DOI:
10.1039/D0MA00596G
(Paper)
Mater. Adv., 2020,
1, 2937-2952
Green synthesis of silver and palladium nanocomposites: a study of catalytic activity towards etherification reaction†
Received
12th August 2020
, Accepted 5th October 2020
First published on 7th October 2020
Abstract
This work deals with the synthesis of biomolecule-based monometallic Ag and Pd and bimetallic Ag–Pd nanocomposites and their catalytic activity towards etherification reaction. The as-synthesized nanocomposites were characterized using N2 adsorption–desorption, high-resolution transmission electron microscopy, Fourier transform infrared spectroscopy, absorption spectroscopy, thermogravimetric analysis, powder X-ray diffraction analysis, X-ray photoelectron spectroscopy and inductively coupled plasma optical emission spectrometry. All three types of nanocatalysts are based on the formation of a complex between the metal and a biomolecule, rutin, with association constant (Ka) values of 3833.33 M−1, 606.48 M−1 and 492.36 M−1 for monometallic Ag and Pd and bimetallic AgPd systems, respectively. The nanocomposites showed catalytic activity towards etherification reactions between different substituted cinnamyl acetate and phenolic compounds under mild and eco-friendly conditions. The values of the product yields were 85–91% with turnover frequency in the range of 17
490–18
724 h−1. The best catalyst was shown to be reusable for 4 cycles without considerable loss in the product yield. The catalytic activity of the Ag-based heterogeneous catalyst towards such O-allylation reaction of phenolic compounds is quite interesting. However, to the best of our knowledge, the literature reports based on such reactions using Ag-based heterogeneous catalysts are still lacking.
Introduction
Nanocomposites have attracted considerable attention in recent years and have become key materials in modern synthetic chemistry due to their unique catalytic activities. Wide applications of nanocatalysts (NCs) in the chemical and pharmaceutical industries have been witnessed, which results from their high surface to volume ratio and easy recovery from the medium after completion of the reactions. Additional benefits are enhancements in energy efficiency with the involvement of green technology, which implies minimization of chemical waste generation.1
Among the numerous nanoparticles, silver nanoparticles represent the most sought-after candidate, mainly due to their unique electrical,2 optical,2–4 catalytic,5 and particularly antimicrobial properties,6,7 which are well established and have been extensively investigated. From a catalysis standpoint, as reported in the literature, the activity of silver is comparable to that of gold but the former one is cheaper to handle. Catalysis by silver is well known for C–H activation, C–C activation, and asymmetric aldol reactions with very high regioselectivity and excellent enantioselectivity towards product formation. The π-Lewis acidity of Ag(I) complexes is utilized for C–C activation in nucleophilic reactions.8
Palladium-based catalysts have become significant and interesting to the modern industrial world because of their superior activity, capacity to inhibit CO formation, high surface to volume ratio, maximum number of active centres, and comparatively lower cost than that of platinum-based catalysts. To enhance the catalytic activity of Pd-based NCs, the dispersed Pd nanoparticles are loaded on a supporting material with a developed specific surface area.9–13
Flavonoid compounds are secondary metabolites in plants that have no direct association with their growth or development. From a green perspective, flavonoid compounds possess good reducing and capping abilities in the synthesis of nanomaterials. Besides their role in nanotechnology, flavonoids have found applications in several other fields.14–17 One such flavonoid is rutin (2-(3,4-dihydroxyphenyl)-5,7-dihydroxy-3-[(2S,3R,4S,5S,6R)-3,4,5-trihydroxy-6-[[(2R,3R,4R,5R,6S)-3,4,5-trihydroxy-6-methyloxan-2-yl]oxymethyl]oxan-2-yl]oxychromen-4-one), the glycoside of quercetin, which is abundantly found in plants, such as in buckwheat seed, fruits, and fruit rinds, especially that of citrus fruits (like orange, grapefruit, lemon, etc.). Rutin can also possess significant scavenging properties towards a few oxidizing species like OH˙, O2˙− and O2˙2− radicals in human systems, as observed in several in vivo and in vitro experiments.18–21
The need for developing greener and biogenic routes in nanomaterial synthesis arises to avoid the use of toxic chemicals, reduce environmental loads, and make the process economically viable.22,23 The choice of solvent, and reducing and/or capping agent for the synthesis of metal nanocomposites plays an important role in such a greener approach and makes the methodology an important finding to the scientific world.24–28 Table S1 (ESI†) summarizes some literature data on the particle size of Ag and Pd nanoparticles depending on the green synthesis conditions and the biomaterials used and finally compares them with our work.
Allyl ethers are considered as one of the useful precursors for several organic reactions including [3,3]-sigmatropic shift, polymerization reactions, 1,3-hydrogen shift, photocatalytic reactions, etc.29,30 They are frequently used as protecting groups for alcoholic compounds.31 These ethers may be present as a core unit in several natural products and also in different biologically active molecules.32 The traditional allyl ether synthesis methods usually involve the use of drastic conditions and highly active equivalents of the allylic group.33 But allyl acetates are considered as a better candidate for allylation as compared to highly activated, unstable allyl halides or tosylates because of their high configurational stability and easy accessibility.34 Usually, allyl ethers can be synthesized using allyl halides and phenols in the presence of strong bases, like metal alkoxides.35 In contrast, the use of soft phenolic nucleophiles for allylation has received less attention. In this regard, complexes of some transition metals, viz. Ru,36 Ni,37 Pd,38 Ir,39etc., have been developed as catalysts to prepare allyl ethers. However, most of the existing methods involve costly non-reusable homogeneous catalysts as well as toxic solvent systems. Hence, the development of an efficient, cost-effective, recyclable and heterogeneous catalytic system is necessary to prepare allyl ethers. Incidentally, Saha et al., and Baig et al., have reported a magnetically separable heterogeneous Pd catalyst for the same.40 In parallel, a Pd metal anchored polystyrenal β-alanine-imine network has been developed by our group for the synthesis of allyl ethers.29 Nevertheless, heterogeneous transition metal nanoparticle-induced syntheses of allyl ethers are one of the most attractive areas. With increasing awareness and the growing importance of developing sustainable, eco-friendly, and economically sound synthetic routes, both Pd- and Ag-based NPs have potential to provide alternative promising solutions for researchers.
In the present study, we chose rutin as a capping agent for the synthesis of Ag nanostructures and both as a reducing and capping agent for Pd nanostructures. The nanocomposites so obtained were characterized using different analytical tools like absorption spectroscopy, N2 adsorption–desorption, field emission scanning electron microscopy (FESEM), transmission electron microscopy (TEM), and so on. Then, these nanocomposites were used as catalysts for the etherification reaction of different substituted cinnamyl acetates and phenolic compounds to find the best conditions of synthesis in water medium under reflux for 18 h (Scheme 1).
 |
| Scheme 1 General reaction scheme of etherification reaction using rutin nanocomposites. | |
Experimental section
Materials
Rutin hydrate (2-(3,4-dihydroxyphenyl)-5,7-dihydroxy-3-[(2S,3R,4S,5S,6R)-3,4,5-trihydroxy-6-[[(2R,3R,4R,5R,6S)-3,4,5-trihydroxy-6-methyloxan-2-yl]oxymethyl]oxan-2-yl]oxychromen-4-one) (≥94%) was obtained from Sigma Aldrich, USA. Silver nitrate (99.9%) was obtained from Glaxo Laboratories Ltd, India. Palladium chloride (59 to 60%) was obtained from Loba Chemie, Mumbai, India. Cinnamyl acetate (99%) was obtained from Sigma Aldrich, USA. p-Cresol (≥99%) and K2CO3 (≥99%) were obtained from Merck Specialities Private Limited, India. CDCl3 was purchased from Sigma Aldrich, USA. Ethyl acetate (≤99.5%) was purchased from Merck Specialities Private Limited, India. Silica Gel G (∼13%) and Silica Gel GF (∼13%) were obtained from Spectrochem Pvt. Ltd, India. The pH of rutin solutions was adjusted using dilute HCl (37%) (Merck) or NaOH (≥97%) (Merck) solutions. All other chemicals were of analytical grade (AR) and used as obtained. All the solvents used were distilled and dried before actual use. Triple distilled water was used throughout the experiments.
Apparatus
Absorption spectra were recorded on a PerkinElmer Lambda 25 UV-Vis spectrophotometer. The temperature-controlled absorbance data were measured using a Varian Cary 50 Bio UV-Vis spectrophotometer. Nitrogen adsorption–desorption isotherms were measured at liquid nitrogen temperature (77 K) using a Quantachrome surface area analyzer. TEM images were obtained using a JEOL JEM 2100 HR with EELS transmission electron microscopy. The Fourier transform infrared (FTIR) spectra of the samples were recorded from 400 to 4000 cm−1 on a PerkinElmer FT-IR 783 spectrophotometer having a resolution of 1 cm−1 using KBr pellets. Powder X-ray diffraction (PXRD) was measured using an X-PERT-PRO Panalytical diffractometer to confirm the actual phase of the prepared material. A centrifuge machine, Remi Elektrotechnik Ltd R-4C, was used to separate the nanocomposites from the aqueous phase. The thermogravimetric analysis of the NCs was carried out using a Diamond TG/DTA Thermogravimetric/Differential Thermal Analyzer of PerkinElmer Instruments in the temperature range from 28 to 439 °C. The concentrations (mol%) of silver and palladium in the nanocomposites were estimated using an inductively coupled plasma-optical emission spectrometry (ICP-OES) instrument (ICAP duo 6500, Thermofisher-Scientific, RF power 1150 Watt, flush pump rate 50 rpm, analysis pump rate 50 rpm, auxiliary gas flow 1.0 L min−1, nebulizer gas flow 0.60 L min−1 and coolant gas flow 12 L min−1, wavelength 224.64 nm, 328.068 nm, and 339.289 nm for silver and 324.27 nm and 340.458 nm for palladium). X-ray photoelectron spectroscopy (XPS) measurement was carried out using a Thermo Scientific ESCALAB 250 Xi system. A Mettler Toledo digital balance correct up to the fourth decimal place was used for measuring the weights of samples. A constant temperature incubator, NOVA Model: SHCI 10(D), was used for obtaining the palladium and silver–palladium nanocomposites. A digital Mettler Toledo Seven Compact pH/ion meter was used to measure and adjust the pH of the rutin solution. Thin-layer chromatography (TLC) was performed on TLC silica gel 60 F254. The products were purified using silica gel (60–120 mesh) column chromatography. Nuclear magnetic resonance (NMR) spectra (1H and 13C NMR) were obtained on a 300 MHz Bruker spectrometer using CDCl3 as a solvent. The 1H and 13C chemical shifts are reported in ppm relative to tetramethylsilane (TMS).
Synthesis of the silver–rutin nanocomposite (Ag@rutin)
5 mL of a 10 mM ethanolic solution of rutin adjusted to pH 12 was treated with 5 mL of a 10 mM aqueous solution of AgNO3. As soon as the addition was complete, the color of the rutin solution changed from faint red to deep brown, indicating the formation of the nanocomposite. To complete the reaction, the solution was kept undisturbed in the dark for 5 to 10 min. A small portion of it was taken out for characterization. The supernatant solution was removed by centrifugation. The obtained solid was then washed two to three times with a water–ethanol (1
:
1) mixture to remove the unreacted rutin and AgNO3. The final product was obtained by drying under an IR lamp.
Synthesis of the palladium–rutin nanocomposite (Pd@rutin)
5 mL of a 10 mM rutin solution adjusted to pH 1 was treated with 5 mL of a 10 mM acidic solution of PdCl2 (prepared in 1 M HCl). Then, the solution was stirred for 5 min. Finally, the mixture was kept at 40 °C for 72 h. After completion of the reaction, a small portion of the colloidal solution was taken out for characterization. The solution was then centrifuged and the supernatant was discarded. The nanocomposite in the centrifugate was washed with a water–ethanol (1
:
1) mixture two to three times and finally dried under an IR lamp.
Synthesis of the silver–palladium–rutin nanocomposite (Ag–Pd@rutin)
5 mL of a 10 mM rutin solution adjusted to pH 1 was treated with 2.5 mL of a 10 mM acidic solution of PdCl2 and 2.5 mL of a 10 mM aqueous solution of AgNO3. Then, the solution was stirred for 5 min. Finally, the mixture was kept at 40 °C for 72 h. A small portion was taken out for characterization. The solution was then centrifuged and the supernatant was discarded. The final product was collected as described above. Scheme 2 gives a clear understanding of the synthesis approach.
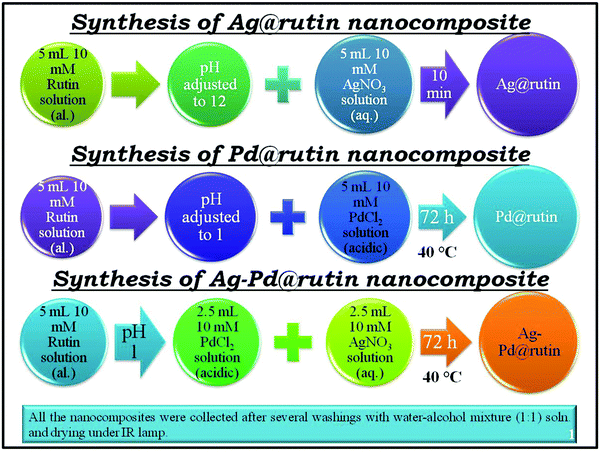 |
| Scheme 2 Synthesis of Ag@rutin, Pd@rutin, and Ag–Pd@rutin nanocomposites. | |
Characterization
Absorption spectroscopy
The metal–rutin complexation was determined using UV-Vis spectrometry at different pHs between 1 and 12. A spectacular color change was observed at pH 1 and 12 (Fig. S1, ESI†), indicating that the most prominent changes occurred at these two pH values. Absorption spectroscopy was therefore used to determine the association constants of metal–rutin complexation at pH 1 and pH 12. Silver–rutin complexation was studied at pH 12 and palladium–rutin complexation and silver–palladium bimetallic complexation with rutin were studied at pH 1 as indicated by the visual color changes. In 2.5 mL of pH adjusted water, 7 μL of a 10 mM ethanolic solution of rutin was added, and then the mixture was treated with the gradual addition (6 μL aliquots) of 1 mM metal ion solutions. The change in absorption data was taken as a function of the concentration of metal ions. Finally, 1/(A − A0) was plotted against 1/[metal], which gave a straight line maintaining the general relationship (Benesi–Hildebrand equation): | 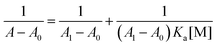 | (1) |
where A0 = the absorbance of the rutin solution in the absence of metal, A1 = absorbance of rutin when it is completely bound with the metal, A = absorbance of rutin with the gradual addition of metal, [M] = concentration of metal, and Ka = binding or association constant.41
The time scan profile obtained using absorption spectroscopy helped to estimate the possible mechanism behind the formation of the nanocomposites.
N2 adsorption–desorption experiment
Before the experiment, the solid material was degassed at ∼300 °C in a surface area analyzer instrument for ∼3 h.
TEM analysis
The nano dimensions of the materials were confirmed by the TEM images. To perform this experiment, a colloidal solution containing each of the nanocomposites was drop cast on a carbon-coated Cu grid. Then, the grid was dried under an IR lamp before experimenting.
FTIR analysis
FTIR analysis was utilized to get a clear idea about the different types of bonds that are present in the nanocomposites. We also extended our study to the change of bond frequencies upon nanocomposite formation from that of a pristine rutin molecule. A little amount of the solid material was mixed thoroughly with dried KBr in a mortar and pestle. Then, the solid mixture was introduced into a steel arrangement followed by application of about 5 tons of pressure on the solid mass through a hydraulic pressure instrument to make a transparent pellet. Finally, the pellet was loaded into a sample holder inside the FTIR instrument analyzer to obtain the spectrum.
Powder XRD
The powder sample was further characterized using X-ray diffraction analysis to ascertain the crystalline nature of the nanocomposites. The powder sample was first taken in a rectangular metal holder and then lightly pressed for PXRD analysis.
Thermogravimetric analysis (TGA)
Thermogravimetric analyses of the three samples were performed to check the thermal properties of the NCs. The experiment was carried out under a N2 atmosphere with a flow rate of 10 mL min−1. Around 6 mg of solid material was taken on a platinum pan and then gradually heated to ∼439 °C with a heating rate of 10 °C min−1 to record the change of weight percentage of the material with the change of temperature.
XPS
XPS is a surface-sensitive technique with a sampling depth of 7.5 nm to 10 nm of the top surface under analysis in the case of polymers.42 XPS was used to confirm the composition and the respective oxidation states of each of the elements present in the nanocomposites. The XPS instrument is equipped with a monochromated Al Kα X-ray source with an X-ray spot size of 900 × 900 μm2. Data were analyzed using Thermo Avantage Software (Version 5.952) using a smart background.
General procedure for O-allylation of cinnamyl acetate with substituted phenols
As described earlier, we have prepared three nanocomposites, namely, Ag@rutin, Pd@rutin, and Ag–Pd@rutin NCs. All of these nanocomposites were used as catalysts for the etherification reaction between cinnamyl acetate (1a) and p-cresol (2a). The general procedures for the reaction are described in the ESI.† The identity of the product was confirmed by 1H and 13C-NMR spectroscopy.
Leaching experiment
The leaching experiment was carried out with all three NCs. After the completion, the reaction mixture was centrifuged. The NC was separated from the reaction mixture and kept aside. Besides, the liquid part containing the reaction mixture was collected and then digested with hot concentrated nitric acid. After three consecutive addition and evaporation steps of the concentrated acid, a solid mass was obtained and it was cooled to room temperature. Thereafter, the solid mass was mixed with a requisite amount of Milli-Q water and then filtered using a microsyringe filter (pore size 0.22 μm). Finally, the filtrate was diluted with water to a specific volume and the resulting solution was analyzed using an ICP-OES instrument.
Recovery of the Pd@rutin nanocomposite
The reusability study was only performed with the Pd@rutin NC as it produced the maximum product yield among the examined NCs. To examine the reusability of the Pd@rutin nanocomposite, an O-allylation reaction was performed using cinnamyl acetate (1a) and p-cresol (2a). After completion of the reaction, the NC was separated from the reaction mixture through centrifugation. Then, the NC was repetitively washed with water and ethyl acetate followed by dichloromethane to remove any traces of the organic part. Finally, the NC was dried at 75 °C for 2 h under vacuum for reactivation. The NC was found to be recyclable for 4 cycles without significant loss in its activity.
Results and discussion
The feed compositions of the nanocomposites of Ag@rutin, Pd@rutin, and Ag–Pd@rutin prepared in different pH media are described with their yields in Table S2 (ESI†).
Absorption spectroscopy
Pd@rutin.
At pH 1 and 40 °C, rutin showed characteristic absorption maxima at three wavelengths (viz. ∼205 (π → π* transition), 250 (π → π* transition) and 350 nm (n → π* transition)). Upon addition of palladium, absorption at 205 and 250 nm increased, and a new shoulder appeared at ∼287 nm. The peak at 250 nm and the shoulder at 287 nm were due to the palladium solution itself under these conditions (Fig. S3, ESI†). The slight change in absorbance at ∼350 nm with the gradual addition of palladium solution to rutin was then the main focus of interaction (Fig. 2). A B–H graph was then plotted at this particular wavelength (Fig. 2 inset) giving an association constant value (Ka) of 606.48 M−1 with a metal:rutin stoichiometry of 1
:
1. The detailed results are tabulated in Table 1.
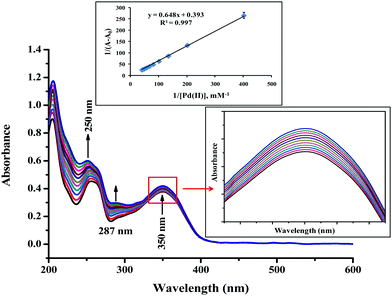 |
| Fig. 2 Absorption spectra for interaction between palladium (2 to 24 μM) and rutin (∼25 μM) at pH 1 with the B–H plot at λmax = 350 nm in the inset. | |
Ag–Pd@rutin.
In the presence of the silver–palladium bimetallic solution, the situation was similar to that of Pd@rutin (Fig. 3). The increase in absorbance at λ = 250 and 287 nm was due to the bimetallic solution at pH 1 and 40 °C (Fig. S4, ESI†). The slight change of absorbance at 350 nm wavelength was due to the interaction between the bimetallic solution and rutin molecules. The B–H plot (Fig. 3 inset (for λ = 350 nm)) was then obtained that suggested a 1
:
1 coordination of metal:rutin with the association constant (Ka) = 492.36 M−1. The detailed results are tabulated in Table 1.
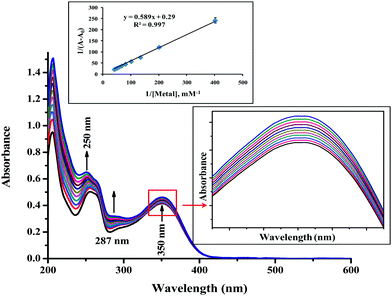 |
| Fig. 3 Absorption spectra for interaction between silver–palladium solution (2 to 24 μM) and rutin (∼25 μM) at pH 1 with the B–H plot at λmax = 350 nm in the inset. | |
Mechanism of nanocomposite formation
The mechanism of nanocomposite formation was studied using an absorption time scan at a particular wavelength taking equal volumes and the same concentration of rutin and metal in the respective pH medium. For the Ag@rutin nanocomposite, equal volumes and the same concentration of AgNO3 and rutin were taken in pH 12 medium and then the absorption time scan was measured at 411 nm up to 45 minutes (2700 s) (Fig. 4). Whereas, for Pd@rutin and Ag–Pd@rutin, equal volumes and the same concentration of rutin and the metal solution were taken in pH 1 medium and then the adsorption time scan was measured at 350 nm up to 45 min (2700 s) (Fig. 4).
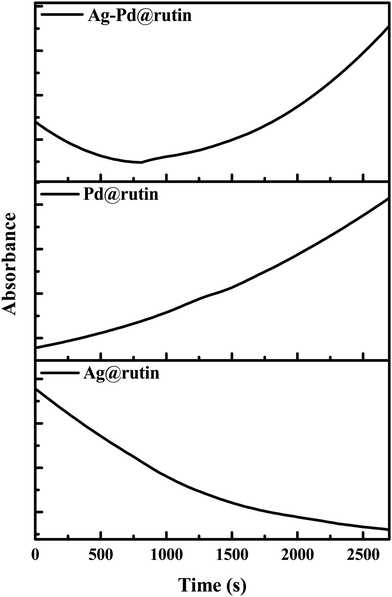 |
| Fig. 4 Time scan data for Ag@rutin (at 411 nm, at room temperature), Pd@rutin (at 350 nm, at 40 °C), and Ag–Pd@rutin (at 350 nm, at 40 °C). | |
For Ag@rutin, a gradual decrease in absorption at 411 nm with time may be due to the rapid reaction between Ag and the rutin moiety, which resulted in the precipitation of the Ag@rutin nanocomposite from the solution.43 Due to such precipitation of the nanocomposite, the amount of free rutin or Ag@rutin in solution decreased, which resulted in a gradual decrease in the absorption of the solution.
For the Pd@rutin nanocomposite, there was a gradual increase in the absorption at 350 nm with time. The above synthesis procedure suggested that about 72 h was required to synthesize the Pd@rutin nanocomposite. So, we may say that this gradual increase of the absorption suggested slow and steady nucleation and growth of the nanocomposite, as described in the classical approach of nucleation and growth.44
For the bimetallic nanocomposite, the situation is quite similar and resultant of the mechanistic pathway of both Ag and Pd. The rapid decrease in the absorption in the first few minutes was due to the interaction between Ag and rutin, whereas the latter increase in the absorption was due to the interaction between Pd and rutin following the classical nucleation and growth mechanism.44
N2 adsorption–desorption and BET analysis
Fig. 5 shows the N2 adsorption–desorption isotherms of Ag@rutin, Pd@rutin, and Ag–Pd@rutin nanocomposites. The isotherm for Ag@rutin resembled type IV with the H4 hysteresis loop. This type of isotherm corresponds to a mesoporous adsorbent and the adsorption behavior can be determined from the adsorbent–adsorptive interaction. This type of adsorption was observed due to the simultaneous occurrence of monolayer and multilayer adsorption on the surface by a pore condensation mechanism. Particle aggregation in the nano-state developed this type of hysteresis (H4).45 For Pd@rutin and Ag–Pd@rutin, the isotherms looked like type II with a H3 hysteresis loop. This convex type of isotherm is generally observed for free monolayer-multilayer adsorption on the surface of the adsorbent at high P/P0 values with a tiny knee at lower P/P0 due to completion of monolayer coverage. The knee is characteristic of an overlap of monolayer adsorption and the beginning of multilayer adsorption. The H3 hysteresis is common for a particle having a plate-like assembly at the surface.45 The difference in the surface properties of Ag@rutin from those of Pd@rutin and Ag–Pd@rutin may have occurred due to the reaction time of the formation of the nanocomposites. For Ag@rutin, aggregated particles were mostly formed due to the rapid development of the nanocomposite. For Pd@rutin and Ag–Pd@rutin, they took sufficient time to form the nanocomposites and developed one layer on top of the other, giving a plate-like assembly.
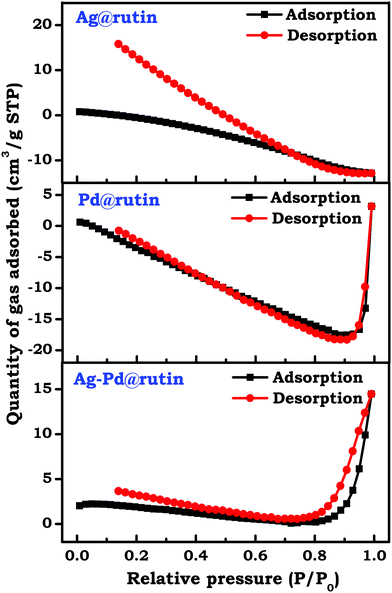 |
| Fig. 5 N2 adsorption–desorption isotherms of the prepared NCs. | |
TEM analysis
The TEM micrographs of the nanocomposites reflect their nano-dimensional properties (Fig. 6). For Ag@rutin, the figure itself and the inset suggest the aggregated nature of the nanocomposite along with the lattice fringes. The porous nature of the Ag@rutin nanocomposite can also be confirmed from these images. For Pd@rutin, the TEM image (Fig. 6) showed discrete smaller particles with clear lattice fringes. A layered structure with a porous nature of the Pd@rutin nanocomposite can also be viewed from the image itself and the inset picture. For Ag–Pd@rutin, the TEM image (Fig. 6) shows aggregated particles with very insignificant lattice fringes. The porous nature cannot be confirmed from this image, whereas the corresponding inset picture indicates a plate-like assembly of the Ag–Pd@rutin nanocomposite. This was the combined result of both Ag@ruitn and Pd@rutin. The small reaction time of the Ag@rutin nanocomposite allowed aggregated particles to form that deposited readily within 5 to 10 min. For the Pd@rutin nanocomposite, the comparatively long reaction time with a very small deposition of the nanocomposite at the bottom of the container after the completion of the reaction suggested that discrete smaller particles were formed with layers of the nanocomposite depositing one after the other. For Ag–Pd@rutin, although the reaction time and type of deposition were similar to that of the Pd@rutin nanocomposite, the overall particle nature was the result of the conjugate effect of both the metals. The particle agglomeration mainly occurred due to silver and the layer like assemblies were observed due to palladium.
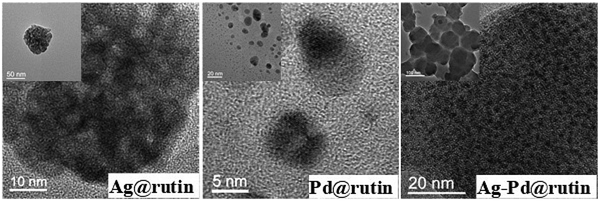 |
| Fig. 6 TEM micrographs of the prepared NCs (The inset image shows an overall picture of the nanocomposite). | |
The elemental mapping of the NCs is shown in Fig. 7. The red color indicates the Ag metal in Ag@rutin NC, whereas green indicates the Pd metal in Pd@rutin NC. In the bimetallic nanocomposite, the efficient mixing of these two colors suggests alloy formation between the two metals, Ag and Pd, in the Ag–Pd@rutin nanocomposite. The alloy formation is also supported by results obtained from PXRD and XPS analyses, discussed later in the XPS section.
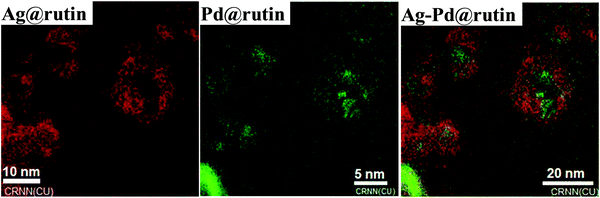 |
| Fig. 7 TEM mapping of the NCs (color code: red for Ag metal, and green for Pd metal). | |
The histograms of the nanocomposites (Fig. 8) suggested that the average particle size of Ag@rutin, Pd@rutin, and Ag–Pd@rutin was ∼50 nm, ∼10 nm, and ∼80 nm, respectively.
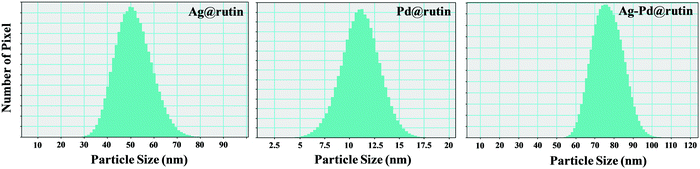 |
| Fig. 8 Histogram of the prepared NCs. | |
FTIR spectral analysis
Fourier transform infrared spectra of the nanocomposites along with the pure rutin compounds are shown in Fig. 9. Although there were several peaks, we were mainly interested in the zone of 500 to 1650 cm−1, which was modified to a good extent upon the addition of different metal solutions. For the Ag@rutin nanocomposite, there was a peak shift from 1362 to 1376 cm−1 (stretching of the C–O–C bond)46 due to the modification of silver on the rutin molecule. At the same time, the multiplet like feature of the pure rutin also disappeared upon modification by silver. For the Pd@rutin nanocomposite, again, the appearance of the multiplet was observed but its peak intensity was quite low. At the same time, there was a new peak at 590 cm−1 due to the formation of the Pd–O bond.47 For the Ag–Pd@rutin nanocomposite, the FTIR spectrum was quite similar to that of the Pd@rutin nanocomposite, indicating that upon bimetallic modification, there was a greater conjugation with palladium than silver metal due to the long reaction time. A similar observation was also obtained from XPS analysis as discussed later. Table 2 describes the different frequencies with possible functionalities obtained from the FTIR analysis.
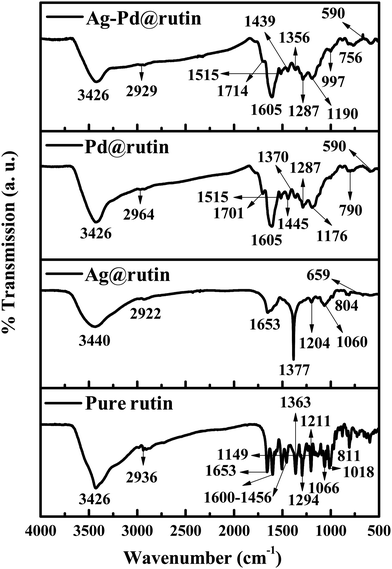 |
| Fig. 9 FTIR spectra of the prepared NCs and pure rutin. | |
Table 2 FTIR spectral data of the prepared samples and pure rutin with their possible functionalities
Sample |
Peak position (cm−1) |
Functionality |
Ref. |
Pure rutin |
3426 |
Aromatic –OH stretching |
46
|
2936 |
Asymmetric –CH2 + asymmetric –CH3 |
1653 |
C O stretching |
1600–1456 |
C–C stretching |
1363 |
C–O–C stretching |
1294 |
1211 |
1149 |
Stretching and bending of the keto group |
1066 |
C–O–C stretching |
1018 |
811 |
C–H stretching |
|
Ag@rutin NC |
3440 |
Aromatic –OH stretching |
46
|
2922 |
C–H stretching |
1653 |
C O stretching |
1377 |
C–O–C stretching |
1204 |
1060 |
804 |
C–H stretching |
659 |
O–H deformation |
47
|
|
Pd@rutin NC |
3426 |
Aromatic –OH stretching |
46
|
2964 |
C–H stretching |
1701 |
C O stretching |
47
|
1605 |
C–C stretching |
46
|
1515 |
1445 |
1370 |
C–O–C stretching |
1287 |
1176 |
Stretching and bending of the keto group |
790 |
C–H vibration |
47
|
590 |
Pd–O bond vibration |
46
|
|
Ag–Pd@rutin NC |
3426 |
Aromatic –OH stretching |
46
|
2929 |
Asymmetric –CH2 + asymmetric –CH3 stretching |
1714 |
C O stretching |
47
|
1605 |
C–C stretching |
46
|
1515 |
1439 |
1356 |
C–O–C stretching |
1287 |
1190 |
997 |
756 |
C–H vibration |
47
|
590 |
Pd–O bond vibration |
46
|
PXRD analysis
The obtained nanocomposites were further characterized by powder X-ray diffraction analysis (Fig. 10). The PXRD data so obtained were then compared with the unique number obtained from the Joint Committee on Powder Diffraction Standards (JCPDS). For the Ag@rutin nanocomposites, the major diffractions located at 2θ values of 31.84°, 38.25°, 44.44°, and 45.57°, which correspond to the (060), (111), (200) and (100) planes. For the Pd@rutin nanocomposites, the most intense diffraction came from the (023) plane at a 2θ value of 40.24°. Whereas, the Ag–Pd@rutin nanocomposite showed diffraction mainly at four positions having 2θ values of 27.94°, 32.35°, 40.11°, and 46.34°. The PXRD data of the prepared samples are tabulated in Table 3 with possible (hkl) planes and JCPDS numbers.
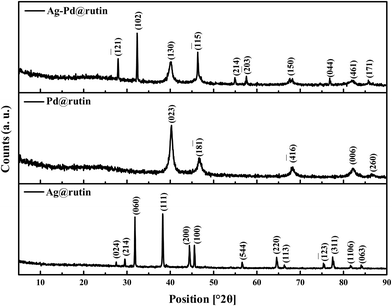 |
| Fig. 10 PXRD patterns of the prepared NCs. | |
Table 3 PXRD data of the prepared nanocomposites and possible assignment of JCPDS no
Sample |
Ag@rutin NC |
Obtained position [°2θ] |
Actual position [°2θ] |
Possible hkl plane |
Possible lattice |
JCPDS no. |
27.513 |
27.493 |
024 |
Rhomb-centered |
87-0422 |
29.531 |
29.608 |
214 |
Rhomb-centered |
87-0422 |
31.846 |
31.770 |
060 |
Primitive |
79-2441 |
38.259 |
38.202 |
111 |
Face-centered |
87-0720 |
44.441 |
44.402 |
200 |
Face-centered |
87-0720 |
45.578 |
45.539 |
100 |
Primitive |
41-1402 |
56.588 |
56.508 |
544 |
Rhomb-centered |
87-0422 |
64.562 |
64.426 |
220 |
Face-centered |
04-0783 |
66.329 |
66.315 |
113 |
Primitive |
89-3081 |
75.392 |
75.323 |
.23 |
Primitive |
89-3081 |
77.497 |
77.472 |
311 |
Face-centered |
04-0783 |
81.679 |
81.606 |
1106 |
Rhomb-centered |
87-0422 |
84.079 |
84.136 |
063 |
Primitive |
84-0713 |
|
Pd@rutin NC |
40.243 |
40.263 |
023 |
End-centered |
79-0322 |
46.723 |
46.733 |
18![[1 with combining macron]](https://www.rsc.org/images/entities/char_0031_0304.gif) |
Primitive |
45-0773 |
68.198 |
68.141 |
16 |
Primitive |
89-6629 |
82.234 |
82.245 |
006 |
End-centered |
79-0264 |
86.692 |
86.619 |
260 |
End-centered |
79-0264 |
|
Ag–Pd@rutin NC |
27.949 |
27.736 |
12![[1 with combining macron]](https://www.rsc.org/images/entities/char_0031_0304.gif) |
Primitive |
45-0773 |
32.357 |
32.467 |
102 |
Primitive |
50-1058 |
40.111 |
40.116 |
130 |
End-centered |
79-0264 |
46.344 |
46.380 |
15 |
Primitive |
89-6629 |
54.959 |
54.909 |
214 |
Primitive |
89-6629 |
57.568 |
57.499 |
03 |
Primitive |
51-1440 |
67.852 |
67.899 |
150 |
End-centered |
79-0264 |
76.814 |
76.876 |
044 |
End-centered |
79-0264 |
82.058 |
82.175 |
461 |
End-centered |
79-0322 |
85.806 |
85.812 |
171 |
End-centered |
79-0322 |
TGA analysis
The TGA graphs of the samples (Fig. 11) indicate the stability of the prepared NCs up to ∼200 °C. Only ∼15%, 10%, and 4% mass loss occurred at ∼100 °C for Ag@rutin, Pd@rutin, and Ag–Pd@rutin NCs, respectively, which may be due to the degradation of the polyphenol molecules present in the NCs. As our reaction proceeded at ∼100 °C, we can conclude that the NCs are stable enough at this temperature and are capable of catalyzing the reaction.
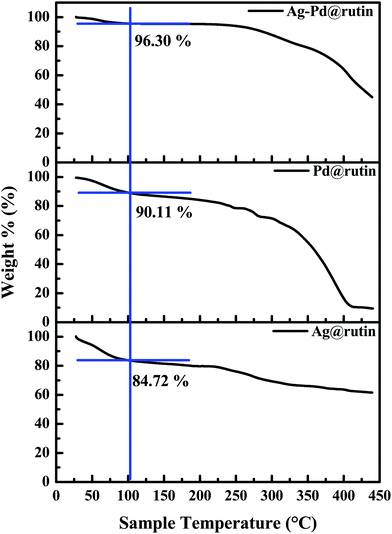 |
| Fig. 11 TGA graphs of all the NCs. | |
XPS analysis
XPS is a surface-sensitive technique with a sampling depth of 7.5 nm to 10 nm of the top surface under analysis in the case of polymers.42 Understandably, the analysis carried out using XPS would certainly provide details of up to 10 nm depth of the top surface layer, rather than the bulk material. Also, the surface free energy of rutin is a lot lower than those of Ag and Pd.48 Pd has a much higher surface free energy (1950 mJ m−2)48 than that of Ag (1300 mJ m−2).48 For that reason, when in a composite, it is expected that Pd would locate underneath the top surface layer to decrease surface free energy for stable equilibrium. With silver having a lower surface free energy and higher sensitivity to XPS, when a composite made up of Ag and Pd and biomaterials is under investigation by XPS, the detected amount of Ag is expected to be higher. To extract specific information from the XPS core-level spectra, deconvolution into several component peaks was carried out, revealing oxidation states along with the bonding environment of the element. However, without cross-matching with each element (for example, if Ag2p spectra are analyzed to find oxidation states, O1s spectra should also be analyzed and referenced appropriately to complete the set), peak assignment solely based on comparisons to the reference binding energy values was incomplete. Binding energy cross-matching with references can only provide some indication and can only be used as a necessary but not sufficient condition to claim bond formation.49 In our present set of samples, the O1s binding energy region (∼531 eV) was overlapped with Pd3p3/2 (∼530 eV). The amount of oxygen (O1s) quantified does include contributions from Pd3p regions and for that reason, the amount reported here from the survey spectra is just for indication and trends only. For the same reason, O1s narrow scan data has not been analyzed in this report either.
The survey scan spectra of these Ag@rutin, Pd@rutin, and Ag–Pd@rutin nanocomposites are shown in the ESI,† Fig. S5. As Ag has a lower surface free energy and higher sensitivity towards XPS, a lot more amount of Ag can be detected than Pd, as reflected in Table 4.
Table 4 Atomic percentages of Ag3d5/2, Pd3d5/2, O1s, C1s, and Cl2p in the prepared NCs
Sample |
Atomic % of |
Ag3d5/2 |
Pd3d5/2 |
O1s |
C1s |
Cl2p |
Ag–Pd@rutin |
0.3
|
0.3
|
29.8 |
67.1 |
2.6 |
Ag@rutin |
25.6
|
0.0 |
29.9 |
42.7 |
1.9 |
Pd@rutin |
0.0 |
0.7
|
32.2 |
66.5 |
0.7 |
In Fig. 12a–d, the narrow scan spectra of silver and palladium are shown. All narrow scan spectral positions were referenced by fixing the C1s sp2–sp3 mixed hybridized position at 284.7 eV. Rutin has both sp2 and sp3 hybridized carbons and differentiating sp2 and sp3 carbons requires further analysis. A combined peak position was the reference position and it was fixed to calculate charge shifting of the narrow scan peaks. C1s narrow scan spectra of all the samples are shown in the ESI,† Fig. S6.
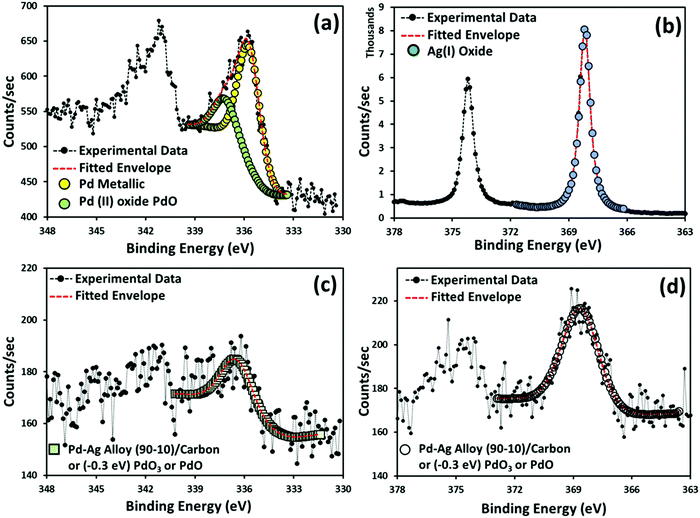 |
| Fig. 12 XPS narrow scan deconvoluted spectra of Pd3d5/2 and Ag3d5/2 of the samples (a) Pd@rutin NC, (b) Ag@rutin NC and (c) and (d) Ag–Pd@rutin NC. Deconvoluted peaks are assigned following the NIST XPS database and references are freely available from the NIST website. | |
In the case of the Pd@rutin nanocomposite (Fig. 12a), Pd showed the presence of metallic palladium50 and palladium(II) oxide.51 There was no silver present in this sample. Silver in the Ag@rutin nanocomposite (Fig. 12b) was present as silver(I) oxide (Ag2O). However, things were different in the case of the Ag–Pd@rutin nanocomposite. XPS revealed some very interesting phenomena happening in these synthesized nanocomposites. As shown in Fig. 12c and d, the Pd3d5/2 peak was positioned at 336.4 eV and the Ag3d5/2 peak was positioned at 368.6 eV. There might have been the formation of Pd–Ag alloy and as per the NIST XPS database, palladium–silver alloy (90–10)/carbon is the compound.52 Using density functional theory (DFT) and by calculating the segregation energies for binary alloy nanoparticles, it has previously been reported that the formation of a Pd core and Ag shell is energetically favorable.53 To cross-check the alloy formation, Ag–Pd alloy should have XRD peaks at 2θ = 39.92° and 2θ = 46.29° corresponding to the 111 and 200 planes, respectively, of Ag–Pd alloy.54 As shown in the PXRD table (Table 3), the Ag–Pd@rutin nanocomposite has a couple of major peaks at 2θ = 40.11° and 2θ = 46.34°. So, these results matched very closely with the palladium silver alloy formation theory, supported by both PXRD and XPS techniques. There was another explanation that could also be investigated. It has been previously reported that Ag3d5/2 and Pd3d5/2 peaks are shifted by 0.3 eV towards a higher BE due to the Ag–Pd interaction55 due to the charge transfer between Ag and Pd.56,57 Considering the extra 0.3 eV charge shifting towards a higher BE, the Ag3d5/2 peak corresponded to silver(I) oxide (Ag2O)58 and the Pd3d5/2 peak corresponded to either palladium(VI) oxide (PdO3)59 or palladium(II) oxide (PdO). The charge shift happened due to charge transfer between Ag and Pd. These results provided evidence of bimetallic interfaces between Ag and Pd, which are partially responsible for the high catalytic activity.
Catalytic activity of the prepared nanocomposites
Catalytic activity of the prepared nanocomposites was examined for the O-allylation reaction of phenolic compounds. Our preliminary study aimed to screen the catalyst using cinnamyl acetate (1a) and p-cresol (2a) as the model substrates (Table 5). The as-synthesized three NCs, Ag@rutin, Pd@rutin, and Ag–Pd@rutin nanocomposites, were used for this study. It was found that both Ag@rutin and Pd@rutin nanocomposites were successful in furnishing the corresponding ether (Table 5, entries 1 and 2). The reactivity of the Pd@rutin nanocomposite was much greater than that of the Ag@rutin system. The activity of the Ag–Pd@rutin nanocomposite was also examined and was found to have nearly the same reactivity as that of the Ag@rutin nanocomposite (Table 5, entry 3). The reaction optimization using Ag@rutin and Ag–Pd@rutin NCs using the same reaction was performed and the results are tabulated in Tables S3 and S4, respectively, in the ESI.† From the TEM data, we found that the average particle size of Ag@rutin was much larger than that of Pd@rutin. Therefore, Pd@rutin having a smaller particle size can provide a higher surface area for the substrates and thus furnished higher product yields than Ag@rutin. Similarly, the Ag–Pd@rutin bimetallic nanocomposite furnished comparatively lower product yields due to the large size of the Ag@rutin NC in the nanocomposite that decreased the availability of the reactive but small Pd NC. However, this result is quite interesting as no report is available in the literature regarding a Ag NC-based catalyst induced O-allylation reaction of phenolic compounds to the best of our knowledge. Finally, the activity of rutin was also examined and it was found that rutin has no catalytic activity in this reaction system (Table 5, entry 4). The most probable reaction mechanism is presented in Scheme 3. The reaction proceeds through an interaction of Pd(II) or Ag (I) with cinnamyl acetate followed by oxidative addition with p-cresol resulting in the formation of a η3-π-allyl complex that undergoes reductive elimination to give the final product,29 which was further characterized by 1H and 13C-NMR spectroscopy (Fig. S5–S9 in the ESI†).
Table 5 Screening of the catalysts for O-allylation of phenolic compoundsa
Entry |
Catalyst |
Time (h) |
Yieldb (%) |
Conditions: cinnamyl acetate (1 mmol), p-cresol (1 mmol), K2CO3 (2 mmol), catalyst (0.00027 mol%), and water, reflux.
Yields refer to those of isolated products obtained by column chromatography.
6 mg of catalyst.
|
1 |
Ag@rutin |
18 |
75 |
2 |
Pd@rutin |
18 |
88 |
3 |
Ag–Pd@rutin |
18 |
72 |
4c |
Rutin |
25 |
— |
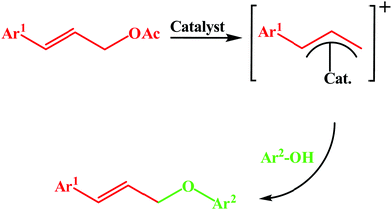 |
| Scheme 3 Plausible mechanism of the etherification reaction using the nanocatalyst. | |
After screening the catalysts, a series of reactions was performed to get the optimized reaction conditions using the Pd@rutin NC. Among various solvents (DMF, toluene, acetonitrile, xylene), water gave the best result in terms of yields (Table 6, entries 1–5). Besides, K2CO3 was found to be suitable as a base as compared to NaHCO3, Cs2CO3, K3PO4 (Table 6, entries 5–8). All the reactions were performed under refluxing conditions as a high temperature was required for the activation of the catalyst (Table 6, entries 9, and 10). During the experiments on the reaction, no product was formed without any catalyst even after 30 h. This observation indicates the necessity of the catalyst (Table 6, entry 11). The best product yield was obtained using water as a green solvent and K2CO3 as a base under refluxing conditions for 18 h with stirring using 0.00027 mol% Pd@rutin NC.
Table 6 Standardization of reaction conditionsa

|
Entry |
Solvent |
Base |
Temp. (°C) |
Time (h) |
Yieldb (%) |
Conditions: cinnamyl acetate (1 mmol), p-cresol (1 mmol), base (2 mmol), Pd@rutin (6 mg, 0.00027 mol%), solvent, temp., and time.
Yields refer to those of isolated products obtained by column chromatography.
Without any catalyst.
|
1 |
DMF |
K2CO3 |
120 |
18 |
68 |
2 |
Toluene |
K2CO3 |
110 |
18 |
14 |
3 |
Acetonitrile |
K2CO3 |
78 |
18 |
31 |
4 |
Xylene |
K2CO3 |
120 |
18 |
9 |
5
|
Water
|
K
2
CO
3
|
100
|
18
|
88
|
6 |
Water |
NaHCO3 |
100 |
18 |
82 |
7 |
Water |
Cs2CO3 |
100 |
18 |
41 |
8 |
Water |
K3PO4 |
100 |
18 |
41 |
9 |
Water |
K2CO3 |
60 |
18 |
59 |
10 |
Water |
K2CO3 |
rt |
30 |
— |
11c |
Water |
K2CO3 |
100 |
30 |
— |
After optimization of the reaction conditions, we started to explore the versatility of the Pd@rutin nanocomposites. Several substituted cinnamyl acetates and phenolic compounds were studied through this reaction to produce the corresponding product ethers (Table 7). Phenols containing an electron-donating group (–Me) and withdrawing group (–Br) in their aromatic ring underwent this etherification reaction smoothly. Similarly, cinnamyl acetate with –Me and –OMe substitution in its aromatic ring also reacted to give the corresponding ethers with good yields (entries 3c–e, Table 7).
Table 7 Synthesis of allyl aryl ether using the Pd@rutin NC
TOF = TON/time, [TON = moles of substrate converted per mole of active site] |
|
Recyclability
The Pd@rutin NC can be reused for 4 consecutive cycles. The reusability chart is presented in Fig. 13. After the recycling experiment, the NC was analyzed thoroughly by FTIR spectroscopic analysis (after the 4th run) (Fig. 14). A table (Table 8) was also made to compare the FTIR peak position of the fresh Pd@rutin catalyst to that of the catalyst after the 4th cycle. The results of the analysis indicates that the nanocomposites were significantly stable up to 4 cycles. Moreover, the leaching experiment of the reaction mixture was also performed by ICP-OES analysis, and no Ag or Pd NPs were detected in the solution, or in the case where Pd was detected, it was below the limit of detection for any of the nanocomposites. Finally, the utility of our newly developed methodology is compared with literature reports and is demonstrated in Table S5 (ESI†). It can be seen that entry 1 in Table S5 (ESI†) suggests a lower time of reaction and higher reusability than our work, but the present work not only deals with the catalytic activity of the nanocomposites but also offers a green synthesis of the nanocatalysts. Both entry 1 and the present work show similar catalytic activity, but the synthesis of the catalyst in entry 1 neither used a biogenic source nor involved a green methodology.
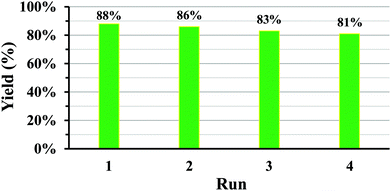 |
| Fig. 13 Recyclability chart for the etherification reaction using the Pd@rutin NC. | |
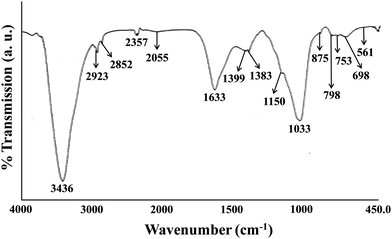 |
| Fig. 14 FTIR spectra of the reused NC (Pd@rutin). | |
Table 8 FTIR spectral data of the Pd@rutin NC and after four catalyst cycles
Initial peak position (cm−1) |
Peak position (cm−1) after reuse |
3426 |
3426 |
2964 |
2923, 2852, 2357 |
1701, 1605, 1515, 1445 |
1633 |
1370 |
1399, 1383 |
1287, 1176 |
1150, 1033, 875 |
790 |
798, 753, 698 |
590 |
561 |
Conclusion
In this work, we have prepared Ag, Pd, and Ag–Pd bimetallic nanocomposites using a greener approach to reduce the environmental load and established interesting catalytic activity of the nanocomposites. We have characterized these metal nanocomposites using different analytical tools like – absorption spectroscopy, N2 adsorption–desorption, TEM, FTIR, PXRD, and XPS analyses. Finally, the catalytic activity of the prepared nanocomposites towards the etherification reaction was studied. From the study, we confirmed that the palladium nanocomposites showed higher catalytic activity than bimetallic and Ag@rutin nanocomposites towards this studied reaction. The Pd@rutin NC can also be reused up to four cycles without considerable loss in product yield. Additionally, the role of Ag in catalyzing such reactions has been demonstrated for the first time to the best of our knowledge with a fairly encouraging yield. It has been perceived that although the formation of Pd@rutin shows a slow formation rate, it exhibits a smaller particle size with dual oxidation states (Pd(II) and Pd(0)) and a better catalytic activity than those of the other two materials. The most significant observation is the drastically reduced amount of the costly metal Pd that is required for the catalysis as compared to homogeneous catalysis. The amount of active catalyst required is only 0.00027 mol% of the material, which is again reusable after regeneration for at least 4 cycles. This is in coherence with the results of the ICP-OES analysis of the reaction media for the leached out metal. Additionally, the reaction proceeds in a water medium with 88% product yield. Therefore, in a nutshell, the work is in agreement with green chemistry perspectives and opens a vast field of organic synthesis with the easy synthesis and commendable use of such potential catalysts.
Conflicts of interest
There are no conflicts to declare.
Acknowledgements
PS expresses sincere thanks to the University Grants Commission (UGC) (Ref. No. of PS 20/12/2015(ii)EU-V dated 24.08.2016) for providing the necessary fellowship. The authors would also like to acknowledge the funding received under the Global Challenge Research Fund (GCRF) provided by the University of Brighton, UK. We also express our sincere gratitude to Mr Nayan Ranjan Saha, Department of Chemical Technology, University of Calcutta, India for carrying out the PXRD analysis. We want to express our sincere gratitude to Prof. Susanta Lahiri and his fellow Dr Nabanita Naskar, Chemical Science Division, Saha Institution for Nuclear Physics, India for performing ICP-OES of our samples to determine the mol percentage of the NCs. We are also thankful to Prof. Abhijit Chakrabarti and his scholar Dr Dipayan Bose, Crystallography & Molecular Biology Division, Saha Institute of Nuclear Physics, India for helping to measure the absorbance data at 40 °C.
References
- N. Sharma, H. Ojha, A. Bharadwaj, D. P. Pathak and R. K. Sharma, Preparation and catalytic applications of nanomaterials: A review, RSC Adv., 2015, 5(66), 53381–53403 RSC.
- P. Saravanan, M. P. Raju and S. Alam, A study on synthesis and properties of Ag nanoparticles immobilized polyacrylamide hydrogel composites, Mater. Chem. Phys., 2007, 103(2–3), 278–282 CrossRef CAS.
- Y. M. Mohan, T. Premkumar, K. Lee and K. E. Geckeler, Fabrication of silver nanoparticles in hydrogel networks, Macromol. Rapid Commun., 2006, 27(16), 1346–1354 CrossRef CAS.
- J. Zhang, S. Xu and E. Kumacheva, Polymer microgels: Reactors for semiconductor, metal, and magnetic nanoparticles, J. Am. Chem. Soc., 2004, 126(25), 7908–7914 CrossRef CAS.
- Y. Lu, P. Spyra, Y. Mei, M. Ballauff and A. Pich, Composite hydrogels: Robust carriers for catalytic nanoparticles, Macromol. Chem. Phys., 2007, 208(3), 254–261 CrossRef CAS.
- Y. M. Mohan, K. Lee, T. Premkumar and K. E. Geckeler, Hydrogel networks as nanoreactors: a novel approach to silver nanoparticles for antibacterial applications, Polymer, 2006, 48(1), 158–164 CrossRef.
- E. A. Deitch, A. A. Marino, V. Malakanok and J. A. Albright, Silver nylon cloth: In vitro and in vivo evaluation of antimicrobial activity, J. Trauma, 1987, 27(3), 301–304 CrossRef CAS.
- V. K.-Y. Lo, A. O.-Y. Chan and C.-M. Che, Gold and silver catalysis: From organic transformation to bioconjugation, Org. Biomol. Chem., 2015, 13(24), 6667–6680 RSC.
- Z. Yin, H. Zheng, D. Ma and X. Bao, Porous palladium nanoflowers that have enhanced methanol electro-oxidation activity, J. Phys. Chem. C, 2009, 113(3), 1001–1005 CrossRef CAS.
- Y. Huang, X. Zhou, J. Liao, C. Liu, T. Lu and W. Xing, Preparation of Pd/C catalyst for formic acid oxidation using a novel colloid method, Electrochem. Commun., 2008, 10(4), 621–624 CrossRef CAS.
- X. Chen, G. Wu, J. Chen, X. Chen, Z. Xie and X. Wang, Synthesis of “clean” and well-dispersive Pd nanoparticles with excellent electrocatalytic property on graphene oxide, J. Am. Chem. Soc., 2011, 133(11), 3693–3695 CrossRef CAS.
- Y. Zhao, L. Zhan, J. Tian, S. Nie and Z. Ning, Enhanced electrocatalytic oxidation of methanol on Pd/polypyrrole–graphene in alkaline medium, Electrochim. Acta, 2011, 56(5), 1967–1972 CrossRef CAS.
- Y. Zhang, H. Shu, G. Chang, K. Ji, M. Oyama, X. Liu and Y. He, Facile synthesis of palladium–graphene nanocomposites and their catalysis for electro-oxidation of methanol and ethanol, Electrochim. Acta, 2013, 109, 570–576 CrossRef CAS.
- T. Iwashina, The structure and distribution of the flavonoids in plants, J. Plant Res., 2000, 113(3), 287–299 CrossRef CAS.
- L. C. Chiang, W. Chiang, M. C. Liu and C. C. Lin, In vitro antiviral activities of caesalpinia pulcherrima and its related flavonoids, J. Antimicrob. Chemother., 2003, 52(2), 194–198 CrossRef CAS.
- S. Khadem and R. J. Marles, Chromone and flavonoid alkaloids: Occurrence and bioactivity, Molecules, 2012, 17(1), 191–206 CrossRef CAS.
- T. P. T. Cushnie and A. J. Lamb, Recent advances in understanding the antibacterial properties of flavonoids, Int. J. Antimicrob. Agents, 2011, 38(2), 99–107 CrossRef CAS.
- A. Korkmaz and D. Kolankaya, The protective effects of ascorbic acid against renal ischemia-reperfusion injury in male rats, Renal Failure, 2009, 31(1), 36–43 CrossRef CAS.
- A. R. Verma, M. Vijayakumar, C. S. Mathela and C.
V. Rao, In vitro and in vivo antioxidant properties of different fractions of moringa oleifera leaves, Food Chem. Toxicol., 2009, 47(9), 2196–2201 CrossRef CAS.
- S. Itagaki, J. Oikawa, J. Ogura, M. Kobayashi, T. Hirano and K. Iseki, Protective effects of quercetin-3-rhamnoglucoside (rutin) on ischemia-reperfusion injury in rat small intestine, Food Chem., 2010, 118(2), 426–429 CrossRef CAS.
- M. L. Calabrò, S. Tommasini, P. Donato, R. Stancanelli, D. Raneri, S. Catania, C. Costa, V. Villari, P. Ficarra and R. Ficarra, The rutin/β-cyclodextrin interactions in fully aqueous solution: Spectroscopic studies and biological assays, J. Pharm. Biomed. Anal., 2005, 36(5), 1019–1027 CrossRef.
- V. Parashar, R. Parashar, B. Sharma and A. C. Pandey, Parthenium leaf extract mediated synthesis of silver nanoparticles: A novel approach towards weed utilization, Dig. J. Nanomater. Bios., 2009, 4(1), 45–50 Search PubMed.
- N. A. Begum, S. Mondal, S. Basu, R. A. Laskar and D. Mandal, Biogenic synthesis of Au and Ag nanoparticles using aqueous solutions of black tea leaf extracts, Colloids Surf., B, 2009, 71(1), 113–118 CrossRef CAS.
- M. Ramya and M. S. Subapriya, Green synthesis of silver nanoparticles, Int. J. Pharma Med. Biol. Sci., 2012, 1(1), 54–61 CAS.
-
P. T. Anastas and J. C. Warner, Green chemistry: Theory and practice, Oxford University Press, Oxford, UK, 1998 Search PubMed.
- C.-J. Li and L. Chen, Organic chemistry in water, Chem. Soc. Rev., 2006, 35(1), 68–82 RSC.
- D. Dallinger and C. O. Kappe, Microwave-assisted synthesis in water as solvent, Chem. Rev., 2007, 107(6), 2563–2591 CrossRef CAS.
- M. N. Nadagouda and R. S. Varma, Green synthesis of Ag and Pd nanospheres, nanowires, and nanorods using vitamin B2: Catalytic polymerisation of aniline and pyrrole, J. Nanomater., 2008, 2008, 1–8 CrossRef.
- M. Halder, Md. M. Islam, S. Ahammed and Sk. M. Islam, Polymeric β-alanine incarcerated Pd(II) catalyzed allylic etherification in water: A mild and efficient method for the formation of C(sp3)–O bonds, RSC Adv., 2016, 6(10), 8282–8289 RSC.
-
(a)
J. Tsuji, Handbook of Organopalladium Chemistry for Organic Synthesis, Wiley, New York, 2002, vol. 5, p. 1669 Search PubMed;
(b)
S. A. Godleski, Comprehensive organic synthesis, Pergamon, Oxford, 1991, vol. 4, p. 585 Search PubMed.
- S. Tanaka, H. Saburi, Y. Ishibashi and M. Kitamura, CpRuIIPF6/quinaldic acid-catalyzed chemoselective allyl ether cleavage. A simple and practical method for hydroxyl deprotection, Org. Lett., 2004, 6(11), 1873–1875 CrossRef CAS.
-
(a) A. Monte, M. S. Kabir, J. M. Cook, M. Rott, W. R. Schwan and L. Defoe, U. S. Pat. Appl. Publ., 2007, 37, 60 Search PubMed;
(b) O. E. O. Hormi and L. Hirvela, New synthetic approaches to 6-thiophenoxysalicylates, 6-phenoxysalicylates and 1-hydroxy-9-xanthones, Tetrahedron Lett., 1993, 34(40), 6463–6466 CrossRef CAS.
-
(a)
A. Heumann, in Transition metals for organic synthesis, ed. M. Beller and C. Bolm, Wiley-VCH, Weinheim, Germany, 2004, p. 251 Search PubMed;
(b) B. C. Ranu and R. Jana, Ionic liquid as catalyst and reaction medium: A simple, convenient and green procedure for the synthesis of thioethers, thioesters and dithianes using an inexpensive ionic liquid, [pmIm]Br, Adv. Synth. Catal., 2005, 347(14), 1811–1818 CrossRef CAS;
(c) S. Vijaikumar and K. Pitchumani, Simple, solvent free syntheses of unsymmetrical sulfides from thiols and alkyl halides using hydrotalcite clays, J. Mol. Catal. A: Chem., 2004, 217(1–2), 117–120 CrossRef CAS;
(d) R. N. Salvatore, R. A. Smith, A. K. Nischwitz and T. Gavin, A mild and highly convenient chemoselective alkylation of thiols using Cs2CO3-TBAI, Tetrahedron Lett., 2005, 46(51), 8931–8935 CrossRef CAS.
- B. M. Trost and T. R. Verhoeven, Allylic alkylation. Palladium-catalyzed substitutions of allylic carboxylates. stereo-and regiochemistry, J. Am. Chem. Soc., 1980, 102(14), 4730–4743 CrossRef CAS.
- A. W. Williamson, XXII.—On etherification, Q. J. Chem. Soc., 1852, 4(3), 229–239 RSC.
-
(a) Z. Sahli, N. Derrien, S. Pascal, B. Demerseman, T. Roisnel, F. Barriere, M. Achard and C. Bruneau, Preparation of chiral ruthenium(iv) complexes and applications in regio-and enantioselective allylation of phenols, Dalton Trans., 2011, 40(20), 5625–5630 RSC;
(b) J. A. van Rijn, E. van Stapele, E. Bouwman and E. Drent, Remarkable activity of the isomerization catalyst [RuCp(PPh3)2](OTs) in O-allylation of phenol with allyl alcohol, J. Catal., 2010, 272(2), 220–226 CrossRef CAS;
(c) M. Austeri, D. Linder and J. Lacour, (Cyclopentadienyl) ruthenium-catalyzed region- and enantioselective decarboxylative allylic etherification of allyl aryl and alkyl carbonates, Adv. Synth. Catal., 2010, 352(18), 3339–3347 CrossRef CAS.
- Y. Yatsumonji, Y. Ishida, A. Tsubouchi and T. Takeda, Nickel (0) triethyl phosphite complex-catalyzed allylic substitution with retention of regio-and stereochemistry, Org. Lett., 2007, 9(22), 4603–4606 CrossRef CAS.
-
(a) F. L. Lam, T. T.-L. Au-Yeung, F. Y. Kwong, Z. Zhou, K. Y. Wong and A. S. C. Chan, Palladium-(S, pR)-ferroNPS-catalyzed asymmetric allylic etherification: Electronic effect of nonconjugated substituents on benzylic alcohols on enantioselectivity, Angew. Chem., Int. Ed., 2008, 47(7), 1280–1283 CrossRef CAS;
(b) Y. Kayaki, T. Koda and T. Ikariya, Halide-free dehydrative allylation using allylic alcohols promoted by a palladium-triphenyl phosphite catalyst, J. Org. Chem., 2004, 69(7), 2595–2597 CrossRef CAS.
- H. Nakagawa, T. Hirabayashi, S. Sakaguchi and Y. Ishii, Allylation of alcohols and carboxylic acids with allyl acetate catalyzed by [Ir(cod)2]+ BF4− complex, J. Org. Chem., 2004, 69(10), 3474–3477 CrossRef CAS.
-
(a) A. Saha, J. Leazer and R. S. Varma,
O-allylation of phenols with allylic acetates in aqueous media using a magnetically separable catalytic system, Green Chem., 2012, 14(1), 67–71 RSC;
(b) R. B. N. Baig and R. S. Varma, Magnetic silica-supported palladium catalyst: Synthesis of allyl aryl ethers in water, Ind. Eng. Chem. Res., 2014, 53(49), 18625–18629 CrossRef.
- S. K. Ghatak, D. Dey, S. Sen and K. Sen, Aromatic amino acids in high selectivity bismuth(III) recognition, Analyst, 2013, 138(8), 2308–2314 RSC.
- C. M. Chan and L. T. Wang, Surface characterization of polymer blends by XPS and ToF-SIMS, Materials, 2016, 9(8), 655–673 CrossRef.
- Y. A. Mirgorod, V. G. Borodina and N. A. Borsch, Investigation of interaction between silver ions and rutin in water by physical methods, Biophysics, 2013, 58(6), 743–747 CrossRef CAS.
-
J. W. Mullin, Crystallization, Butterworth-Heinemann, Boston, 3rd edn, 1997, ISBN: 0-7506-3759-5 Search PubMed.
- M. Thommes, K. Kaneko, A. V. Neimark, J. P. Olivier, F. Rodriguez-Reinoso, J. Rouquerol and K. S. W. Sing, Physisorption of gases, with special reference to the evaluation of surface area and pore size distribution (IUPAC technical report), Pure Appl. Chem., 2015, 87(9–10), 1051–1069 CAS.
- M. Samsonowicz, I. Mamińska, M. Kalinowaska and W. Lewandowski, Alkali metal salts of rutin-synthesis, spectroscopic (FT-IR, FT-Raman, UV-VIS), Antioxidant and antimicrobial studies, Spectrochim. Acta, Part A, 2015, 151, 926–938 CrossRef CAS.
- A. Kaur, D. Goyal and R. Kumar, Surfactant mediated interaction of vancomycin with silver nanoparticles, Appl. Surf. Sci., 2018, 449, 23–30 CrossRef CAS.
- P. Wynblatt, A calculation of the surface energies of fcc transition metals, Surf. Sci. Lett., 1984, 136(2–3), L51–L56 CAS.
- G. Greczynski and L. Hultman, X-ray photoelectron spectroscopy: Towards reliable binding energy referencing, Prog. Mater. Sci., 2020, 107, 100591 CrossRef CAS.
- J. C. Bertolini, P. Delichere, B. C. Khanra, J. Massardier, C. Noupa and B. Tardy, Electronic properties of supported Pd aggregates in relation with their reactivity for 1, 3-butadiene hydrogenation, Catal. Lett., 1990, 6(2), 215–224 CrossRef CAS.
- M. C. Militello and S. J. Simko, Palladium oxide (PdO) by XPS. Surface science spectra, Surf. Sci. Spectra, 1994, 3(4), 395–401 CrossRef CAS.
- P. Ptáček and Z. Bastl, XPS Characterization of Supported Bimetallic Palladium–Silver Clusters, Appl. Surf. Sci., 1990, 45(4), 319–323 CrossRef.
- L. L. Wang and D. D. Johnson, Predicted trends of core–shell preferences for 132 late transition-metal binary-alloy nanoparticles, J. Am. Chem. Soc., 2009, 131(39), 14023–14029 CrossRef CAS.
- L. Samiee, M. D. Mobarake, R. Karami and M. Ayazi, Developing of ethylene glycol as a new reducing agent for preparation of Pd-Ag/PSS composite membrane for hydrogen separation, J. Petrol. Sci. Technol., 2012, 2(2), 25–32 Search PubMed.
- Y. Ma, J. Bansmann, T. Diemant and R. J. Behm, Formation, stability and CO adsorption properties of PdAg/Pd (1 1 1) surface alloys, Surf. Sci., 2009, 603(7), 1046–1054 CrossRef CAS.
- I. A. Abrikosov, W. Olovsson and B. Johansson, Valence-band hybridization and core level shifts in random Ag–Pd alloys, Phys. Rev. Lett., 2001, 87(17), 176403 CrossRef CAS.
- Z. Yi, X. Tan, G. Niu, X. Xu, X. Li, X. Ye, J. Luo, B. Luo, W. Wu, Y. Tang and Y. Yi, Facile preparation of dendritic Ag–Pd bimetallic nanostructures on the surface of Cu foil for application as a SERS-substrate, Appl. Surf. Sci., 2012, 258(14), 5429–5437 CrossRef CAS.
- L. J. Gerenser, Photoemission investigation of silver/poly(ethylene terephthalate) interfacial chemistry: The effect of oxygen-plasma treatment, J. Vac. Sci. Technol., A, 1990, 8(5), 3682–3691 CrossRef CAS.
- J. M. Tura, P. Regull, L. Victoria and M. D. de Castellar, XPS and IR (ATR) analysis of Pd oxide films obtained by electrochemical methods, Surf. Interface Anal., 1988, 11(8), 447–449 CrossRef CAS.
Footnote |
† Electronic supplementary information (ESI) available. See DOI: 10.1039/d0ma00596g |
|
This journal is © The Royal Society of Chemistry 2020 |