251-TAT–DCF1), HTLV-1 infection-related pathways were specifically enriched in the U251-TAT–DCF1 group (Fig. 1H), indicating that TAT–DCF1 exerts a unique function in U251 cells. Moreover, TLN1, SLC25A31, ADCY8, VDAC2 and ATF1 were enriched in HTLV-I infection-related signaling pathways. It has been shown that TLN1 is an antiparallel actin-binding homodimer39 and functions as a target of the tumor suppressor in ovarian serous carcinoma.40 In addition, TLN1 has also been pursued as a novel therapeutic target in glioma.41 Furthermore, it has been reported that ADCY8 affects glioma risk in a gender-specific fashion.42 In conclusion, the proteins enriched in HTLV-I infection-related signaling pathways may be potential targets for GBM therapy, indicating that the potential mechanisms underlying GBM therapy through TAT–DCF1 may be mediated by the proteins enriched in HTLV-I infection-related pathways in U251 cells.
From the interaction network, we can see that TAT–DCF1 interacted with TAF6 (Fig. 2) in the U251-TAT–DCF1 group. Studies have shown that TAF6 is a component of the TFIID complex involved in RNA polymerase preinitiation and acts in vitro as an essential coactivator of transcription for the tumor suppressor protein P53,43 implying that TAT–DCF1 may act on P53 via TAF6, and consequently participate in preventing tumor progression. Moreover, we detected the expression of RPS27A and TOP2A (Fig. 4A), which are also enriched in DNA topology. RPS27A is one of two (the other is L40) ribosomal proteins that is naturally synthesized as a ubiquitin C-terminal extension protein,44 which is overexpressed in breast cancer,24 human renal cancer cells,45 and colorectal carcinoma.25 Downregulation of RPS27A has been shown to inhibit the proliferation of K562 and K562/G01 cells via downregulation of pERK in the MAPK signaling pathway.46 Here, we show that the expression of RPS27A was downregulated following treatment with TAT–DCF1, which is consistent with these other reports. Furthermore, TOP2A controls and alters the topological states of DNA during transcription and is thus involved in processes such as chromosome condensation and chromatid separation.26 Overexpression and prognostic relevance of TOP2A have been reported for different solid tumors including adrenocortical carcinoma,26 breast cancer,47 prostate cancer,48 renal cell carcinoma,49 and colorectal cancer.50 In the present study, TOP2A was significantly downregulated, suggesting an important role in glioma. DCF1 may induce changes in DNA topology to inhibit tumorigenesis through interactions with RPS27A and TOP2A. It is well known that apoptosis occurs through two main pathways, extrinsic and intrinsic apoptosis signaling, with intrinsic apoptosis being regulated by the anti-apoptotic BCL-2 and pro-apoptotic BAX protein families.51 Here, we show that BCL-2 was downregulated upon the addition of TAT–DCF1, whereas BAX was upregulated, implying that TAT–DCF1 transduction may exert an inhibitory function through the BCL2 and BAX signaling pathway. It is also possible that DCF1 may influence the classical tumor inhibition pathway to inhibit glioma proliferation.
5. Conclusions
In conclusion, functional enrichment analyses indicate that TAT–DCF1 induced important biological changes in U251 cells. The differential protein interaction networks of TAT–DCF1 and biological experiments revealed that the antitumor effect of TAT–DCF1 was at least in part mediated by the TOP2A/RPS27A/HMGB2 and BCL-2 signaling pathways. Our study suggests that the TAT–DCF1 peptide possesses great potential for the development of glioblastoma therapy through the interaction with TAF6-related pathways.
Funding
This work was supported by National Natural Science Foundation of China (31500827, 81471162, and 61873156), Natural Science Foundation of Shanghai (17ZR1409900), Science and Technology Commission of Shanghai Municipality (14JC1402400), The Programme for Changjiang Scholars and Innovative Research Team in University of Ministry of Education, China (IRT_17R88).
Conflicts of interest
There are no conflicts of interest to declare.
Acknowledgements
We would like to thank Dr Natalie Ward (Medical College of Wisconsin, Wauwatosa, WI, USA) for editing this manuscript.
References
- D. R. Johnson and B. P. O'Neill, Glioblastoma survival in the United States before and during the temozolomide era, J. Neurooncol., 2012, 107(2), 359–364 CrossRef CAS PubMed.
- I. Paw,
et al., Mechanisms regulating glioma invasion, Cancer Lett., 2015, 362(1), 1–7 CrossRef CAS PubMed.
- W. F. Anderson, Human gene therapy, Nature, 1998, 392(6679 suppl), 25–30 CAS.
- L. Wang,
et al., A novel function of dcf1 during the differentiation of neural stem cells in vitro, Cell. Mol. Neurobiol., 2008, 28(6), 887–894 CrossRef PubMed.
- T. Wen, P. Gu and F. Chen, Discovery of two novel functional genes from differentiation of neural stem cells in the striatum of the fetal rat, Neurosci. Lett., 2002, 329(1), 101–105 CrossRef CAS PubMed.
- Q. Liu,
et al., Dcf1 Triggers Dendritic Spine Formation and Facilitates Memory Acquisition, Mol. Neurobiol., 2018, 55(1), 763–775 CrossRef CAS PubMed.
- Y. Chen,
et al., DCF1 subcellular localization and its function in mitochondria, Biochimie, 2018, 144, 50–55 CrossRef CAS PubMed.
- J. Wang,
et al., The antitumor effect of TAT-DCF1 peptide in glioma cells, Neuropeptides, 2018, 71, 21–31 CrossRef CAS PubMed.
- D. Szklarczyk,
et al., STRINGv10: protein-protein interaction networks, integrated over the tree of life, Nucleic Acids Res., 2015, 43(Database issue), D447–D452 CrossRef CAS PubMed.
- T. Milenkovic and N. Przulj, Uncovering biological network function via graphlet degree signatures, Cancer Inf., 2008, 6, 257–273 Search PubMed.
- N. Przulj, D. G. Corneil and I. Jurisica, Modeling interactome: scale-free or geometric?, Bioinformatics, 2004, 20(18), 3508–3515 CrossRef CAS PubMed.
- C. UniProt, UniProt: a hub for protein information, Nucleic Acids Res., 2015, 43(Database issue), D204–D212 Search PubMed.
- J. Xie,
et al., An Adaptive Hybrid Algorithm for Global Network Alignment, IEEE/ACM Trans. Comput. Biol. Bioinform., 2016, 13(3), 483–493 CAS.
- S. Ullrich,
et al., The novel membrane protein TMEM59 modulates complex glycosylation, cell surface expression, and secretion of the amyloid precursor protein, J. Biol. Chem., 2010, 285(27), 20664–20674 CrossRef CAS PubMed.
- Y. Xie,
et al., Overexpression of DCF1 inhibits glioma through destruction of mitochondria and activation of apoptosis pathway, Sci. Rep., 2014, 4, 3702 CrossRef PubMed.
- S. Zanotti, A. Fisseler-Eckhoff and H. G. Mannherz, Changes in the topological expression of markers of differentiation and apoptosis in defined stages of human cervical dysplasia and carcinoma, Gynecol. Oncol., 2003, 89(3), 376–384 CrossRef CAS PubMed.
- B. Yan,
et al., Apoptotic DNA fragmentation factor maintains chromosome stability in a P53-independent manner, Oncogene, 2006, 25(39), 5370–5376 CrossRef CAS PubMed.
- T. Dohi,
et al., Mitochondrial survivin inhibits apoptosis and promotes tumorigenesis, J. Clin. Invest., 2004, 114(8), 1117–1127 CrossRef CAS PubMed.
- M. T. Lai,
et al., Talin-1 overexpression defines high risk for aggressive oral squamous cell carcinoma and promotes cancer metastasis, J. Pathol., 2011, 224(3), 367–376 CrossRef CAS PubMed.
- B. Clemencon, M. Babot and V. Trezeguet, The mitochondrial ADP/ATP carrier (SLC25 family): pathological implications of its dysfunction, Mol. Aspects Med., 2013, 34(2–3), 485–493 CrossRef CAS PubMed.
- C. Gallerne,
et al., The fourth isoform of the adenine nucleotide translocator inhibits mitochondrial apoptosis in cancer cells, Int. J. Biochem. Cell Biol., 2010, 42(5), 623–629 CrossRef CAS PubMed.
- Y. Yoshida,
et al., A comprehensive method for detecting ubiquitinated substrates using TR-TUBE, Proc. Natl. Acad. Sci. U. S. A., 2015, 112(15), 4630–4635 CrossRef CAS PubMed.
- H. Sun,
et al., TRAF6 is upregulated in colon cancer and promotes proliferation of colon cancer cells, Int. J. Biochem. Cell Biol., 2014, 53, 195–201 CrossRef CAS PubMed.
- S. M. Adams,
et al., Differential expression of translation-associated genes in benign and malignant human breast tumours, Br. J. Cancer, 1992, 65(1), 65–71 CrossRef CAS PubMed.
- J. M. Wong,
et al., Ubiquitin-ribosomal protein S27a gene overexpressed in human colorectal carcinoma is an early growth response gene, Cancer Res., 1993, 53(8), 1916–1920 CAS.
- M. Jain,
et al., TOP2A is overexpressed and is a therapeutic target for adrenocortical carcinoma, Endocr.-Relat. Cancer, 2013, 20(3), 361–370 CAS.
- M. T. Lotze and R. A. DeMarco, Dealing with death: HMGB1 as a novel target for cancer therapy, Curr. Opin. Invest. Drugs, 2003, 4(12), 1405–1409 CAS.
- M. Stros,
et al., HMGB1 and HMGB2 proteins up-regulate cellular expression of human topoisomerase IIalpha, Nucleic Acids Res., 2009, 37(7), 2070–2086 CrossRef CAS PubMed.
- W. da Huang, B. T. Sherman and R. A. Lempicki, Systematic and integrative analysis of large gene lists using DAVID bioinformatics resources, Nat. Protoc., 2009, 4(1), 44–57 CrossRef PubMed.
- M. Bustin, Revised nomenclature for high mobility group (HMG) chromosomal proteins, Trends Biochem. Sci., 2001, 26(3), 152–153 CrossRef CAS PubMed.
- G. H. Goodwin, C. Sanders and E. W. Johns, A new group of chromatin-associated proteins with a high content of acidic and basic amino acids, Eur. J. Biochem., 1973, 38(1), 14–19 CrossRef CAS PubMed.
- Y. Liu, H. Liang and X. Jiang, MiR-1297 promotes apoptosis and inhibits the proliferation and invasion of hepatocellular carcinoma cells by targeting HMGA2, Int. J. Mol. Med., 2015, 36(5), 1345–1352 CrossRef CAS PubMed.
- J. Li,
et al., Long non-coding RNA MALAT1 drives gastric cancer progression by regulating HMGB2 modulating the miR-1297, Cancer Cell Int., 2017, 17, 44 CrossRef PubMed.
- J. H. Kwon,
et al., Overexpression of high-mobility group box 2 is associated with tumor aggressiveness and prognosis of hepatocellular carcinoma, Clin. Cancer Res., 2010, 16(22), 5511–5521 CrossRef CAS PubMed.
- L. Zhang,
et al., Identifying Tmem59 related gene regulatory network of mouse neural stem cell from a compendium of expression profiles, BMC Syst. Biol., 2011, 5, 152 CrossRef CAS PubMed.
- Q. Liu,
et al., Dcf1 Triggers Dendritic Spine Formation and Facilitates Memory Acquisition, Mol. Neurobiol., 2018, 55(1), 763–775 CrossRef CAS PubMed.
- R. Green and H. F. Noller, Ribosomes and translation, Annu. Rev. Biochem., 1997, 66, 679–716 CrossRef CAS PubMed.
- S. Belin,
et al., Dysregulation of ribosome biogenesis and translational capacity is associated with tumor progression of human breast cancer cells, PLoS One, 2009, 4(9), e7147 CrossRef PubMed.
- M. Muto,
et al., Novel serum autoantibodies against talin1 in multiple sclerosis: Possible pathogenetic roles of the antibodies, J. Neuroimmunol., 2015, 284, 30–36 CrossRef CAS PubMed.
- H. Tang,
et al., miR-9 functions as a tumor suppressor in ovarian serous carcinoma by targeting TLN1, Int. J. Mol. Med., 2013, 32(2), 381–388 CrossRef CAS PubMed.
- A. M. Saratsis,
et al., Comparative multidimensional molecular analyses of pediatric diffuse intrinsic pontine glioma reveals distinct molecular subtypes, Acta Neuropathol., 2014, 127(6), 881–895 CrossRef CAS PubMed.
- N. M. Warrington, T. Sun and J. B. Rubin, Targeting brain tumor cAMP: the case for sex-specific therapeutics, Front. Pharmacol., 2015, 6, 153 Search PubMed.
- C. J. Thut,
et al., p53 transcriptional activation mediated by coactivators TAFII40 and TAFII60, Science, 1995, 267(5194), 100–104 CrossRef CAS PubMed.
- K. L. Redman and M. Rechsteiner, Identification of the long ubiquitin extension as ribosomal protein S27a, Nature, 1989, 338(6214), 438–440 CrossRef CAS PubMed.
- H. Kanayama,
et al., Changes in expressions of proteasome and ubiquitin genes in human renal cancer cells, Cancer Res., 1991, 51(24), 6677–6685 CAS.
- H. Wang,
et al., RPS27a promotes proliferation, regulates cell cycle progression and inhibits apoptosis of leukemia cells, Biochem. Biophys. Res. Commun., 2014, 446(4), 1204–1210 CrossRef CAS PubMed.
- B. Szekely,
et al., A 3-gene proliferation score (TOP-FOX-67) can re-classify histological grade-2, ER-positive breast cancers into low- and high-risk prognostic categories, Breast Cancer Res. Treat., 2013, 138(3), 691–698 CrossRef CAS PubMed.
- M. F. de Resende,
et al., Prognostication of prostate cancer based on TOP2A protein and gene assessment: TOP2A in prostate cancer, J. Transl. Med., 2013, 11, 36 CrossRef PubMed.
- D. Chen,
et al., TOP2A, HELLS, ATAD2, and TET3 Are Novel Prognostic Markers in Renal Cell Carcinoma, Urology, 2017, 102, 265 e1–265 e7 CrossRef PubMed.
- A. Coss,
et al., Increased topoisomerase IIalpha expression in colorectal cancer is associated with advanced disease and chemotherapeutic resistance via inhibition of apoptosis, Cancer Lett., 2009, 276(2), 228–238 CrossRef CAS PubMed.
- B. Deng,
et al., Targeted delivery of neurogenin-2 protein in the treatment for cerebral ischemia-reperfusion injury, Biomaterials, 2013, 34(34), 8786–8797 CrossRef CAS PubMed.
Footnote |
† Electronic supplementary information (ESI) available. See DOI: 10.1039/c9mo00068b |
|
This journal is © The Royal Society of Chemistry 2020 |