DOI:
10.1039/D0MA00187B
(Paper)
Mater. Adv., 2020,
1, 363-370
High-efficiency and stable photocatalytic hydrogen evolution of rhenium sulfide co-catalyst on Zn0.3Cd0.7S†
Received
9th April 2020
, Accepted 3rd May 2020
First published on 4th May 2020
Abstract
Photocatalytic hydrogen evolution is an attractive technology to solve the growing energy crisis. The development of advanced photocatalysts is an attractive but challenging issue. For the first time, we use inorganic ReS2 as a co-catalyst for Zn0.3Cd0.7S using a solvent thermal method, which enables highly efficient and recyclable H2 evolution. The Zn0.3Cd0.7S/ReS2 composite photocatalysts contain 4 wt% ReS2 co-catalysts, and Na2S–Na2SO3 is used as the sacrificial reagent. Compared with bare Zn0.3Cd0.7S, Zn0.3Cd0.7S/ReS2 corresponds to 101 fold enhancement with the highest photocatalytic H2 evolution rates of 92.45 mmol h−1 g−1. Furthermore, it still maintains high hydrogen production after a 30 hours cycle experiment. In this paper, the mechanism of photocatalytic enhancement is expounded by various experimental methods. In situ Fourier Transform Infrared (FT-IR) spectra and online mass spectrometry were used to discover the sources of hydrogen. The experimental results show that ReS2 is a promising joint catalyst for obtaining high-gloss catalytic hydrogen from water under visible light.
1. Introduction
Hydrogen is considered to be a clean energy source, which could solve problems in the future such as the scarcity of fossil resources. However, the use of renewable resources rather than fossil fuels to produce hydrogen is a fundamental technical challenge.1,2 Honda and Fujishima presented the concept of photoelectrochemical decomposition of water into H2 and O2, which converts solar energy into chemical energy and/or electrical energy.3,4 In recent years, using photocatalytic reactions of semiconductors to generate hydrogen from water has attracted considerable interest, and many semiconductor photocatalysts have been discovered including sulfides, nitrides, metals/metal oxides, and so on.5–8 Among them, metal sulfides and their composites are regarded as good candidates for photocatalytic H2 production because of their suitable band gap and catalytic functions. CdS is the most widely used metal sulfide photocatalytic material for photocatalytic hydrogen production. It has a band gap of 2.4 eV, absorbs visible light, and has a suitable redox potential. However, CdS particles are easily agglomerated into irregular large-sized particles, resulting in the reduction of specific surface area, with a high degree of recombination of photogenerated electrons and holes, and being prone to photocorrosion, which severely limits the photocatalytic efficiency. To solve the above problems, a variety of effective methods, such as element doping, construction of heterogeneous knots, or the use of co-catalysts, has been developed.9–11
Compared with CdS, ZnS has a better performance, but the band gap of ZnS is 3.6 eV, which allows it to absorb only the energy of ultraviolet light. In order to take full advantage of their respective advantages, CdS and ZnS are combined to form a ZnxCd1−xS solid solution as a high-efficiency catalyst for light.12 For example, Xing et al. synthesized a ZnxCd1−xS solid solution using a simple coprecipitation method and N2 atmosphere heat treatment.13 By adjusting the ratio of Zn to Cd, the forbidden bandwidth of the ZnxCd1−xS solid solution is between 2.20 eV and 3.12 eV. Although the ZnxCd1−xS solid solution exhibits higher photocatalytic activity than the one-component ZnS and CdS,14–17 these solid solutions still exhibit lower photocatalytic efficiency. Therefore, finding new solutions is necessary.
2D transition metal trihalides (TMDs), such as MoS2 and WS2, have large interlayer spaces and weak interlayer interactions, and have been widely studied as effective catalysts for hydrogen evolution.18,19 Recently, it is confirmed that a new type of TMD, rhenium disulfide (ReS2), has extraordinary photocatalytic properties due to its remarkable electron–hole separation and is a promising photocatalyst for hydrogen production.20–24 With this in mind, we expect ReS2 to act as a cocatalyst to further enhance the photocatalytic performance, which may provide new opportunities for photocatalytic H2 evolution.25–31 However, to the best of our knowledge, it has rarely been reported as a cocatalyst for photocatalytic hydrogen production.
In this work, ReS2 was successfully loaded onto ZnxCd1−xS nanorods using a simple two-step hydrothermal method. The ZnxCd1−xS nanorods provide a substrate for the loading of ReS2. The introduction of ReS2 enables the transfer of photogenerated electrons, inhibits carrier recombination and improves the stability of ZnxCd1−xS during a photocatalytic reaction. Therefore, ZnxCd1−xS with ReS2 as a cocatalyst exhibits higher hydrogen production performance and stability. The optimum performance is confirmed by adjusting the ratio of zinc/Cd and the amount of ReS2 loaded. These results reveal that the ReS2/Zn0.3Cd0.7S composite loaded with 4 wt% ReS2 exhibits the highest hydrogen production rate of 92.45 mmol h−1 g−1, and it has a stable hydrogen production rate for more than 30 hours when Na2S–Na2SO3 is used as the sacrificial reagent.
2. Experimental section
2.1 Materials preparation
Synthesis of ZnxCd1−xS nanorods.
In a typical synthesis of ZnxCd1−xS nanorods (x = 0, 0.1, 0.2, 0.3, 0.4, 0.5, 0.7 and 1), 2 mmol L-cysteine, zinc acetate and dihydrate cadmium acetate amounting to a total concentration of 1 mmol with various molar ratios were dissolved in 35 mL of a binary solution of ethanol amine. The reaction suspension was stirred vigorously for 0.5 h until a homogeneous dispersion was formed. Then, the solution was transferred into a Teflon-lined autoclave and maintained at 180 °C for 24 h. After the autoclave was cooled to room temperature, Zn0.3Cd0.7S nanorods were washed with distilled water and absolute ethanol several times, and then vacuum dried at 60 °C overnight.
Synthesis of 10% ReS2/Zn0.3Cd0.7S.
10.02 mg of NH4ReO4, 5.62 mg of thioacetamide (C2H5NS) and 400 mg of CdS were dissolved in 40 ml of H2O. The reaction suspension was stirred vigorously for 30 min until a homogeneous dispersion was formed. The reaction mixture was added to a Teflon-lined stainless steel autoclave and maintained at 220 °C for 48 h. After the autoclave was cooled to room temperature, 10% ReS2/Zn0.3Cd0.7S was washed with distilled water and absolute ethanol for four times, and then vacuum dried at 60 °C overnight.
2.2 Characterization
The phase and crystal structures of the as-prepared samples were characterized by X-ray diffraction (XRD) on a Bruker D8 diffractometer using Cu Kα (λ = 1.5406) radiation in the 2θ range from 5° to 70°. X-ray photoelectron spectroscopy (XPS) data were obtained using a Thermo ESCALAB 250XI X-ray photoelectron spectrometer (Al Kα, 150 W, C 1s 284.8 eV). The high-resolution transmission electron microscopy (HRTEM) images and element mapping were obtained using JEOL JEM-2100 F (UHR) field emission transmission electron microscopy. UV-vis diffuse reflectance spectra (DRS) of the samples were determined using a UV-vis spectrometer (PerkinElmer, Lambda 850; BaSO4 as a reference) and recorded in the range of 200–800 nm. Time-resolved photoluminescence (PL) spectra (380 nm excitation) were recorded using an FLS980 multifunctional steady-state and transient fluorescence spectrometer (Edinburgh Instruments, room temperature). The transient surface photovoltage data were recorded using a 500 MHz digital oscilloscope (TDS 3054C, Tektronix, Beaverton, OR, USA). An inductively coupled plasma (ICP) spectrometer (ICPE-9820, Shimadzu) was used to confirm the ionic concentration.
2.3 Photocatalytic H2 production
The photocatalytic hydrogen tests were conducted in a Labsolar-III AG closed gas circulation and exhaust system (Beijing Perfect Light Technology Co., Ltd, China) maintaining the photo-reaction temperature at 5 °C using a low-temperature thermostat bath (Poly Science, USA). In the photo-reaction system, 20 mg of ReS2/CdS was suspended in 50 mL of DI water containing 2.1 g of Na2S and 0.8 g of Na2SO3. A 300 W xenon lamp (PLS-SXE-300UV, Beijing Trusttech Co. Ltd, China) with a UV-cutoff filter (providing visible light λ ≥ 420 nm) served as the visible-light source to trigger the water splitting reactions. To identify and quantify the gases produced, a volume of 1.5 mL of gas was sampled hourly and measured using a gas chromatograph (GC9790II, Zhejiang Fuli Analytical Instrument Co., Ltd, China) equipped with a thermal conductivity detector (TCD) and a 5 Å molecular sieve column, where argon (Ar) was used as the carrier gas. The quantification of the H2 yield was based on a calibration curve.
2.4 Apparent quantum efficiency (AQE) calculations
The apparent quantum efficiency (AQE) was measured under the same photocatalytic reaction conditions, except for the wavelength of the incident light. For the H2 yields of 1 h photoreaction under monochromatic light, AQE was calculated by the following equation:
2.5 Photoelectrochemical measurements
Photocurrent response, linear sweep voltammetry and electrochemical impedance of the catalysts were measured using an electrochemical workstation (CHI 630e) in a three-electrode quartz reactor using 0.5 M Na2SO4 solution and a mixed solution of 0.1 M K3[Fe(CN)6] and K4[Fe(CN)6]·3H2O, respectively, as the electrolyte solutions. Linear sweep voltammetry (LSV) was carried out at a scan rate of 10 mV s−1 from 0.4 to −0.6 V. Working electrodes for samples were prepared by applying the doctor-blading method. Then, 0.01 g of ethyl cellulose was dissolved in 15 ml of ethanol with 0.1 g of catalyst. Subsequently, a glass stick was applied to FTO at a high-temperature using a layer of adhesive tape on the edge. Finally, it was dried in air and activated at 120 °C for 2 h. All tests were conducted under visible light irradiation.
2.6
In situ Fourier transform infrared spectrometer (FT-IR) analysis
A Fourier transform infrared spectrometer (Nicolet IS-50) was used for in situ FT-IR measurements. The sample was filled into the in situ IR cell, and Ar and H2O gases were introduced into the cell and a fibre source (FX300, Beijing Perfect light Technology Co., Ltd, China) through the CaF2 window of the cell. Before the measurement, the sample was degassed at 423 K for 4 h. The baseline was obtained after adsorption equilibrium on the sample for 1 h. 1% ReS2/Zn0.3Cd0.7S was used after treatment by Na2S–Na2SO3.
2.7 On-line mass spectrometry analysis
10 ml of H2O/D2O with 10 mg of photocatalyst and 2.1–0.8 g of Na2S–Na2SO3 was added to the closed quartz reactor (Beijing Perfect light Technology Co., Ltd, China). One end of the reactor was continuously fed with Ar and the other end was connected with the sampling port of the mass spectrometer (HPR-20 R&D, Beijing Hiden Analytical Technology Co., Ltd, China). H2 and D2 were detected simultaneously in the MID mode of the SEM detector. After the MS baseline was stable, 300 W high pressure xenon lamp (PLS-SXE300, Beijing Perfect light Technology Co., Ltd, China) was used to illuminate the gas products in the reactor on-line.
3. Results and discussions
Structures and compositions of ReS2/Zn0.3Cd0.7S (4 wt% of ReS2) are visualized in Fig. 1. Fig. 1a and b show the XRD patterns of CdS, ZnS, Zn0.3Cd0.7S, ReS2/Zn0.3Cd0.7S, and the standard diffraction patterns of ZnS (PDF #77-2100) and CdS (PDF #77-2306).32 Compared with the standard diffraction peaks of ZnS and CdS, the diffraction peaks of Zn0.3Cd0.7S were shifted towards the lower-angle and higher-angle side, respectively, indicating that the sample is not a compound of ZnS and CdS, but a Zn0.3Cd0.7S solid solution. After the loading of ReS2 on the surface of Zn0.3Cd0.7S, the diffraction peaks of Zn0.3Cd0.7S were not shifted and no new peaks appeared. This indicates that the loading of ReS2 cannot affect the crystal structure of Zn0.3Cd0.7S. In addition, the value of full width at half maximum of the solid-soluble is found to be very large, which can be attributed to the different thickness of the Zn0.3Cd0.7S and ReS2/Zn0.3Cd0.7S samples. The loading of ReS2 onto Zn0.3Cd0.7S did not significantly alter the nanorod morphology of Zn0.3Cd0.7S, but there were obvious interfaces formed between ReS2 and Zn0.3Cd0.7S, indicating that ReS2 is tightly bound to the Zn0.3Cd0.7S nanorod surface. This finding is further confirmed by transmission electron microscopy (TEM) and high-resolution TEM (HRTEM) of ReS2/Zn0.3Cd0.7S (Fig. 1c–g). HRTEM shows that the grid spacing of ReS2/Zn0.3Cd0.7S is 0.33 nm, which is consistent with the XRD test results (Fig. 1f). In addition, element mapping of ReS2/Zn0.3Cd0.7S corresponding to the area is marked in Fig. 1, confirming that elemental S is uniformly dispersed in the whole selected area, while Re, Cd and Zn are distributed separately, indicating that there is intimate contact between ReS2 and Zn0.3Cd0.7S. An effective interfacial contact can shorten the charge transfer distance and facilitate electron transfer between CdS and Zn0.3Cd0.7S. To further characterize the surface chemical composition and valence state, ReS2/Zn0.3Cd0.7S was analyzed by X-ray photoelectron spectroscopy (XPS). As shown in Fig. 1m and n, the Cd 1s and Zn 2p XPS spectra demonstrate the structural characteristics of Zn0.3Cd0.7S. Fig. 1o shows two distinct peaks at 41.4 and 43.8 eV, which can be assigned to the Re 4f7/2 and Re 4f5/2 states of Re4+ in ReS2,33 respectively. All the above results corroborate the successful deposition of ReS2 on the Zn0.3Cd0.7S surface.
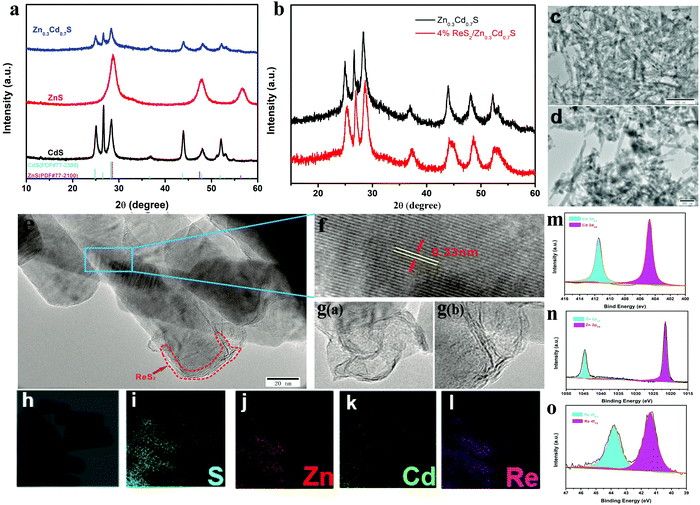 |
| Fig. 1 Composition and morphological characterizations of ReS2/Zn0.3Cd0.7S. (a and b) XRD pattern, (c) Zn0.3Cd0.7S TEM image, (d) ReS2/Zn0.3Cd0.7S TEM image, (e–g) HRTEM image, (f) Zn0.3Cd0.7S HRTEM image, (g) ReS2/Zn0.3Cd0.7S HRTEM image, (h–l) EDS elemental mapping, and (m–o) XPS analysis of ReS2/Zn0.3Cd0.7S. | |
The effect of ReS2 modification on the optical properties of Zn0.3Cd0.7S was studied by UV-vis diffusion reflection spectroscopy (Fig. 2a). After ReS2 was loaded, the light absorption of CdS and Zn0.3Cd0.7S in the visible light absorption area was significantly enhanced, while the light absorption of pure CdS and Zn0.3Cd0.7S was not very different, which indicates that the enhancement of visible light is due to the presence of ReS2. This may be attributed to the inherent high visible light capture capability of black ReS2 nanoparticles. According to the plot of (αhv)2versus energy (hv), the band gap value of ReS2/Zn0.3Cd0.7S was significantly reduced, which is more conductive to the excitation of visible light. Mott–Schottky plots (Fig. S5, ESI†) and XPS price band spectrum (Fig. S6, ESI†) also show that the price band of ReS2/Zn0.3Cd0.7S is more negative, which can facilitate a reduction reaction.
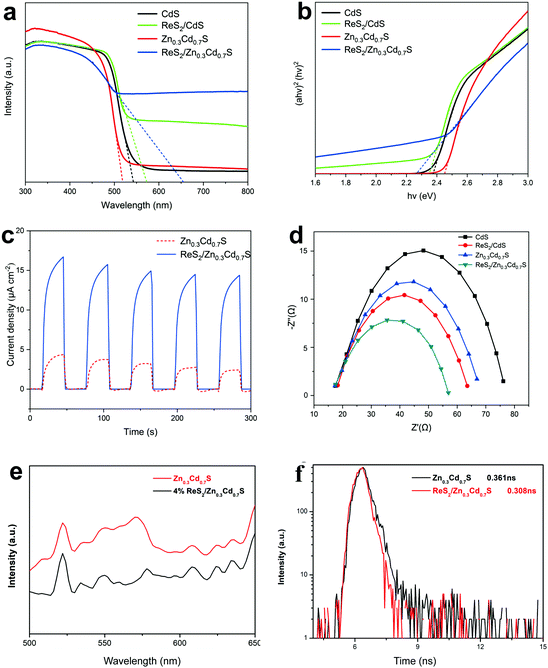 |
| Fig. 2 Optical properties and charge carrier dynamics of 4% ReS2/Zn0.3Cd0.7S and Zn0.3Cd0.7S. (a) UV-vis, (b) (αhν)2vs. photon energy (hν), (c) photocurrent responses, (d) electrochemical impedance plots, (e) PL spectra, and (f) time-resolved PL spectra. | |
To confirm and better understand the enhanced migration and separation of photo-induced charge carriers of ReS2/Zn0.3Cd0.7S, photoelectrochemical measurements were performed. As expected, ReS2/Zn0.3Cd0.7S showed a nearly 3 times higher photocurrent intensity than bare Zn0.3Cd0.7S, which indicates that ReS2 is an effective co-catalyst to promote the migration of photo-induced carriers (Fig. 2c). Meanwhile, the effect of ReS2 deposition on the kinetics of charge migration was further studied by electrochemical impedance spectroscopy (EIS). In comparison with pure CdS, ReS2/CdS, and Zn0.3Cd0.7S, the semicircle in the Nyquist plot of ReS2/Zn0.3Cd0.7S has a smaller radius, and it is indicative of accelerated charge transport and improved charge separation during photocatalysis, which demonstrates the prominent role of ReS2 nanoparticles as co-catalysts (Fig. 2d). Photoluminescence (PL) spectra (Fig. 2e) and time-resolved PL spectra (Fig. 2f) show that after the loading of ReS2 on Zn0.3Cd0.7S, more photocarriers are produced, and the lifetimes are 0.361 ns (Zn0.3Cd0.7S) and 0.308 ns (ReS2/Zn0.3Cd0.7S), indicating that the photogenerated carriers of ReS2/Zn0.3Cd0.7S are more rapidly separated, and thus, hydrogen can be produced through a redox reaction.
Due to the unique characteristics of ReS2 in promoting light absorption and charge separation, we studied the role of ReS2 as a catalyst loaded on ZnxCd1−xS for photocatalytic hydrogen production. Fig. 3a shows the production of hydrogen for photocatalysts of solid-solubles with different Zn–Cd ratios under the condition of visible light irradiation and Na2S–Na2SO3 as the sacrificial reagent. With the ratio of Zn and Cd in ZnxCd1−xS being changed, the test results for comparing photocatalytic hydrogen production are shown in Fig. 3a, and the optimal ratio product of Zn and Cd is Zn0.3Cd0.7S (0.91 mmol g−1 h−1). By adjusting the amount of loading of ReS2 (Fig. 3b), it can be found that the loading of 4 wt% ReS2 on Zn0.3Cd0.7S has the highest hydrogen production (92.45 mmol g−1 h−1), which is 101 times higher than that of pure Zn0.3Cd0.7S. This shows that ReS2 can greatly improve the photocatalytic hydrogen production of Zn0.3Cd0.7S. To understand the details of enhanced photocatalytic hydrogen evolution, the apparent quantum efficiency (AQY) of ReS2 (4 wt%)/Zn0.3Cd0.7S was calculated at different irradiated wavelengths, as shown in Fig. 3b. At wavelengths of 400, 420, and 500 nm, the AQY of ReS2 (4 wt%)/Zn0.3Cd0.7S is 23.24%, 20.95% and 0.88%, respectively. The above results show that ReS2 is a very promising candidate as a common catalyst for hydrogen evolution. To determine the photocorrosion properties of CdS, we performed 30 hours of circulatory tests of Zn0.3Cd0.7S and ReS2/Zn0.3Cd0.7S, as shown in Fig. 3c and d. A time period of five hours is considered as a group. The results show that ReS2/Zn0.3Cd0.7S maintains a high photocatalytic hydrogen production performance even after 30 hours of light irradiation, with no significant reduction in yield. The above results confirm that ReS2/Zn0.3Cd0.7S is a promising photocatalytic material with high photocatalytic activity and high cycling stability.
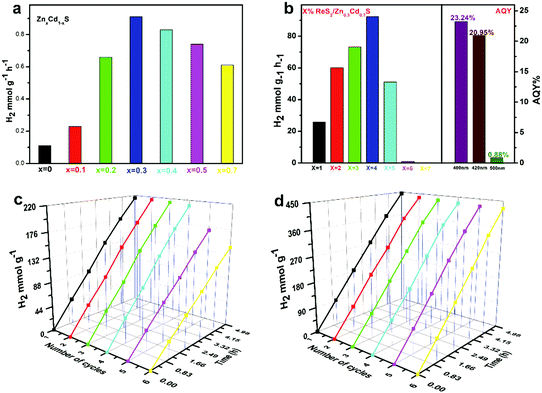 |
| Fig. 3 Photocatalytic H2 evolution on ReS2/Zn0.3Cd0.7S and Zn0.3Cd0.7S photocatalysts. (a) Hydrogen generation rate of ZnxCd1−xS under visible-light irradiation, (b) hydrogen generation rate of Zn0.3Cd0.7S with loading of ReS2 of different amounts under visible-light irradiation and apparent quantum efficiency under irradiation with monochromatic light at different wavelengths, (c) cycling tests of photocatalytic H2 evolution on Zn0.3Cd0.7S under visible-light irradiation, and (d) cycling tests of photocatalytic H2 evolution on ReS2/Zn0.3Cd0.7S under visible-light irradiation. | |
In the field of photocatalytic hydrogen production, sacrificial reagents are often used to improve the hydrogen production activity, but there may be hydrogen sources in these reagents. To confirm that water is indeed decomposed to hydrogen, isotope experiments were performed. When Na2S–Na2SO3 was used as the sacrificial reagent in H2O solution, only H2 was found and neither HD nor D2 was detected (Fig. 4a). In D2O solution, only D2 and no H2 or HD was detected (Fig. 4b). In addition, H2 and D2 were produced after light irradiation, and no signals of H2 and D2 were found under dark conditions, indicating that the hydrogen-producing reaction is performed under light-excited conditions and that the materials cannot produce hydrogen by themselves.
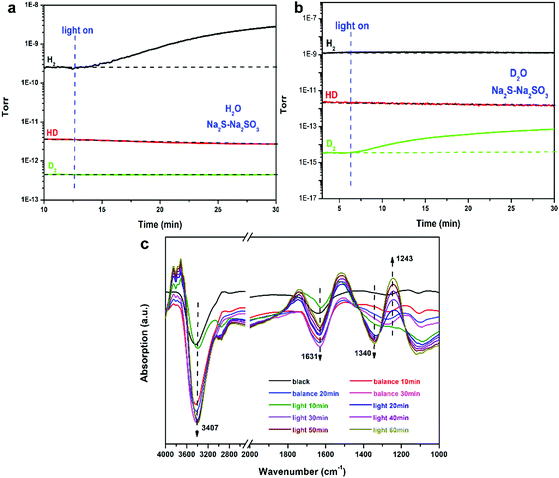 |
| Fig. 4
In situ FT-IR spectra and on-line mass spectrometry of photocatalytic hydrogen production on ReS2/Zn0.3Cd0.7S: (a) H2O solution of Na2S–Na2SO3; (b) D2O solution of Na2S–Na2SO3; (c) in situ FT-IR spectra of the H2O reaction on ReS2/Na2S–Na2SO3 with Na2S–Na2SO3 as the sacrificial reagent. | |
Fig. 4c shows the in situ FT-IR spectra of the H2O reaction on ReS2/Zn0.3Cd0.7S when Na2S–Na2SO3 was used as the sacrificial reagent. The spectrum shows a peak at 3407 cm−1 which can be attributed to the stretching vibration of adsorbed H2O molecules. The two peaks at 1631 and 1340 cm−1 can be assigned to the stretching vibration of adsorbed SO32−,34–37 and the peak at 1243 cm−1 can be assigned to the stretching vibration of SO42−. After illumination for 60 min, the IR peaks of H2O and SO32− decreased with no obvious position change, while the IR peaks of SO42− increased with the increasing time of light illumination. This result indicates that H2O decomposition occurs during the photocatalytic hydrogen evolution, meanwhile, the SO32− as the sacrificial reagent can be oxidized to SO42−. The above results are also confirmed by online mass spectrometry.
4. Conclusions
In summary, we successfully developed ReS2 as a highly efficient cocatalyst on Zn0.3Cd0.7S nanorods for extraordinarily efficient photocatalytic hydrogen evolution. The optimal photocatalytic hydrogen evolution rate of ReS2/Zn0.3Cd0.7S is 92.45 mmol h−1 g−1, and the best quantum efficiency is 23.24% at 400 nm. What's more, there was no significant decrease in the photocatalytic activity after the 30 hours cycle of photocatalytic reaction. The excellent catalytic activity is due to the interaction between Zn0.3Cd0.7S and ReS2 that inhibits the compound of photogenic runners. When the Zn elements are added to CdS, the photo-corrosive effect can be inhibited and they may synergize with the surface ReS2 to exhibit better photocatalytic properties. In addition, photocatalytic hydrogen evolution over ReS2/Zn0.3Cd0.7S has been comprehensively elucidated at the molecular level using on-line mass spectrometry, and in situ FT-IR. It is anticipated that this work will promote the development of hydrogen production systems with advanced co-catalysts and provide extremely high photocatalytic activities.
Conflicts of interest
There are no conflicts to declare.
Acknowledgements
This work was supported by the National Natural Science Foundation of China (No. 51872147), the 111 Project (D20015), and the Program for Innovative Research Team of Science and Technology in the University of Henan Province (19IRTSTHN025).
References
- H. Kisch, Semiconductor Photocatalysis—Mechanistic and Synthetic Aspects, Angew. Chem., Int. Ed., 2013, 52, 812–847 CrossRef CAS PubMed.
- J. Li, X. Gao, B. Liu, Q. Feng, X. B. Li, M. Y. Huang, Z. Liu, J. Zhang, C. H. Tung and L. Z. Wu, Graphdiyne: A Metal-Free Material as Hole Transfer Layer To Fabricate Quantum Dot-Sensitized Photocathodes for Hydrogen Production, J. Am. Chem. Soc., 2016, 138, 3954–3957 CrossRef CAS PubMed.
- K. Honda and A. Fujishima, Electrochemical Photolysis of Water at a Semiconductor Electrode, Nature, 1972, 238, 37–38 CrossRef PubMed.
- X. B. Chen, S. H. Shen, L. J. Guo and S. S. Mao, Semiconductor-based photocatalytic hydrogen generation, Chem. Rev., 2010, 110, 6503–6570 CrossRef CAS PubMed.
- X. Wang, K. Maeda, A. Thomas, K. Takanabe, G. Xin, J. M. Carlsson, K. Domen and M. Antonietti, A metal-free polymeric photocatalyst for hydrogen production from water under visible light, Nat. Mater., 2009, 8, 76–80 CrossRef CAS PubMed.
- L. Cheng, Q. Xiang, Y. Liao and H. Zhang, CdS-based photocatalysts, Energy Environ. Sci., 2018, 11, 1362–1391 RSC.
- Y. J. Yuan, Z. T. Yu, D. Q. Chen and Z. G. Zou, Metal-complex chromophores for solar hydrogen generation, Chem. Soc. Rev., 2017, 46, 603–631 RSC.
- L. Jing, W. Zhou, G. Tian and H. Fu, Surface tuning for oxide-based nanomaterials as efficient photocatalysts, Chem. Soc. Rev., 2013, 42, 9509–9549 RSC.
- A. Nag, S. Sapra, C. Nagamani, A. Sharma, N. Pradhan, S. V. Bhat and D. D. Sarma, A Study of Mn2+ Doping in CdS Nanocrystals, Chem. Mater., 2007, 19, 3252–3259 CrossRef CAS.
- N. J. Borys, M. J. Walter, J. Huang, D. V. Talapin and J. M. Lupton, The role of particle morphology in interfacial energy transfer in CdSe/CdS heterostructure nanocrystals, Science, 2010, 330, 1371–1374 CrossRef CAS PubMed.
- J. Yang, H. Yan, X. Wang, F. Wen, Z. Wang, D. Fan, J. Shi and C. Li, Roles of cocatalysts in Pt–PdS/CdS with exceptionally high quantum efficiency for photocatalytic hydrogen production, J. Catal., 2012, 290, 151–157 CrossRef CAS.
- W. Zhang and R. Xu, Surface engineered active photocatalysts without noble metals: CuS–ZnxCd1−xS nanospheres by one-step synthesis, Int. J. Hydrogen Energy, 2009, 34, 8495–8503 CrossRef CAS.
- C. Xing, Y. Zhang, W. Yan and L. Guo, Nano-CdS confined within titanate nanotubes for efficient photocatalytic hydrogen production under visible light illumination, Nanotechnology, 2019, 25, 035603 Search PubMed.
- C.-C. Chan, C.-C. Chang, C.-H. Hsu, Y.-C. Weng, K.-Y. Chen, H.-H. Lin, W.-C. Huang and S.-F. Cheng, Efficient and stable photocatalytic hydrogen production from water splitting over ZnxCd1−xS solid solutions under visible light irradiation, Int. J. Hydrogen Energy, 2014, 39, 1630–1639 CrossRef CAS.
- Y. Wang, J. Wu, J. Zheng, R. Jiang and R. Xu, Ni2+-doped ZnxCd1−xS photocatalysts from single-source precursors for efficient solar hydrogen production under visible light irradiation, Catal. Sci. Technol., 2011, 1, 940–947 RSC.
- J. D. Huang, J. Y. Liu and K. L. Han, Hybrid functionals studies of structural and electronic properties of ZnxCd1−xS and (ZnxCd1−x)(SexS1−x) solid solution photocatalysts, Int. J. Hydrogen Energy, 2012, 37, 17870–17881 CrossRef CAS.
- M. R. Gholipour, C. C. Nguyen, F. Béland and T. O. Do, Hollow microspheres consisting of uniform ZnxCd1−xS nanoparticles with noble-metal-free co-catalysts for hydrogen evolution with high quantum efficiency under visible light, J. Photochem. Photobiol., A, 2018, 358, 1–9 CrossRef CAS.
- X. Zong, H. Yan, G. Wu, G. Ma, F. Wen, L. Wang and C. Li, Enhancement of Photocatalytic H2 Evolution on CdS by Loading MoS2 as Cocatalyst under Visible Light Irradiation, J. Am. Chem. Soc., 2008, 130, 7176–7177 CrossRef CAS PubMed.
- K. Chang, X. Hai and J. Ye, Transition Metal Disulfides as Noble-Metal-Alternative Co-Catalysts for Solar Hydrogen Production, Adv. Energy Mater., 2016, 6, 1502555 CrossRef.
- Q. Zhang, W. Wang, J. Zhang, X. Zhu, Q. Zhang, Z. Zhang Ren, S. Song, J. Wang, Z. Ying, R. Wang, X. Qiu, T. Peng and L. Fu, Highly Efficient Photocatalytic Hydrogen Evolution by ReS2 via a Two-Electron Catalytic Reaction, Adv. Mater., 2018, 30, 1707123 CrossRef PubMed.
- Q. Zhang, W. Wang, J. Zhang, X. Zhu and L. Fu, Thermally Induced Bending of ReS2 Nanowalls, Adv. Mater., 2018, 30, 1704585 CrossRef PubMed.
- H. Liu, B. Xu, J. M. Liu, J. Yin, F. Miao, C. G. Duan and X. G. Wan, Highly efficient and ultrastable visible-light photocatalytic water splitting over ReS2, Phys. Chem. Chem. Phys., 2016, 18, 14222–14227 RSC.
- Q. Zhang, S. Tan, R. G. Mendes, Z. Sun, Y. Chen, X. Kong, Y. Xue, M. H. Rümmeli, X. Wu, S. Chen and L. Fu, Extremely Weak van der Waals Coupling in Vertical ReS2 Nanowalls for High-Current-Density Lithium-Ion Batteries, Adv. Mater., 2016, 28, 2616–2623 CrossRef CAS PubMed.
- M. Mao, C. Cui, M. Wu, M. Zhang, T. Gao, X. Fan, J. Chen, T. Wang, J. Ma and C. Wang, Flexible ReS2 nanosheets/N-doped carbon nanofibers-based paper as a universal anode for alkali (Li, Na, K) ion battery, Nano Energy, 2018, 45, 346–352 CrossRef CAS.
- H. Li, Z. Liang, Q. Deng, M. T. Hu, N. Du and W. Hou, Facile Construction of Defect-rich Rhenium Disulfide/Graphite Carbon Nitride Heterojunction via Electrostatic Assembly for Fast Charge Separation and Photoactivity Enhancement, ChemCatChem, 2019, 11, 1633–1642 CrossRef CAS.
- H. M. Liu, B. Xu, J. M. Liu, J. Yin, F. Miao, C. G. Duan and X. G. Wan, Highly efficient and ultrastable visible-light photocatalytic water splitting over ReS2, Phys. Chem. Chem. Phys., 2016, 18, 14222–14227 RSC.
- J. A. Song, R. R. Sun, Y. L. Chen, D. J. Sun and X. Y. Li,
L-Cysteine assisted synthesis of Zn0.5Cd0.5S solid solution with different morphology, crystal structure and performance for H2 evolution, Int. J. Hydrogen Energy, 2018, 43, 18220–18231 CrossRef CAS.
- Y. T. Lu, D. D. Wang, P. Yang, Y. K. Dua and C. Lu, Coupling ZnxCd1−xS nanoparticles with graphene-like MoS2: superior interfacial contact, low overpotential and enhanced photocatalytic activity under visible-light irradiation, Catal. Sci. Technol., 2014, 4, 2650–2657 RSC.
- H. T. Zhao, R. R. Sun, X. Y. Li and X. Sun, Enhanced photocatalytic activity for hydrogen evolution from water by Zn0.5Cd0.5S/WS2 heterostructure, Mater. Sci. Semicond. Process., 2017, 59, 68–75 CrossRef CAS.
- J. A. Song, Y. L. Chen, D. J. Sun and X. Y. Li, Perylenetetracarboxylic diimide modified Zn0.7Cd0.3S hybrid photocatalyst for efficient hydrogen production from water under visible light irradiation, Inorg. Chem. Commun., 2018, 92, 27–34 CrossRef CAS.
- Q. Li, H. Meng, J. G. Yu, W. Xiao, Y. Q. Zheng and J. Wang, Enhanced Photocatalytic Hydrogen-Production Performance of Graphene–ZnxCd1−xS Composites by Using an Organic S Source, Chem. – Eur. J., 2014, 20, 1176–1185 CrossRef CAS PubMed.
- J. Song, Q. Tian, J. Gao, H. Wu, Y. Chen and X. Li, Controlled preparation of CdS nanoparticle arrays in amphiphilic perylene tetracarboxylic diimides: organization, electrontransfer and semiconducting properties, CrystEngComm, 2014, 16, 1277–1286 RSC.
- C. M. Corbet, C. McClellan, A. Rai, S. S. Sonde, E. Tutuc and S. K. Banerjee, Field Effect Transistors with Current Saturation and Voltage Gain in Ultrathin ReS2, ACS Nano, 2015, 9, 363–370 CrossRef CAS PubMed.
- F. Li, Q. Gu, Y. Niu, R. Wang, Y. Tong, S. Zhu, H. Zhang, Z. Zhang and X. Wang, Hydrogen evolution from aqueous-phase photocatalytic reforming of ethylene glycol over Pt/TiO2 catalysts: role of Pt and product distribution, Appl. Surf. Sci., 2017, 391, 251–258 CrossRef CAS.
- H. A. Al-Abadleh and V. H. Grassian, FT-IR study of water adsorption on aluminum oxide surfaces, Langmuir, 2003, 19, 341–347 CrossRef CAS.
- E. Yoda, FT-IR Study of Dissociative Water Adsorption on 1-Butyl-3-methylimidazolium Exchanged Mordenite Zeolite, J. Phys. Chem. C, 2009, 113, 9851–9856 CrossRef CAS.
- L. Li, R. Bennett, W. Conway, H. Yu, S. Clifford, M. Maeder and G. Puxty, Development and Evaluation of a Novel Method for Determining Absorbent Composition in Aqueous Ammonia-Based CO2 and SO32− and SO42− Loaded Capture Process Solutions via FT-IR Spectroscopy, Energy Fuels, 2018, 32, 8563–8570 CrossRef CAS.
Footnote |
† Electronic supplementary information (ESI) available. See DOI: 10.1039/d0ma00187b |
|
This journal is © The Royal Society of Chemistry 2020 |