DOI:
10.1039/D0FO02704A
(Paper)
Food Funct., 2021,
12, 241-251
Low-dose alcohol ameliorated high fat diet-induced anxiety-related behavior via enhancing adiponectin expression and activating the Nrf2 pathway
Received
16th October 2020
, Accepted 23rd November 2020
First published on 24th November 2020
Abstract
Long-term high-fat-diet (HFD)-induced obesity is associated with many comorbidities, such as cognitive impairment and anxiety, which are increasing public health burdens that have gained prevalence in adolescents. Although low-dose alcohol could attenuate the risk of cardiovascular disease, its mechanism on HFD-induced anxiety-related behavior remains not clear. The mice were divided into 4 groups, Control (Con), Alcohol (Alc), HFD and HFD + Alc groups. To verify the effects of low-dose alcohol on HFD-induced anxiety-related behavior, the mice were fed with HFD for 16 weeks. At the beginning of week 13, the HFD-fed mice were administered intragastrically with low-dose alcohol (0.8 g kg−1) for 4 weeks. After 4 weeks of oral administration, low-dose alcohol decreased body weight and Lee's index in HFD-induced obese mice. Moreover, low-dose alcohol alleviated the anxiety-related behaviors of obese mice in the open field test and the elevated plus maze test. The HFD-induced damage to the hippocampus was improved in hematoxylin–eosin staining assay in mice. In addition, low-dose alcohol also suppressed HFD-induced oxidative stress and increased HFD-suppressed adiponectin (APN) expression and nuclear factor erythroid 2-related factor 2 (Nrf2) activation in the hippocampus. Taken together, low-dose alcohol significantly ameliorates HFD-induced obesity, oxidative stress and anxiety-related behavior in mice, which might be related to APN upregulation, Nrf2 activation and related antioxidase expression including SOD1, HO-1, and catalase.
1. Introduction
Human obesity is usually comorbid with physiological alterations in the brain regions that mediate affective processes.1 Obesity is considered a complex condition that can initiate the development of many comorbidities and psychosocial problems like depression and anxiety. With the increase of the global obesity epidemic, examination of how diet and weight gain affect neural function in cognition, motivation and emotion has become the forefront of research. There is a strong association between these emotional disorders and metabolic syndrome.2,3 Consumption of sugary and fatty foods is sufficient to alter the neural systems involved in cognition and anxiety including the hippocampus, nucleus accumbens, prefrontal cortex and amygdala in ways that are expected to promote poor mental health.4 Cumulative evidence showed that a diet rich in fatty foods decreased hippocampus volume, impaired cognitive function including memory and learning in humans, and more importantly, increased the vulnerability to depression and anxiety.5–7 Further epidemiological statistics showed that anxiety symptoms might be more common than depressive symptoms in obese people.8 However, the underlying biological mechanisms about anxiety disorders in obese individuals remain to be established.
Alcohol consumption is a practice that prevails in society.9 Moderate alcohol drinking could reduce the risk of many diseases, including endocrine diseases and multiple cardiovascular disorders.10,11 Moreover, the relationship between alcohol use and mood was often characterized as a J-shaped curve, such that moderate drinkers tended to report better subjective well-being and fewer depressive symptoms than alcohol abstainers and excessive drinkers.12 In our previous study, we found that low-dose alcohol could ameliorate homocysteine-induced anxiety-related behavior which might be related to attenuating oxidative stress.13 However, the role of low-dose alcohol on HFD-induced anxiety-related behavior has been rarely elucidated.
Abundant studies supported the fact that low-dose alcohol consumption increased plasma adiponectin (APN) levels and improved insulin sensitivity in relatively insulin-resistant individuals, an effect that might be mediated through alcohol-induced increases in the APN level.14–16 APN is a pleiotropic endogenous adipokine secreted from adipose tissue. APN is involved in regulating glucose levels as well as fatty acid oxidation.17 In the clinic, a decline in the APN level had been observed in obese patients. APN had been associated with various metabolism-associated disorders, such as diabetes and neurodegenerative diseases.18,19 Furthermore, researchers found that the APN treatment produced antidepressant- and anxiolytic-like behavioral effects in normal-weight mice and in diet-induced obese diabetic mice, which might be related to the nuclear receptor peroxisome proliferator-activated receptor gamma.3,20 Concurrently, mounting studies demonstrated that APN participated in reducing the level of oxidative stress by activating the nuclear factor erythroid 2-related factor 2 (Nrf2) to facilitate antioxidase induction.21,22 The Nrf2 transcriptional pathway played an important role in the regulation of genes that control the expression of proteins that are critical in the detoxication and elimination of reactive oxygen species (ROS).23 According to these, we hypothesize that low-dose alcohol, regulating the APN level and activating Nrf2 signaling pathway, may play a protective role in the brain tissue of obese animals. To our knowledge, low-dose alcohol treatment has not been tested in HFD-induced anxiety-related behavior.
Thus, to better understand the mechanisms underlying the protective role of alcohol on anxiety-related behavior induced by HFD, herein we investigated whether alcohol protected hippocampal tissue from HFD-induced oxidative stress through increasing the APN level and further delineated potential mechanisms implicated.
2. Materials and methods
2.1 Animal experiments
Male C57BL/6 mice at 4 weeks of age were bought from the Experimental Animal Center of the Xi'an Jiaotong University (Xi'an, China). The animals received food and autoclaved water in a temperature-controlled (24 °C) facility with a 12 h light/12 h dark cycle for 1 week prior to initiating the experiments. The animals were randomly divided into four different groups: (1) control group (Con, n = 8), (2) alcohol group (Alc, n = 8), (3) HFD group (HFD, n = 8), and (4) HFD plus Alc group (HFD + Alc, n = 8). The control diet (10% calories from fat, D12450B) and HFD (60% calories from fat, D12492) were purchased from Research Diets Inc. (New Brunswick, NJ, USA). For the dose of alcohol according to our previous experiment and other research about alcohol consumption protecting against brain injury,13,24 the animals were administered alcohol at a dose of 0.8 g per kg body weight. At the beginning of the 13th week, the mice were administered with 10% (v/v) alcohol or water by intragastric administration (i.g.) at 8 o'clock each day for 4 weeks. The whole experiment was performed continually for 16 weeks. The summary of the experimental design is shown in Fig. 1. When the test was finished, all the mice were anesthetized to collect blood samples from the orbital socket and isolate the perirenal fat and the hippocampus tissues. All animal procedures were performed in accordance with the Guidelines for the Care and Use of Laboratory Animals of Xi'an Jiaotong University and approved by the Animal Ethics Committee of Xi'an Jiaotong University.
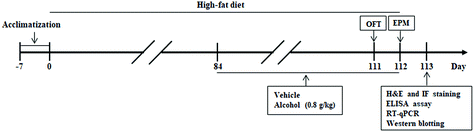 |
| Fig. 1 Experimental design and schedule for evaluating the effects of alcohol in HFD-fed mice. After 7 days of acclimatization, mice were fed high-fat diet for 112 days. Alcohol or vehicle was administered (i.g.) once a day for 28 days (from week 13 to week 16). An open field test (OFT) was performed on day 111, elevated plus maze test (EPM) was carried out on day 112, and the animals were subsequently killed. Hematoxylin–eosin (H&E) and immunofluorescence (IF) staining, enzyme-linked immunosorbent assay (ELISA), and biochemical assays were performed after behavioral tests. OFT, open field test; EPM, elevated plus maze test; RT-qPCR, Real-Time Quantitative PCR. | |
2.2 Open field test (OFT)
The open field test is a widely used test for assessing anxiety-related behavior. Experimental protocols were carried out as described in our previous research.13 One hour after administering alcohol or water, the mouse was placed at the center of the open field. The behavioral parameters were recorded for 5 minutes for each animal by use of a behavioral tracking analysis system (Chengdu Techman Software Co. Ltd, Sichuan, China). After each trial, the maze was cleaned in order to remove permeated odors from previous animals.
2.3 Elevated plus maze test (EPM)
The elevated plus maze test is typically used for evaluating anxiety-related behavior in rodents. Experimental protocols were carried out as described in our previous research.13 One hour after administering alcohol or water, the mouse was placed at the center of the elevated plus maze. The behavioral parameters were recorded for 5 minutes for each animal by use of a behavioral tracking analysis system (Chengdu Techman Software Co. Ltd, Sichuan, China). After each trial, the surface of the elevated plus maze was cleaned in order to remove permeated odors from previous animals.
2.4 Obesity parameters
During the whole experiment, body weights were measured weekly. On the first day of the 1st week and the last days of the 12th and 16th weeks, the body lengths (nose to anus) were measured. To determine the body mass index of mice, we calculated Lee's index with the formula [one-third of body weight (grams)/nasal–anal length (centimeters) × 1000. When the test was finished, perirenal adipose tissues were carefully removed and weighed.
2.5 Enzyme-linked immunosorbent assay (ELISA) for serum APN level
The serum level of APN was determined using an enzyme-linked immunosorbent assay kit according to the manufacturer's instructions (Nanjing Jiancheng Bioengineering Institute, Nanjing, China).
2.6 Hematoxylin–Eosin (H&E) staining
The H&E staining assay was used to visually observe the pathological changes of the tissue in mice. The brains and perirenal adipose tissue were removed, fixed with 4% buffered paraformaldehyde, dehydrated in the ascending series of ethanol and embedded in paraffin. Serial paraffin sections (6 μm) were stained for H&E. The pathological changes were assessed by light microscopy. Three mice in each group were used for H&E staining.
2.7 Immunofluorescence assay
To explore the effects of alcohol on Nrf2 expression in obese mice, paraffin sections, containing the hippocampus, were prepared for immunofluorescence staining using a Leica radial microtome and mounted on triethoxysilane-coated glass microscope slides. After deparaffinization, the sections were immersed in 10 mM citrate buffer (pH 6.0) and heated at 95 °C for 20 min to achieve antigen retrieval. After washing with PBS for 5 min
×
2 times, the sections were blocked in 5% bovine serum albumin (BSA) at room temperature for 20 min. Then the samples were incubated overnight with an anti-Nrf2 antibody (1
:
500 in dilution) at 4 °C. Subsequently, they were incubated with a TRITC-conjugated goat anti-rabbit IgG at a 1
:
200 dilution and an Alexa Fluor 488-labeled goat anti-mouse IgG (P0188, Beyotime, Shanghai, China) for 2 h at room temperature, and then counterstained using DAPI (5 μg ml−1) for 10 min. The images were observed using fluorescence microscopy (Leica, Germany Q9).
2.8 Parameters of oxidative stress quantification
The hippocampus tissues isolated from C57BL/6 mice were homogenized and centrifuged at 14
000 rpm for 15 min at 4 °C. Supernatants obtained by this procedure were utilized for the evaluation of oxidative stress parameters including SOD and catalase activity, and MDA and H2O2 concentrations according to the manufacturer's instructions (Nanjing Jiancheng Bioengineering Institute, Nanjing, China).
2.9 Western blotting
The hippocampus tissues were homogenized in ice-cold RIPA buffer containing the Halt protease inhibitor cocktail (Roche, Basel, Switzerland). Lysates were clarified by centrifugation at 12
000 rpm for 10 min at 4 °C. All steps were carried out on ice. The protein concentration of the supernatants was determined using a bicinchoninic acid protein assay kit according to the manufacturer's instructions (Nanjing Jiancheng Bioengineering Institute, Nanjing, China). Protein (20 μg) from each sample was separated by 8%–12% SDS-PAGE and electrotransferred to polyvinylidene fluoride membranes. The membranes were blocked with 5% non-fat milk for 1 h at room temperature and incubated overnight at 4 °C with primary antibodies including rabbit polyclonal anti-APN (ABP50587, 1
:
1000) antibody, rabbit anti-Nrf2 (CY5136, 1
:
1000) antibody, rabbit polyclonal anti-Keap1 (SAB32450, 1
:
1000) antibody, rabbit anti-heme oxygenase-1 (HO-1, CY5113, 1
:
1000) antibody, rabbit polyclonal anti-SOD1 (SAB32058, 1
:
1000) antibody, rabbit polyclonal anti-catalase (CY6783, 1
:
800) antibody, rabbit polyclonal anti-3-nitrotyrosine (3-NT, bs8551R, 1
:
1000) antibody and mouse monoclonal anti-GAPDH (AT0098, 1
:
5000) antibody. After being washed thrice with Tris-buffered saline with Tween 20, the membranes were incubated with corresponding horseradish peroxidase-conjugated secondary antibodies at room temperature for 2 h. The bands were visualized using an electrochemiluminescence system, and the band density was determined by ImageJ software (NIH, USA).
2.10 Real-time quantitative PCR
Total RNA was isolated from mice hippocampus tissue using Trizol (Invitrogen, San Diego, CA, USA). Four micrograms of RNA were reverse transcribed using a RevertAid FirstStand cDNA Synthesis Kit (ThermoScientific, Waltham, MA, USA) according to the manufacturer's protocol. Quantitative real-time PCR was performed using an SYBR Premix Pro Taq HS qPCR kit (Accurate Biotechnology Co., Ltd, China). The PCR reactions were performed in 96-well plates in an iQ5 Real-Time PCR Detection System (Bio-Rad Laboratories, Hercules, CA). GAPDH served as an endogenous control, and the expression levels of the other tested genes were normalized to the level of GAPDH. The PCR conditions were as follows: initial denaturation: 95 °C, 5 min; 40 cycles of denaturation (95 °C, 30 s), annealing (56 °C, 30 s), and elongation (72 °C, 60 s). The standard curve was produced and the data were analyzed using the Bio-Rad iQ5 software (Bio-Rad Laboratories, Hercules, CA). The fluorescent signals were collected during the extension phase, Ct values of the sample were calculated, and the mRNA levels were analyzed by the 2−ΔΔCt method.
The primers were designed using the sequence information of the NCBI database. Primer sequences are listed in Table 1.
Table 1 Primers used for real-time quantitative PCR
Primers |
Forward primer 5′-3′ |
Reverse primer 5′-3′ |
APN |
TCAGTGGATCTGACGACACC |
TGCCATCCAACCTGCACAA |
Nrf2 |
CTCCGTGGAGTCTTCCATTTAC |
TTAGGGCCGTTCTGTTTGAC |
Keap1 |
CCAGCTGCCCAATTCATGGC |
CATGCCCTGCTCCCGAAG |
SOD1 |
CTCAGGAGAGCATTCCATCATT |
CTCCCAGCATTTCCAGTCTT |
HO-1 |
GTACACATCCAAGCCGAGAA |
TGGTACAAGGAAGCCATCAC |
Catalase |
AGGCGGGAACCCAATAGGA |
CTGTCAAAGTGTGCCATCTCGT |
GAPDH |
ACCACAGTCCATGCCATCAC |
TCCACCACCCTGTTGCTGTA |
2.11 Statistical analysis
The results were expressed as mean ± SEM. The data were initially submitted for Levene's test for homogeneity of variance. Data were analyzed using one-way ANOVA followed by Fisher's least significant difference test (LSD-t). A value of P < 0.05 was defined as statistically significant. All data were analyzed with the SPSS version 21.0 (IBM SPSS Statistics 21).
3. Results
3.1 Effects of alcohol on obesity parameters in mice
After 12 weeks of HFD, body weight was significantly heavier (Fig. 2A, p < 0.05) in the HFD group than in the Con group. Lee's index was also obviously higher (Fig. 2B, p < 0.05) in the HFD-fed mice. At the end of the experiment, alcohol treatment decreased body weight (Fig. 2A, p < 0.05) and Lee's index (Fig. 2B, p < 0.05) clearly in the HFD-fed mice without influencing the change of body weight and Lee's index in the Alc group. In addition, HFD greatly increased the ratio of the weight of perirenal fat to body weight (Fig. 2C, p < 0.05), and histological examination showed that HFD enlarged the adipocyte size of perirenal fat (Fig. 2D). There was no statistical difference regarding the ratio of perirenal fat weight to body weight between the HFD group and the HFD + Alc group (Fig. 2C).
 |
| Fig. 2 Effects of alcohol on obesity parameters in mice. (A) Change curve of body weight; (B) Lee's index (the formula [one-third of body weight (grams)/nasal–anal length (centimeters) × 1000); (C) ratio of perirenal fat weight to body weight; (D) representative H&E staining images of perirenal fat. Scale bar, 20 μm. Each column represents the mean ± SEM, eight mice per group, * P < 0.05, vs. Con group; # P < 0.05, vs. HFD group. | |
3.2 Alcohol ameliorated anxiety-related behavior induced with HFD in mice
In the present study, the aim was to evaluate whether alcohol (0.8 g kg−1, i.g.) regulated HFD-induced anxiety-related behavior in mice. Results from the OFT showed that regardless of the reagents treated, the movement time was not affected in all groups (Fig. 3A and B, p > 0.05). In the OFT, long-term HFD decreased the time spent in the center zone (Fig. 3A and C, p < 0.05) and the number of rearing times (Fig. 3A and D, p < 0.05), whereas these parameters increased significantly in the Alc group. Meanwhile, the mice manifested increased time spent in the center zone (Fig. 3A and C, p < 0.05) and rearing times (Fig. 3A and C, p < 0.05) in the HFD + Alc group compared to the HFD group. In the EPM, no alterations were observed in the number of entries into the closed arms in all groups (Fig. 3E and F, p > 0.05). The results showed that long-term HFD obviously decreased the percentage of time spent in the open arms (Fig. 3E and G, p < 0.05) and percentage of entries into the open arms (Fig. 3E and H, p < 0.05), whereas these parameters were increased in the Alc group. In addition, the oral administration of alcohol notably increased the percentage of time spent in the open arm (Fig. 3E and G, p < 0.05) and the percentage of entries into the open arms (Fig. 3E and H, p < 0.05) in HFD-induced obese mice. The above results unfolded that low-dose alcohol had an anti-anxiety effect on the HFD-induced anxiety-related behavior in mice.
 |
| Fig. 3 Effects of alcohol on anxiety-related behavior in the open field (OFT) and elevated plus maze (EPM) tests. (A) Representative thermal images of mice motion trajectory in the OFT. The redder the trajectory color, the more frequently the mice travelled there. The red square represents the center zone. (B) Total movement time in the OFT. (C) Time spent in the center zone in the OFT; (D) number of rearing in the OFT. (E) Representative thermal images of mice motion trajectory in the EPM. The red rectangle represents the open arms. The redder the trajectory color, the more frequently the mice travelled there. (F) Number of entries into the closed arms in the EPM. (G) Percent of time spent in the open arms in the EPM. (H) Percent of entries into the open arms in the EPM. Each column represents the mean ± SEM, eight mice per group. *P < 0.05, vs. Con group; #P < 0.05, vs. HFD group. | |
3.3 Alcohol attenuated oxidative stress status in obese mice
The changes in the anxiety-related behavior of mice may be associated with damage to the hippocampus.25 To determine the above hypothesis, the histological changes of the hippocampus in mice were observed. In the Con group and Alc group, the hippocampal neurons were round, with clear and intact structure and good arrangement in the hippocampal CA1 (Fig. 4B) and CA3 regions (Fig. 4A). In contrast, long-term HFD contributed to the nuclei deeply stained in the HFD group mice, thereby indicating pyknosis and irregular arrangement, especially in the CA1 region. After administering alcohol to the HFD-fed mice, the deeply stained cells decreased obviously compared with those in the HFD group. These results illustrated that alcohol might prevent damage in the hippocampal cells induced by long-term HFD.
 |
| Fig. 4 Effects of alcohol on the level of oxidative stress induced by HFD in the hippocampus. (A) CA1 region; (B) CA3 region. Scale bar, 20 μm. Obvious changes, such as deep staining and pyknosis, are marked with red arrows. (C) SOD activity (n = 6); (D) catalase activity (n = 6); (E) MDA concentration (n = 6); (F) H2O2 concentration (n = 6); (G) NO concentration (n = 6); (H) protein level of 3-NT were analyzed using western blotting. Representative results of four independent experiments are shown. Each column represents the mean ± SEM. *P < 0.05, vs. Con group. #P < 0.05, vs. HFD group. | |
Next, due to the long-term obesity causing severe oxidative stress,26 we speculated that oxidative stress might be involved in the process of hippocampal changes. So, the level of oxidative stress in the hippocampus was determined by relative analysis. The results are shown in Fig. 4. Long-term HFD decreased SOD (Fig. 4C, p < 0.05) and catalase (Fig. 4D, p < 0.05) activity, and increased evidently MDA (Fig. 4E, p < 0.05) and H2O2 (Fig. 4F, p < 0.05) concentration correspondingly in hippocampi tissues. The results illustrated that alcohol increased SOD (Fig. 4C, p < 0.05) and catalase activity (Fig. 4D, p < 0.05) and reduced MDA concentration (Fig. 4E, p < 0.05) but did not affect H2O2 concentration (Fig. 4F, p > 0.05) in the Alc group compared to the Con group. Moreover, data further demonstrated that SOD (Fig. 4C, p < 0.05) and catalase activity (Fig. 4D, p < 0.05) enhanced distinctly and MDA (Fig. 4E, p < 0.05) and H2O2 concentration (Fig. 4F, p < 0.05) decreased in the HFD + Alc group compared with those in the HFD group. No alterations were observed in the level of NO in all mice (Fig. 4G, p > 0.05). However, the protein level of 3-NT increased obviously (Fig. 4H, p < 0.05) in the HFD group compared to the Con group. Importantly, alcohol decreased 3-NT protein level (Fig. 4H, p < 0.05) in HFD-fed mice. These data showed that alcohol had a benign effect in regulating oxidative stress and nitrosative stress induced by long-term HFD in mice.
3.4 Alcohol enhanced APN expression in obese mice
Clinical data suggested that alcohol changed the APN level in humans; whether alcohol reduced the APN level in obese mice needed to be verified. To elucidate the signaling mechanisms underlying the effects of alcohol, we measured the expression of APN genes and proteins involved in hippocampus. First, the mRNA (Fig. 5A, p < 0.05) and protein (Fig. 5B, p < 0.05) levels of APN in the hippocampus were dramatically downregulated in the HFD group compared to the Con group. Furthermore, the APN level in the serum was decreased (Fig. 5C, p < 0.05) after 16 weeks of HFD compared to the Con group. In contrast, alcohol treatment could enhance the mRNA (Fig. 5A, p < 0.05) and protein (Fig. 5B, p < 0.05) expressions of APN and the serum level of APN (Fig. 5C, p < 0.05) in hippocampus in the HFD + Alc group compared to the HFD group, consistent with previous reports.27
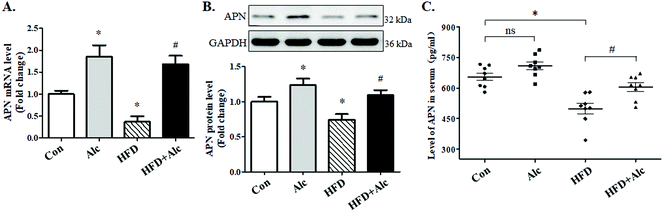 |
| Fig. 5 Effects of alcohol on the expression of APN in mice. (A) Total RNA was extracted and subjected to RT-qPCR for the assessment of APN mRNA level (n = 4); (B) protein level of APN in the hippocampus (n = 4); (C) APN level in serum (n = 6). The bar graph shows the mRNA level of APN after normalization to GAPDH. Each column represents the mean ± SEM. *P < 0.05, vs. Con group. #P < 0.05, vs. HFD group. | |
3.5 Alcohol activated Nrf2/Keap1 pathway in obese mice
Next, whether the Nrf2/Keap1 signaling pathway was involved in the effect of alcohol treatment on oxidative stress was examined. Long-term HFD downregulated evidently Nrf2 mRNA (Fig. 6A, p < 0.05) and protein (Fig. 6B, p < 0.05) levels compared to the Con group. Similarly, the mRNA (Fig. 6C, p < 0.05) and protein (Fig. 6D, p < 0.05) levels of Keap1, which targets Nrf2 for proteasomal degradation,28 increased significantly in the HFD group compared to the Con group. Nevertheless, alcohol treatment obviously upregulated the mRNA (Fig. 6A, p < 0.05) and protein (Fig. 6B, p < 0.05) levels of Nrf2 and decreased the mRNA (Fig. 6C, p < 0.05) and protein (Fig. 6D, p < 0.05) levels of Keap1 in HFD-induced obese mice. Besides, the immunofluorescence results of Nrf2 in hippocampus showed that long-term HFD reduced the reactivity of Nrf2 in the HFD group, while alcohol treatment significantly enhanced the expression levels of Nrf2 in HFD-induced obese mice (Fig. 6E). Further results indicated that hippocampal HO-1 mRNA (Fig. 6F, p < 0.05) and protein (Fig. 6G, p < 0.05) levels, SOD1 mRNA (Fig. 6H, p < 0.05) and protein (Fig. 6I, p < 0.05) levels, and catalase mRNA (Fig. 6J, p < 0.05) and protein (Fig. 6K, p < 0.05) levels were significantly decreased in the HFD group compared to the Con group, while alcohol treatment could enhance the above antioxidase expression in HFD-fed mice.
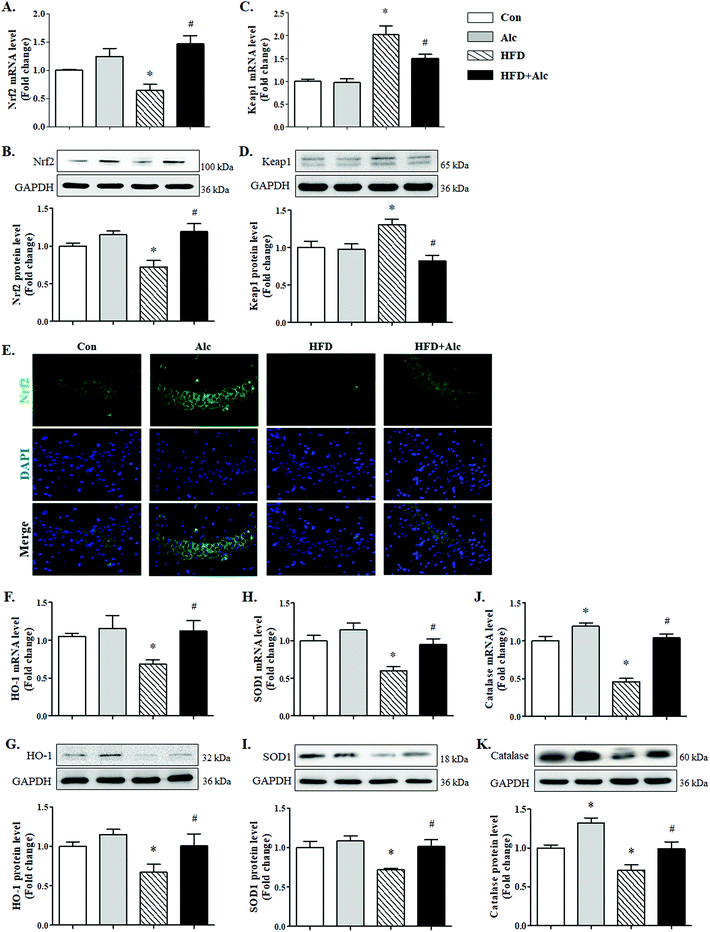 |
| Fig. 6 Effects of alcohol on the Nrf2/Keap1 pathway in the hippocampus. (A) Protein and (B) mRNA level of Nrf2; (C) protein and (D) mRNA level of Keap1; (E) representative immunofluorescence images of Nrf2 performed in the hippocampus CA3 region; (F) protein and (G) mRNA level of HO-1; (H) protein and (I) mRNA level of SOD1; and (J) protein and (K) mRNA level of catalase. Each column represents the mean ± SEM, four mice per group. *P < 0.05, vs. Con group. #P < 0.05, vs. HFD group. | |
4. Discussion
In the current study, we demonstrated that alcohol treatment ameliorated anxiety-related behavior in HFD-induced obese mice, through concomitantly promoting APN expression, activating Nrf2, upregulating hippocampal antioxidases (SOD1, HO-1 and catalase), and attenuating the damage of oxidative stress.
In modern society, overweight children are predisposed to social and emotional complications tied to overnutrition including depression, low self-esteem, and learning problems. In addition, controversy exists as to whether the overweight/obese phenotype was a root cause of childhood social and emotional problems or a sequela of resultant bullying. In juvenile animals, however, long-term HFD impacted working through pathways tied to glucocorticoids, neurogenesis and oxidative stress,29–31 and it was found that obesity impacted anxiety in adults.32 We aimed that five-week-old mice were randomly assigned to receive free access to a long-term HFD and elicited anxiogenic-like behavior after prolonged exposure to an HFD. In line with clinical studies, obesity is associated with increased susceptibility to various comorbidities like depression and anxiety disorders.4 The long-term HFD-induced obesity notably was known to alter emotionality and to facilitate anxiety-related behavior in rodents.33 In the present study, long-term HFD-induced obesity was demonstrated to cause oxidative stress leading to severe injury of hippocampus tissues, which increased anxiety-related behavior. These findings suggested that HFD-induced obesity could cause systemic oxidative stress and psychiatric disorders, including anxiety-related behavior.
Anxiety disorders stimulated by enhanced oxidative stress resulting from apoptosis/necrosis is the main cause of emotional and cognitive dysfunction both in humans and in animal models of obesity.34–36 Moreover, the amygdala–hippocampus subnetwork, belonging to the limbic system, influenced intrinsic brain dynamics and encodes fluctuations in mood.25 Oxidative stress is a relative overload of oxidants, including ROS and reactive nitrogen species (RNS), which causes increased free radical production that reduces cellular function and contributes to the pathophysiology of many diseases. Several reports suggested that obese patients are under oxidative stress and that various complications are related to oxidative stress.37 ROS and RNS are produced by virtually all cells and tissues. To our knowledge, excessive NO production in endothelial cells can rapidly combine with O2−˙ to form the powerful oxidant ONOO−. ONOO− is a strong 1- or 2-electron oxidant and has the capacity to nitrate tyrosine in proteins, an irreversible chemical process that consists of the addition of a nitro group to the tyrosine residues to generate 3-NT.38 In the current study, long-term HFD caused the increase of MDA and H2O2 concentrations and the decrease of SOD and catalase activities in the hippocampus, which further resulted in neurocyte death. Our data showed that increased 3-NT protein level was mainly due to the increase of ROS concentration, not the change of NO level, indicating that HFD might directly induce ROS production which further promoted the production of RNS. Besides, HFD destroyed the redox homeostasis and aggravated the degree of oxidative stress, which led to a significant increase in the number of dead hippocampal cells. Herein, we speculate that it may be the reason for HFD-induced anxiety-related behavior in mice.
Humans consume and abuse alcohol, and thus understanding alcohol's effects on the nervous system necessarily involves knowing the pharmacology of the drug. More information on the balance of effects of regular moderate alcohol consumption on anxiogenic-like symptoms in obesity disorders is, therefore, of considerable public health interest. In our previous research, we demonstrated that low-dose alcohol could improve anxiety-related behavior induced by hyperhomocysteinemia.13 According to the results of previous experiments, we found that 0.8 g kg−1 alcohol, failing to change movement time in the OFT and the number of entries into the closed arms in the EPM, had no observable hyperactivity or motor dysfunction in these mice. Excluding the acute effects of alcohol, we observed that low-dose alcohol, increasing the percentage of entries into the open arms and extending the residence time in the central area, could enhance the exploration desire and ameliorated anxiety-related behavior in HFD-fed mice. Interestingly, in East Asians, genetic variants that lead to slower metabolism of alcohol and its metabolite acetaldehyde are more common than in Europeans.39 Thus, the impact of alcohol consumption on humans requires further study.
The most novel finding of the present study is that the reduction of APN and Nrf2 expression, in the hippocampus from HFD-fed mice, was associated with abnormal oxidative stress and anxiety-related behavior, which could be partially prevented by alcohol treatment. Our results were consistent with clinical data that moderate alcohol consumption was associated with increased APN concentration, which further attenuated the level of ROS.16,40 Another study showed that hyperglycemia-induced oxidative stress by increasing lipid peroxidation inactivated Nrf2 and subsequently reduced HO-1 induction, resulting in cardiomyocyte apoptosis.21 APN supplementation activated Nrf2, which in turn facilitated myocardial HO-1 induction, reduced myocardial oxidative stress and improved cardiac function.21 Activation of Nrf2 in response to stress signals was thought to result from a disruption of this association, releasing Nrf2 for translocation into the nucleus to affect its transcriptional activity.23 The activation of the Nrf2 pathway improved neurological impairment which demonstrated that the redox homeostasis was one of the vital regulatory mechanisms.41 In this study, we found that alcohol treatment resulted in increased APN expression and Nrf2 pathway activation, consistent with findings reporting that low-dose alcohol had an antioxidative effect.42 In addition, Nrf2 activity is regulated in part by the actin-associated Kelch-like ECH-associated protein 1 (Keap1) protein, which is initially proposed to act by binding and tethering the transcription factor in the cytoplasm. Similarly, in our present study, we provided additional evidence showing that alcohol attenuated oxidative stress through producing antioxidases (SOD1, HO-1 and catalase) subsequent to reduced Keap1 activating Nrf2. Although it has been observed that low-dose alcohol enhances APN expression and activates the Nrf2 pathway in this experiment, whether inhibiting APN expression or blocking Nrf2 translocation can reverse the anxiolytic effect of low-dose alcohol in HFD-fed mice remains to be further studied.
5. Conclusions
To summarize, our findings suggested that low-dose alcohol protected HFD-induced obese mice from the augmentation of anxiety-related behavior. More importantly, these protective effects could be mediated by upregulating APN expression and increasing Nrf2 signaling expression. These results identified low-dose alcohol consumption as a promising approach to modulate metabolism-associated behavioral deficits in HFD-induced obesity-prone mice.
Author contributions
Hao Hu conceived and designed the experiments; Jie Cheng, Meng Zhang, Shaoli Cheng, Fan Li, Bingyi Zhang, Xiaoming Sun and Huijuan Hu performed the experiments; Shaoli Cheng, Lina Chen and Zhenghang Zhao analyzed the data; Hao Hu and Zhanqin Zhang contributed reagents/materials/analysis tools; Hao Hu, Zhanqin Zhang and Jie Cheng wrote the paper.
Conflicts of interest
The authors have disclosed that they do not have any conflicts of interest.
Acknowledgements
This work was funded by the Fundamental Research Funds for the Central Universities (Grants xzy012019100).
Notes and references
- E. Atlantis, R. D. Goldney and G. A. Wittert, Obesity and depression or anxiety, Br. Med. J., 2009, 339, b3868 CrossRef.
- M. Guo, C. Li, Y. Lei, S. Xu, D. Zhao and X. Y. Lu, Role of the adipose PPARgamma-adiponectin axis in susceptibility to stress and depression/anxiety-related behaviors, Mol. Psychiatry, 2017, 22, 1056–1068 CrossRef CAS.
- K. G. Kahl, U. Schweiger, C. Correll, C. Muller, M. L. Busch, M. Bauer and P. Schwarz, Depression, anxiety disorders, and metabolic syndrome in a population at risk for type 2 diabetes mellitus, Brain Behav., 2015, 5, e00306 Search PubMed.
- Y. Alonso-Caraballo, K. J. Hodgson, S. A. Morgan, C. R. Ferrario and P. J. Vollbrecht, Enhanced anxiety-like behavior emerges with weight gain in male and female obesity-susceptible rats, Behav. Brain Res., 2019, 360, 81–93 CrossRef CAS.
- S. Dutheil, K. T. Ota, E. S. Wohleb, K. Rasmussen and R. S. Duman, High-Fat Diet Induced Anxiety and Anhedonia: Impact on Brain Homeostasis and Inflammation, Neuropsychopharmacology, 2016, 41, 1874–1887 CrossRef CAS.
- R. J. Anderson, K. E. Freedland, R. E. Clouse and P. J. Lustman, The prevalence of comorbid depression in adults with diabetes: a meta-analysis, Diabetes Care, 2001, 24, 1069–1078 CrossRef CAS.
- F. N. Jacka, N. Cherbuin, K. J. Anstey, P. Sachdev and P. Butterworth, Western diet is associated with a smaller hippocampus: a longitudinal investigation, BMC Med., 2015, 13, 215 CrossRef.
- C. S. Ho, Y. Lu, N. Ndukwe, M. W. Chew, A. Shabbir, J. B. So and R. C. Ho, Symptoms of Anxiety and Depression in Obese Singaporeans: a Preliminary Study, East Asian Arch. Psychiatry, 2018, 28, 3–8 Search PubMed.
- Y. Li, J. Schoufour, D. D. Wang, K. Dhana, A. Pan, X. Liu, M. Song, G. Liu, H. J. Shin, Q. Sun, L. Al-Shaar, M. Wang, E. B. Rimm, E. Hertzmark, M. J. Stampfer, W. C. Willett, O. H. Franco and F. B. Hu, Healthy lifestyle and life expectancy free of cancer, cardiovascular disease, and type 2 diabetes: prospective cohort study, Br. Med. J., 2020, 368, l6669 CrossRef.
- R. A. Bell, E. J. Mayer-Davis, M. A. Martin, R. B. D'Agostino Jr. and S. M. Haffner, Associations between alcohol consumption and insulin sensitivity and cardiovascular disease risk factors: the Insulin Resistance and Atherosclerosis Study, Diabetes Care, 2000, 23, 1630–1636 CrossRef CAS.
- P. E. Ronksley, S. E. Brien, B. J. Turner, K. J. Mukamal and W. A. Ghali, Association of alcohol consumption with selected cardiovascular disease outcomes: a systematic review and meta-analysis, Br. Med. J., 2011, 342, d671 CrossRef.
- R. G. Scott, C. H. Wiener and D. Paulson, The Benefit of Moderate Alcohol Use on Mood and Functional Ability in Later Life: Due to Beers or Frequent Cheers?, Gerontologist, 2020, 60, 80–88 Search PubMed.
- J. Cheng, F. Li, X. Sun, S. Liu, L. Chen, F. Tian, Z. Zhao, H. Hu and X. Li, Low-dose alcohol ameliorated homocysteine-induced anxiety-related behavior via attenuating oxidative stress in mice, Neurosci. Lett., 2019, 714, 134568 CrossRef.
- A. Sierksma, H. Patel, N. Ouchi, S. Kihara, T. Funahashi, R. J. Heine, D. E. Grobbee, C. Kluft and H. F. Hendriks, Effect of moderate alcohol consumption on adiponectin, tumor necrosis factor-alpha, and insulin sensitivity, Diabetes Care, 2004, 27, 184–189 CrossRef CAS.
- C. Thamer, M. Haap, A. Fritsche, H. Haering and M. Stumvoll, Relationship between moderate alcohol consumption and adiponectin and insulin sensitivity in a large heterogeneous population, Diabetes Care, 2004, 27, 1240 CrossRef.
- M. M. Joosten, R. F. Witkamp and H. F. Hendriks, Alterations in total and high-molecular-weight adiponectin after 3 weeks of moderate alcohol consumption in premenopausal women, Metab., Clin. Exp., 2011, 60, 1058–1063 CrossRef CAS.
- K. Maeda, K. Okubo, I. Shimomura, T. Funahashi, Y. Matsuzawa and K. Matsubara, cDNA cloning and expression of a novel adipose specific collagen-like factor, apM1 (AdiPose Most abundant Gene transcript 1), Biochem. Biophys. Res. Commun., 1996, 221, 286–289 CrossRef CAS.
- A. Ahmad, T. Ali, M. W. Kim, A. Khan, M. H. Jo, S. U. Rehman, M. S. Khan, N. B. Abid, M. Khan, R. Ullah, M. G. Jo and M. O. Kim, Adiponectin homolog novel osmotin protects obesity/diabetes-induced NAFLD by upregulating AdipoRs/PPARalpha signaling in ob/ob and db/db transgenic mouse models, Metab., Clin. Exp., 2019, 90, 31–43 CrossRef CAS.
- R. C. Ng and K. H. Chan, Potential Neuroprotective Effects of Adiponectin in Alzheimer’s Disease, Int. J. Mol. Sci., 2017, 18, 592 CrossRef.
- K. H. Chan, K. S. Lam, O. Y. Cheng, J. S. Kwan, P. W. Ho, K. K. Cheng, S. K. Chung, J. W. Ho, V. Y. Guo and A. Xu, Adiponectin is protective against oxidative stress induced cytotoxicity in amyloid-beta neurotoxicity, PLoS One, 2012, 7, e52354 CrossRef CAS.
- H. Li, W. Yao, M. G. Irwin, T. Wang, S. Wang, L. Zhang and Z. Xia, Adiponectin ameliorates hyperglycemia-induced cardiac hypertrophy and dysfunction by concomitantly activating Nrf2 and Brg1, Free Radical Biol. Med., 2015, 84, 311–321 CrossRef CAS.
- N. T. Pun and P. H. Park, Role of p62 in the suppression of inflammatory cytokine production by adiponectin in macrophages: Involvement of autophagy and p21/Nrf2 axis, Sci. Rep., 2017, 7, 393 CrossRef.
- T. Nguyen, P. Nioi and C. B. Pickett, The Nrf2-antioxidant response element signaling pathway and its activation by oxidative stress, J. Biol. Chem., 2009, 284, 13291–13295 CrossRef CAS.
- G. Xu, C. Li, A. L. Parsiola, J. Li, K. D. McCarter, R. Shi, W. G. Mayhan and H. Sun, Dose-Dependent Influences of Ethanol on Ischemic Stroke: Role of Inflammation, Front. Cell. Neurosci., 2019, 13, 6 CrossRef CAS.
- L. A. Kirkby, F. J. Luongo, M. B. Lee, M. Nahum, T. M. Van Vleet, V. R. Rao, H. E. Dawes, E. F. Chang and V. S. Sohal, An Amygdala-Hippocampus Subnetwork that Encodes Variation in Human Mood, Cell, 2018, 175, 1688–1700 CrossRef CAS.
- F. McMurray, D. A. Patten and M. E. Harper, Reactive Oxygen Species and Oxidative Stress in Obesity-Recent Findings and Empirical Approaches, Obesity, 2016, 24, 2301–2310 CrossRef CAS.
- J. W. Beulens, L. J. van Loon, F. J. Kok, M. Pelsers, T. Bobbert, J. Spranger, A. Helander and H. F. Hendriks, The effect of moderate alcohol consumption on adiponectin oligomers and muscle oxidative capacity: a human intervention study, Diabetologia, 2007, 50, 1388–1392 CrossRef CAS.
- K. N. Lewis, E. Wason, Y. H. Edrey, D. M. Kristan, E. Nevo and R. Buffenstein, Regulation of Nrf2 signaling and longevity in naturally long-lived rodents, Proc. Natl. Acad. Sci. U. S. A., 2015, 112, 3722–3727 CrossRef CAS.
- Y. Tomiga, S. Yoshimura, S. G. Ra, Y. Takahashi, R. Goto, I. Kugimoto, Y. Uehara, K. Kawanaka and Y. Higaki, Anxiety-like behaviors and hippocampal nNOS in response to diet-induced obesity combined with exercise, J. Physiol. Sci., 2019, 69, 711–722 CrossRef CAS.
- Y. Milaneschi, W. K. Simmons, E. F. C. van Rossum and B. W. Penninx, Depression and obesity: evidence of shared biological mechanisms, Mol. Psychiatry, 2018, 24, 18–33 CrossRef.
- C. Andre, A. L. Dinel, G. Ferreira, S. Laye and N. Castanon, Diet-induced obesity progressively alters cognition, anxiety-like behavior and lipopolysaccharide-induced depressive-like behavior: focus on brain indoleamine 2,3-dioxygenase activation, Brain, Behav., Immun., 2014, 41, 10–21 CrossRef CAS.
- L. van Dammen, V. Wekker, S. R. de Rooij, H. Groen, A. Hoek and T. J. Roseboom, A systematic review and meta-analysis of lifestyle interventions in women of reproductive age with overweight or obesity: the effects on symptoms of depression and anxiety, Obes. Rev., 2018, 19, 1679–1687 CrossRef CAS.
- H. M. Jang, S. K. Han, J. K. Kim, S. J. Oh, H. B. Jang and D. H. Kim, Lactobacillus sakei Alleviates High-Fat-Diet-Induced Obesity and Anxiety in Mice by Inducing AMPK Activation and SIRT1 Expression and Inhibiting Gut Microbiota-Mediated NF-kappaB Activation, Mol. Nutr. Food Res., 2019, 63, e1800978 CrossRef.
- B. Gargouri, H. S. Bhatia, M. Bouchard, B. L. Fiebich and H. Fetoui, Inflammatory and oxidative mechanisms potentiate bifenthrin-induced neurological alterations and anxiety-like behavior in adult rats, Toxicol. Lett., 2018, 294, 73–86 CrossRef CAS.
- S. J. Gainey, K. A. Kwakwa, J. K. Bray, M. M. Pillote, V. L. Tir, A. E. Towers and G. G. Freund, Short-Term High-Fat Diet (HFD) Induced Anxiety-Like Behaviors and Cognitive Impairment Are Improved with Treatment by Glyburide, Front. Behav. Neurosci., 2016, 10, 156 Search PubMed.
- F. Ng, M. Berk, O. Dean and A. I. Bush, Oxidative stress in psychiatric disorders: evidence base and therapeutic implications, Int. J. Neuropsychopharmacol., 2008, 11, 851–876 CAS.
- H. T. Roh, S. Y. Cho and W. Y. So, Obesity promotes oxidative stress and exacerbates blood-brain barrier disruption after high-intensity exercise, JSHS, 2017, 6, 225–230 Search PubMed.
- R. Radi, Nitric oxide, oxidants, and protein tyrosine nitration, Proc. Natl. Acad. Sci. U. S. A., 2004, 101, 4003–4008 CrossRef CAS.
- M. J. Shin, Y. Cho and D. G. Smith, Alcohol Consumption, Aldehyde Dehydrogenase 2 Gene Polymorphisms, and Cardiovascular Health in Korea, Yonsei Med. J., 2017, 58, 689–696 CrossRef CAS.
- M. Matsuda and I. Shimomura, Roles of adiponectin and oxidative stress in obesity-associated metabolic and cardiovascular diseases, Rev. Endocr. Metab. Disord., 2014, 15, 1–10 CrossRef CAS.
- H. Ding, X. Wang, H. Wang, L. Zhu, Q. Wang, Y. Jia, W. Wei, C. Zhou, H. Wu and K. Ding, Nrf2-ARE signaling provides neuroprotection in traumatic brain injury via modulation of the ubiquitin proteasome system, Neurochem. Int., 2017, 111, 32–44 CrossRef CAS.
- W. J. Bae, Y. S. Choi, S. J. Kim, H. J. Cho, S. H. Hong, S. W. Kim, T. K. Hwang, D. J. Kim and J. Y. Lee, Effects of Moderate Alcohol Intake in the Bladder of the Otsuka Long Evans Tokushima Fatty Diabetic Rats, J. Korean Med. Sci., 2015, 30, 1313–1320 CrossRef CAS.
|
This journal is © The Royal Society of Chemistry 2021 |