DOI:
10.1039/C9MD00451C
(Research Article)
RSC Med. Chem., 2020,
11, 142-147
Conversion of amino acids to aryl/heteroaryl ethanol metabolites using human CYP2D6-expressing live baker's yeast†
Received
20th September 2019
, Accepted 18th November 2019
First published on 23rd December 2019
Abstract
Different natural aromatic/heterocyclic L-amino acids were biotransformed into aryl/heteroaryl ethanol metabolites via oxidative deamination, decarboxylation and reduction cascades using live baker's yeast cells producing intracellular human CYP2D6 enzyme. Among the three yeast strains expressing 3 different CYP2D6 variants, CYP2D6(2) (i.e. CYP2D6 wild-type) provided the best result under neutral pH conditions at RT. We have successfully converted six natural amino acids into their corresponding alcohols, having one carbon atom less, with moderate yields. Some of the downstream products like tryptophol and tyrosol induced the pTrKB (Tropomyosin receptor kinase B) activation pattern similar to that of BDNF (brain-derived neurotrophic factor), thereby depicting potential antidepressant activity. Control experiments and molecular modelling studies revealed that this tandem bio-transformation probably happens via a pyruvate intermediate. This study establishes that CYP2D6-expressing live yeast cells can be a powerful tool for the enzymatic C–N, C–C bond cleavage of amino-acids.
Amino acids are among the vital building blocks of all living organisms and act as a versatile tool for probing biological systems. Amino acids constitute a highly attractive class of molecules for the synthesis of complex organic compounds. Given their importance, many synthetic and biocatalytic methods have been developed where they have been used as substrates for the synthesis of various pharmaceutically active compounds.1 The reactive free amino and carboxylic groups in amino acids provide ample inspiration for the effective design of C–N, C–C bond cleavage methods, and also pose interesting challenges in the field of organic chemistry. There are a few discrete reports on the deamination and decarboxylation of amino acids using toxic metal reagents.2 The hurdle in dealing with the above mentioned transformations is the insolubility of amino acids in organic solvents, which require additional protection in order to do further modification. There is only one method reported so far, using a cocktail of reagents at high temperature, where deamination and decarboxylation of amino acids followed by C–C bond formation took place in one pot3 (Scheme 1; upper panel). It would be remarkable if these complex reaction conditions could be swapped for milder reaction conditions, mediated by an enzyme (Scheme 1; lower panel). In this regard, tandem biocatalysis has received increasing attention for the synthesis of compounds of pharmaceutical importance. It has several distinctive features as compared to chemical catalysis due to its non-toxic nature, high chemoselectivity and regioselectivity. Although cascade biocatalysis happens in the metabolic pathways in cells, it is desirable to develop non-natural cascade biocatalysis by using recombinant enzymes. Over the years, several types of cascade biocatalysis have been reported. Nevertheless, it is still very important to develop a new type of biocatalysis for practical synthesis;4 however, the appropriate choice of enzyme for biotransformation is of paramount importance. It must be based on a prospective substrate's ability to interact strongly with an enzyme's active site. CYP enzymes are a family of haemoproteins that have been reported to catalyse varied biotransformation reactions that include hydroxylation, epoxidation, N- or O-dealkylation, and Baeyer–Villiger oxidation.5 The catalytic abilities of CYPs, expressed in bacteria, have been exploited to modify natural products.6 Diverse bacterial CYP isoforms have been secreted into supernatants of bacterial cell cultures for use in a wide variety of biotransformations.7 Being prokaryotes, bacteria do not have the machinery to express the major drug-metabolising human CYP proteins, which are naturally bound to endoplasmic reticular (ER) membranes to form correctly folded, intracellular enzymes. However, the intracellular architecture (including ER) of baker's yeast (Saccharomyces cerevisiae), a simple eukaryote, is very similar to that of human cells.8–10 Hence, we established yeast strains where CYP gene expression cassettes were stably integrated into chromosomal loci with the aim of preventing the loss of the heterologous genetic information during the growth of recombinant yeast in the absence of any selection, in complex YPD (Yeast, Peptone, Dextrose)growth medium.8,9,11 When the enzyme activity of the intracellular CYP2D6(2) enzyme expressed in yeast cells containing 1 or 2 chromosomally integrated copies of the CYP2D6(2) gene was compared with the cell harbouring a CYP2D6(2) gene encoding multi-copy 2 μ plasmid (Fig. 1), it was observed that the yeast cells expressing CYPs from an extra-chromosomal multicopy 2 μ-plasmid underwent severe plasmid loss when grown for 72 h in complete YPD medium, which is non-selective. In contrast, chromosomally integrated 2 and 1-copy yeast strains stably expressed the CYP enzyme (bars 1 and 2, Fig. 1). It has been reported that the CYP isozyme, CYP2D6, with its hydrogen-bond donor and acceptor abilities, has the potential to target nitrogen atoms.12 CYP2D6 also has a well-defined active site cavity of around 6–8 Å, containing amino acid residues that could be important for embarking on novel chemical strategies.9,13 Molecular modelling studies have shown that the amino acids Arg and Pro have the propensity to bind nitrogen atoms leading to their deamination,14 whereas the amino acid Glu has a strong binding affinity for carbonyl oxygen, facilitating its decarboxylation.15 With this in mind, we thought that intrinsically, amino acids could be effective substrates for the CYP2D6 enzyme active site, which has Arg/Pro/Glu residues participating in CYP2D6-mediated metabolisms.12 Hence, we were curious to know the consequence of CYP2D6’s action, expressed within live yeast cells, on natural amino acids.
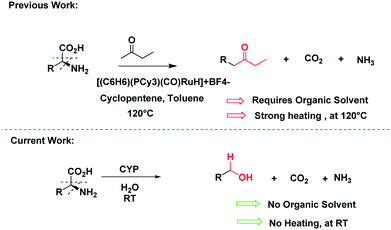 |
| Scheme 1 One-pot deamination and decarboxylation of amino acids. | |
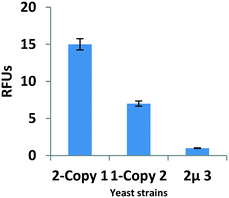 |
| Fig. 1 Human CYP2D6(2) enzyme activity in yeast cells containing 1 or 2 chromosomally integrated copies of the CYP2D6(2) gene, as compared with the cell harbouring a CYP2D6(2) gene encoding the multi-copy 2 μ plasmid, grown for 72 h in a fed-batch. | |
We began our experimental study by growing 2-copy CYP2D6(2)-expressing yeast cells9,16 (Fig. 1) in YPD complex medium, for high biomass production and concomitant high specific enzyme activity (see ESI,† section 8), before adding an amino acid substrate to cells that would be grown in selective, minimal SD (synthetic defined) medium. The medium was changed to minimal SD to avoid any spurious interactions of the substrate with the nutritious components of YPD. Then, 3.0 mL of cells (OD600, ∼80) from a 72 h YPD culture (at a time point when the intracellular enzyme was amply expressed), were inoculated in 200 mL of minimal SD medium.8,9 For the very first amino acid-based biotransformation, 10 mg of tryptophan (Trp), dissolved in DMSO, was added to the SD culture, which was incubated at 30 °C for 48 h with shaking at 200 rpm. The enzyme produced by the yeast CYP 2D6 is membrane-bound. During the reaction, the enzyme interacted with tryptophan and converted it into tryptophol. After the reaction, the broth was centrifuged, and the biomass obtained was discarded and the cell-free broth containing tryptophol was later extracted with ethyl acetate and purified by flash chromatography on a silica column, which afforded a brownish solid compound. The spectroscopic data surprisingly showed that the product formed was tryptophol (Schemes 2 and 1a). We, therefore, inferred that Trp must have undergone a highly selective multistep one-pot transformation involving oxidative deamination and decarboxylation followed by reduction, resulting in the synthesis of tryptophol. This CYP2D6-mediated transformation directly achieved C–N and C–C bond cleavage in a single pot, forming the alcohol from the amino acid, without using any toxic reagents or harsh conditions. Tryptophol induces sleep in humans and has been suggested to be a functional analogue of serotonin and melatonin.17 With the aim of improving tryptophol yields, we set out to compare the ability of yeast cells, individually expressing 2-copies of three different CYP2D6 variants (1, 2 and C) (ESI,† Sections 2–7),9,10,18 to convert Trp to tryptophol. Although all 3 yeast strains converted Trp to tryptophol, the variant 2D6(2) (2D6-wt) provided the best yields (∼45%) (Fig. 2A).
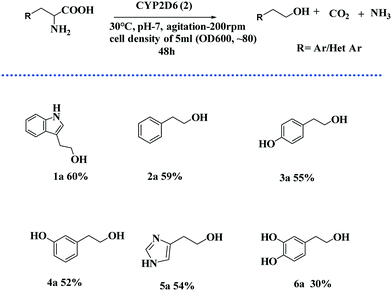 |
| Scheme 2 Products obtained from CYP2D6(2) reactions on various amino acids, under optimal reaction conditions. | |
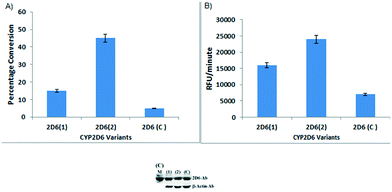 |
| Fig. 2 (A) Percentage conversion of tryptophan to tryptophol. (B) Comparison of CYP2D6 activities of the three CYP2D6 variants, as measured using 7-ethoxymethoxy-3-cyanocoumarin (EOMCC) as the substrate. (C) Western blots of 10 μg of total cellular proteins obtained, after lysis, from cells bearing different 2D6 variants. Lane 1: 2D6(1); lane 2: 2D6(2); lane 3: 2D6(C); M: 1 pmole of CYP2D6(2) Sacchrosomes (microsomal, ER-bound enzyme isolated from CYP2D6(2)-harbouring recombinant yeast cells). | |
In parallel, differences in the specific activity of the CYP2D6 variants in the conversion of 7-ethoxymethoxy-3-cyanocoumarin (EOMCC) to the fluorescent product 7-hydroxy-3-cyanocoumarin were assessed (Fig. 2B). The results mirrored the differences in the metabolism of the 3 variants of Trp. Within cells, harbouring the 3 variants, the CYP2D6 protein was produced at similar levels (Fig. 2C) indicating that irrespective of the substrate, Trp or EOMCC, the activities of the variants differed greatly.
The enzyme activity of a picomole of CYP2D6(2) microsomal enzyme (i.e. Sacchrosomes) was compared individually with a picomole of commercially available CYP2D6 microsomal enzymes isolated from insect and bacterial cells (Fig. 3). It was observed that CYP2D6(2) Sacchrosomes, obtained from whole yeast cells, were at least twice as active as the other three commercially available enzymes obtained from Corning, Cypex and Invitrogen (Thermo Fisher). The experiment was performed for reassurance that besides it being highly advantageous to use live whole yeast cells that can continuously produce an active human CYP enzyme over 2–3 days, there could be an added benefit if the intracellular enzyme activity was high. Both properties could then simultaneously support the productivity of deamination and decarboxylation of amino acids.
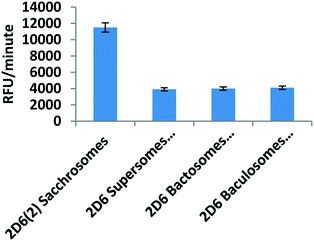 |
| Fig. 3 Comparison of the activities of 1 pmole of CYP2D6(2) microsomal (ER-bound) enzyme (Sacchrosomes), isolated from baker's yeast, with a pmole of microsomal enzyme obtained from insect cells [Baculosomes (Invitrogen); Supersomes (Corning)], and bacterial cells [Bactosomes (Cypex)], using 4 μM EOMCC as a substrate. | |
In order to determine the optimal conditions for achieving the maximal conversion of tryptophan to tryptophol, using the CYP2D6(2) wild-type variant, different physical parameters were evaluated (Fig. 4). In this context, temperature (20–60 °C), pH (5.0–9.0), agitation rate (0–300 rpm), cell concentration (2.0, 3.0 5.0, 8.0, and 10.0 ml of OD600, ∼80) and incubation period (12–60 h) were studied. On increasing the substrate concentration to 200 mg, up to 60% conversion was obtained; however, a further increase in substrate concentration slightly decreased the production yield in 200 mL SD culture (Fig. 4D). From these optimisation studies, we clearly observed that 30 °C, pH 7.0, agitation at 200 rpm and inoculums cell density of 5 mL (OD600, ∼80) supported the maximum conversion of tryptophan to tryptophol, of 60%. Since production yields almost peaked at 48 h, the conversion time for transformations performed later was reduced from 60 h to 48 h, making the process more economical.
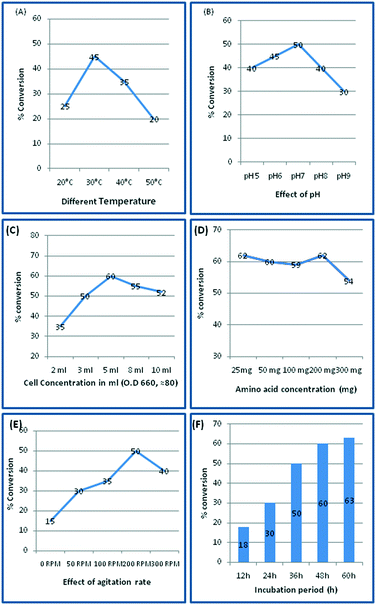 |
| Fig. 4 Plots showing the effects of (A) temperature, (B) pH, (C) cell concentration, (D) amino acid concentration, (E) agitation rate, and (F) incubation period, on the % conversion of the substrate to product, using CYP2D6(2) enzyme expressed in 200 mL of SD medium. | |
We then surveyed the substrate scope of the CYP2D6-mediated amino acid to alcohol transformation (Scheme 2). We found that the one-pot cascade of reactions catalysed by CYP2D6 possesed wider applications. In general, amino acids with aryl and heterocyclic side chains readily react with the CYP2D6 enzyme to form the corresponding alcohols that are of importance both biologically and chemically. Metabolite ethanols are known to possess various neurorelative properties,19 so we wanted to see the neuroprotective activity of the downstream product obtained. Towards this end, we carried out the experiments and we observed that tryptophol and tyrosol were able to protect the neurons from corticosterone-induced toxicity, thereby displaying neuroprotective properties (Fig. 5A and B). Both resulted in the induction of BDNF neurotropic response and induced BDNF-dependent activation of pTrkb along with its downstream signaling cascade (Fig. 6). TRP and TYRO induced the activation of the prosurvival pathway in differentiated human SHSY5Y cells, thereby shutting off neuronal apoptosis. We used DHF (7,8 dihydroxyflavone, 5 nM) as the positive control and CORT (corticosterone-400 μM) as the negative control. Our study depicted DHF as a neuroprotective chemical entity capable of rescuing differentiated neuroblastoma cells from toxicity induced by corticosterone. DHF activates the TrkB receptor by dimerization, thereby activating various survival proteins ahead of increasing the expression of BDNF protein, as shown by ELISA. The effective conc. of 5 nM was indicated after performing multiple line experiments.
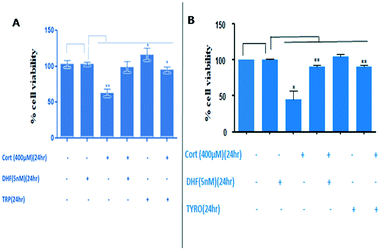 |
| Fig. 5 Neuroprotective activities of TRP, TYRO. (A and B) TRP and TYRO treatment of corticosterone provoked neuronal cell death in differentiated mouse neuroblastoma cell lines (N2a) obtained from ECACC through Sigma-Aldrich. Cells were subjected to differentiation 3 days ahead of treatment, pretreated with (10 μM) TYRO, followed by toxicity studies for 24 h, and then were subjected to quantitative analysis. P-value ≤ 0.05*, <0.01**. | |
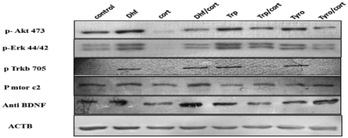 |
| Fig. 6 TRP and TYRO induce PTrkb phosphorylation protecting neuronal cells from apoptosis. HumanSHSY5Y cells obtained from ECACC through Sigma-Aldrich were differentiated for 6 days, followed by compound treatment the next day in a time-dependent manner; lysates were subjected to Western blot analysis for checking protein expression levels. | |
There are two possible pathways that can explain these biosynthetic transformations [pathway (a) or (b); Scheme 3].
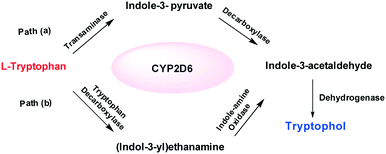 |
| Scheme 3 The two possible pathways predicted for tryptophol synthesis. The reaction mechanism follows path (a), undergoing transamination and decarboxylation followed by reduction. | |
For example, Trp to tryptophol biotransformation may take place via the indole-3-pyruvate intermediate (pathway-a), or indole-3-ethanamine (pathway-b). In order to determine which of the two pathways is more plausible, we performed control experiments (Scheme 4) followed by in silico molecular docking studies (Fig. 7).
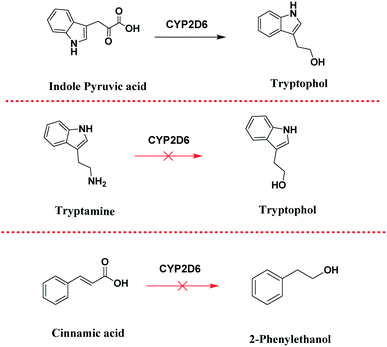 |
| Scheme 4 Control experiments with indole pyruvic acid, tryptamine and cinnamic acid. | |
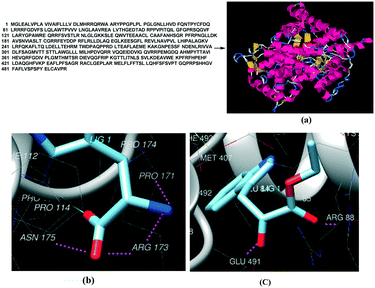 |
| Fig. 7 (a) 3D structure of CYP2D6(2)/CYP2D6-wild type (NCBI Accession #M20403; also referred to as CYP2D6-wild type; [16]) by ab initio methods. (b) Major interactions of protein bound to (b) tryptophan and (c) indole-3-pyruvate. | |
As control experiments, indole pyruvic acid, tryptamine, and cinnamic acid were incubated, with shaking, with CYP2D6(2)-expressing yeast cells. It was found that tryptamine and cinnamic acid do not undergo any transformation, whereas the target alcohol, tryptophol, was obtained only from indole pyruvic acid. These experiments strongly suggest that the tandem reactions from the amino acid to the alcohol, mediated by CYP2D6, proceed solely through pathway-a. The 3D structure of CYP2D6(2)/CYP2D6-wild type protein (NCBI Accession #M20403) (ESI,† section 3) was generated using ab initio methods (Fig. 7a).
Tryptophan and the probable intermediate indole-3-pyruvate were docked into the active site of the CYP2D6 protein structure.
Molecular docking of tryptophan showed that the protonated nitrogen of tryptophan formed H-bonds with Arg173 (Scheme 5) and other residues, which may lead to deamination. Indole-3-pyruvate bound to Glu491, Arg88, Ala85 (Scheme 5) may facilitate decarboxylation, which is in agreement with the literature.14,15 The docked images of tryptophan and indole-3-pyruvate are shown in Fig. 7b and c. On the basis of both control experiments and docking studies, one can conclude that the most likely reaction mechanism follows pathway-a.
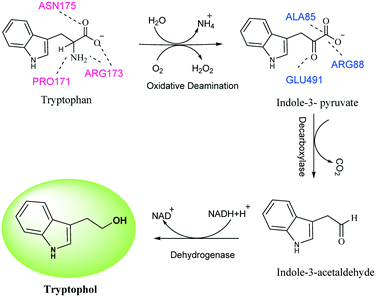 |
| Scheme 5 The proposed mechanism for the formation of tryptophol; the amino acid residues in the CYP2D6(2) enzyme active site, involved in the different steps of the tandem set of reactions, are coloured pink, blue and red. | |
Conclusions
In summary, we have successfully developed CYP2D6-mediated multistep transformations where oxidative deamination and decarboxylation followed by reduction can take place in one pot. This achieves the direct C–C and C–N bond cleavage of amino acid substrates on a preparative scale and does not require any reactive reagents or pre-functionalization of the substrates. Among the ethanol metabolites obtained, tryptophol and tyrosol showed potential antidepressant activities. Both stimulated pTrKB activation patterns, like that of BDNF, thereby depicting the potential neuroprotective activities of these compounds. Furthermore, a versatile biocatalytic method has been established that can be used as a toolbox for C–C and C–N bond cleavage. Our results suggest that human CYP2D6-expressing recombinant baker's yeast cells can become part of a major toolbox for the simultaneous one-pot transformation of amino acids into pharmaceutically active products. The downstream products have shown high antidepressant activities, which will be disclosed in due course. Further studies will be undertaken to take the products to the next level.
Conflicts of interest
There are no conflicts to declare.
Acknowledgements
The authors are thankful to DST-India (EMR-2016-004710), DST-India (EMR/2016/002786), CSIR-IIIM HCP0008 and CYP Design Ltd (UK) for funding. M. B. and S. C. thank CSIR-IIIM, Jammu for providing the facilities.
Notes and references
- C. Najera and J. M. Sansano, Chem. Rev., 2007, 107, 4584 CrossRef CAS PubMed.
-
(a) G. Golime, G. Bogonda, H. Y. Kim and K. Oh, ACS Catal., 2018, 8, 4986 CrossRef CAS;
(b) M. Mure, Acc. Chem. Res., 2004, 37, 131 CrossRef CAS PubMed;
(c) M. D. Kenneth, G. B. Bantchev, E. L. Walter, R. E. Murray, M. Appell, J. C. Lansing and B. R. Moser, Ind. Eng. Chem. Res., 2017, 56, 864 CrossRef;
(d) P. S. Kumar, R. M. Raj, S. K. Rani and D. Easwaramoorthy, Ind. Eng. Chem. Res., 2012, 51, 6310 CrossRef CAS;
(e) D. Liu, J. Sun, B. A. Simmons and S. Singh, ACS Sustainable Chem. Eng., 2018, 6, 7232 CrossRef CAS.
- N. Kalutharage and S. Y. Chae, Angew. Chem., 2013, 125, 13896 CrossRef.
-
(a) M. Breuer, K. Ditrich, T. Habicher, B. Hauer, M. Kesseler, R. Sturmer and T. Zelinski, Angew. Chem., Int. Ed., 2004, 43, 788 CrossRef CAS PubMed;
(b) S. Panke, M. Held and M. Wubbolts, Curr. Opin. Biotechnol., 2004, 15, 272 CrossRef CAS PubMed;
(c) R. N. Patel, ACS Catal., 2011, 1, 1056 CrossRef CAS;
(d) C. M. Clouthier and J. N. Pelletier, Chem. Soc. Rev., 2012, 41, 1585 RSC.
-
(a) A. Chefsonand and K. Auclair, Mol. BioSyst., 2006, 2, 462 RSC;
(b) F. P. Guengerich, Chem. Res. Toxicol., 2001, 14, 611 Search PubMed.
-
(a) A. Greule, J. E. Stok, J. J. De Voss and M. J. Cryle, Nat. Prod. Rep., 2018, 35, 757 RSC;
(b) R. Bernhardt, J. Biotechnol., 2006, 124, 128 CrossRef CAS PubMed;
(c) M. J. Coon, Annu. Rev. Pharmacol., 2005, 45, 1 CrossRef CAS PubMed;
(d) S. G. Sligar, Essays Biochem., 1999, 34, 71 CrossRef CAS PubMed.
-
(a) K. Yasuda, H. Sugimoto, K. Hayashi, T. Takita, K. Yasukawa, M. Ohta, M. Kamakura, S. Ikushiro, Y. Shiro and T. Sakaki, Biochim. Biophys. Acta, Proteins Proteomics, 2018, 23, 1866 Search PubMed;
(b) D. Schmitz, S. Janocha, F. M. Kiss and R. Bernhardt, Biochim. Biophys. Acta, Proteins Proteomics, 2018, 11, 1866 Search PubMed;
(c) P. J. Almhjell, C. E. Boville and F. H. Arnold, Chem. Soc. Rev., 2018, 47, 8980 RSC;
(d) R. K. Zhang, X. Huangand and F. H. Arnold, Curr. Opin. Chem. Biol., 2018, 49, 67 CrossRef PubMed;
(e) O. F. Brandenberg, R. Fasan and F. H. Arnold, Curr. Opin. Biotechnol., 2017, 47, 102 CrossRef CAS PubMed;
(f) H. M. Girvan and A. W. Munro, Curr. Opin. Chem. Biol., 2016, 31, 136 CrossRef CAS PubMed.
- I. S. Williams, S. Chib, V. Nuthakki, S. Bharate, S. Saran and B. Chaudhuri, J. Agric. Food Chem., 2017, 65, 7440 CrossRef CAS PubMed.
- I. S. Williams, L. Gatchie, S. B. Bharate and B. Chaudhuri, ACS Omega, 2018, 3, 8903 CrossRef CAS PubMed.
- H. Yamazaki, Z. Guo, M. Persmark, M. Mimura, F. J. Gonzalez, C. Sugahara, F. P. Guengerich and T. Shimada, Mol. Pharmacol., 1994, 46, 568 CAS.
-
I. S. Williams and B. Chaudhuri, A handbook on high value fermentation products, Wiley-Scrivener, 2019, p. 323, ISBN #9781119460015 Search PubMed.
- G. Cruciani, E. Carosati, B. D. Boeck, T. Howe and R. Vianello, J. Med. Chem., 2005, 48, 6970 CrossRef CAS PubMed.
- A. Wang, C. D. Stout, Q. Zhang and E. F. Johnson, J. Biol. Chem., 2015, 290, 5092 CrossRef CAS PubMed.
- G. S. Hossain, H. Shin, J. Li, J. Chen and L. Liu, RSC Adv., 2016, 6, 82676 RSC.
- M. Lobell and D. H. GCrout, J. Am. Chem. Soc., 1996, 118, 1867 CrossRef CAS.
- S. W. Ellis, K. Rowland, M. J. Ackland, E. Rekka, A. P. Simula, M. S. Lennard, C. R. Wolf and G. T. Tucker, Biochem. J., 1996, 316, 647 CrossRef CAS PubMed.
-
(a) E. M. Cornford, P. D. Crane, L. D. Braun, W. D. Bocash, A. M. Nyerges and W. H. Oldendorf, J. Neurochem., 1981, 36, 1758 CrossRef CAS PubMed;
(b) E. MCornford, W. D. Bocash, L. D. Braun, P. D. Crane, W. H. Oldendorf and A. J. MacInnis, J. Clin. Invest., 1979, 63, 1241 CrossRef PubMed;
(c) S. J. Garden, R. B. Silva and A. C. Pinto, Tetrahedron, 2002, 58, 8399 CrossRef CAS.
- P. Rowland, F. E. Blaney, M. G. Smyth, J. J. Jones, V. R. Leydon, A. K. Oxbrow, C. J. Lewis, M. G. Tennant, S. Modi, D. S. Eggleston, R. J. Chenery and A. M. Bridges, J. Biol. Chem., 2006, 281, 7614 CrossRef CAS PubMed.
- E. M. Cornford, W. D. Bocash, L. D. Braun, P. D. Crane and W. H. Oldendorf, J. Clin. Invest., 1979, 63, 241–1248 CrossRef PubMed.
Footnotes |
† Electronic supplementary information (ESI) available. See DOI: 10.1039/c9md00451c |
‡ Both the authors contributed equally. |
|
This journal is © The Royal Society of Chemistry 2020 |
Click here to see how this site uses Cookies. View our privacy policy here.