DOI:
10.1039/C9FO02262G
(Paper)
Food Funct., 2020,
11, 799-812
The preventive effect of phenolic-rich extracts from Chinese sumac fruits against nonalcoholic fatty liver disease in rats induced by a high-fat diet
Received
27th September 2019
, Accepted 29th December 2019
First published on 30th December 2019
Abstract
The objective of this study is to investigate the preventive effect of phenolic-rich extracts from Chinese sumac (Rhus chinensis Mill.) fruits against nonalcoholic fatty liver disease (NAFLD) in rats induced by a high-fat diet and to clarify the underlying mechanisms. The results showed that the phenolic-rich extract remarkably improved some critical biochemical indexes, including TG, TC, MDA, ALT, AST, and endogenous antioxidant enzymes. The results of immunofluorescence and TUNEL assay showed that the extract obviously reduced the level of NF-κB in cell nuclei and suppressed hepatocyte apoptosis. Moreover, immunohistochemistry and western blot analyses further revealed that the phenolic-rich extract can improve NAFLD in high-fat diet induced rats by regulating several key proteins related to lipid metabolism, inflammation and apoptosis of hepatocytes, namely upregulating the expression levels of p-AMPK, PPAR-α, CPT1 and Bcl-2, and downregulating the levels of PPAR-γ, CYP2E1, p-P38, p-NF-κB, iNOS, COX-2, caspase-3D and Bax. These results indicate that the phenolic-rich extract from Chinese sumac fruits could prevent NAFLD in rats by regulating some critical proteins in several signalling pathways and may be provided as a new natural ingredient for developing functional foods and/or nutraceuticals to prevent NAFLD.
1. Introduction
Nonalcoholic fatty liver disease (NAFLD) is one of the most common chronic liver diseases in the world.1 The main feature of NAFLD is excessive accumulation of fat (triglyceride, TG) in liver cells without significant alcohol consumption and with no other clear causes of liver damage.2 NAFLD may develop from simple hepatic steatosis in early stages into more serious liver diseases, including nonalcoholic steatohepatitis (NASH), hepatic fibrosis, hepatic cirrhosis, and hepatic cellular carcinoma.3 An epidemiological study showed that the global prevalence of NAFLD is about 25%.2 Among NAFLD sufferers, 59% have NASH.2 Moreover, many previous studies have proved that NAFLD is closely related to type 2 diabetes, obesity, and dyslipidemia.2,3
The pathogenesis of NAFLD is complex, and NAFLD may be caused by a variety of regulatory disorders, including hepatocyte oxidative stress, lipid accumulation, inflammation, apoptosis, endoplasmic reticulum stress, and mitochondrial dysfunction.2 The liver synthesizes a large amount of TGs to maintain the balance of energy metabolism when a high-fat diet is taken in for a long time. However, the TGs accumulate in the liver when the content of synthetic TGs exceeds the liver transport rate.2 These accumulated TGs are excessively oxidized in the liver, which results in the production of reactive oxygen species (ROS) and malondialdehyde (MDA), thereby causing damage to organelles, such as the mitochondria and the cell membrane, and leading to cell inflammation and apoptosis. The condition progresses to NASH and other severe liver diseases.1,4 Therefore, the regulatory factors related to lipid metabolism, inflammation, and apoptosis signalling pathways play important roles in NAFLD. Peroxidase proliferative receptor-α (PPAR-α), carnitine palmitoyl transferase-1 (CPT1), and PPAR-γ are vital in β-oxidation and fatty acid synthesis.2 P38 mitogen-activated protein kinase (P38) and nuclear factor κB (NF-κB) play critical roles in inflammation regulation.5 The cleavage of the protein cysteinyl aspartate specific proteinase-3 (caspase-3) induces cell apoptosis.6 The improvement of these protein regulatory factors can improve NAFLD and is the key to NAFLD treatment and prevention.2,7
NAFLD has a very high incidence, but there is no specific clinical drug used for its treatment. Therefore, preventing NAFLD through the intake of natural active ingredients from food materials may be particularly important. Many studies have found that various vegetables and fruits are rich in phenolic substances, which are considered their main active ingredients. These substances can effectively prevent or alleviate NAFLD in vivo and in vitro.4,7,8 Chinese sumac (Rhus chinensis Mill.) is widely distributed in Asia and belongs to the family Anacardiaceae.9 According to folk medicine records, the fruits of this plant have been used to treat or prevent several liver-related diseases, such as hepatitis, phlegm, and jaundice.9–12 Moreover, we previously reported that the fruits are enriched in phenolic substances and contain about 10 kinds of phenolic substances, including gallic acid, quercetin-3-O-arabinoside, quercetin-3-O-rhamnoside, myricetin-3-O-galactoside, and myricetin-3-O-rhamnoside.9,11,12 We also found that different extracts from this fruit, especially an 80% ethanol extract (rich in phenolic compounds), prevented alcoholic fatty liver in rats.9 Based on previous results and traditional records, it can be hypothesized that the phenolic-rich extract from these fruits may prevent NAFLD. However, to our knowledge, a scientific study on the NAFLD prevention of Chinese sumac fruits has not been conducted. Thus, the present work investigated whether the phenolic-rich extract of Chinese sumac fruits can prevent NAFLD effectively in rats fed with a high-fat diet and aimed to clarify the potential underlying mechanisms via the determination of several key protein expression levels of multiple signalling pathways.
2. Materials and methods
2.1 Reagents
All biochemical detection kits used were purchased from the Nanjing Jiancheng Bioengineering Institute (Nanjing, China). All antibodies used in the western blot test in this study were provided by Abcam (Cambridge, UK). Other reagents used were of analytical grade.
2.2 Preparation and analysis of the sample
Chinese sumac (R. chinensis Mill.) fruits were supplied by Kunming Plant Classification Biotechnology Co., Ltd (October 2017) and stored at −20 °C. The phenolic-rich extract of Chinese sumac fruits was prepared with 80% ethanol according to the method reported in our previous paper.9,12 The total phenolic content (TPC) of the phenolic-rich extract was 418.72 ± 29.58 mg of gallic acid equivalent per g of dried extract weight. The phenolic composition of this extract was identified and quantified by using UHPLC-ESI-HRMS/MS. As we reported previously, the extract contained gallic acid, quercetin-3-O-rhamnoside, myricetin-3-O-rhamnoside, kaempferol-3-O-hexoside, and myricetin-3-O-galactoside.9 The extract was stored at −20 °C for further use.
2.3 Animal treatment
24 Sprague-Dawley male rats weighing 200 g ± 5% (no specific pathogen, 6–8 weeks old) used in this experiment were supplied by Liaoning Changsheng Biotechnology Co., Ltd (Certificate no.: SCKK (Liao) 2015-0002). All rats were adapted to the experimental environment for one week before the formal experiment and were randomly divided into four groups: group C (control group), group M (model group), group EL (low dose group, 200 mg of extract per kg of body weight, about 83.74 mg of TPC per kg of body weight), and group EH (high dose group, 600 mg of extract per kg of body weight, about 251.23 mg of TPC per kg of body weight). The rats in group C were given basic feed, whereas the rats in the other groups were supplied with high-fat feed (60% basic feed, 15% lard oil, 10% yolk powder, 12% corn oil, 2.5% cholesterol, and 0.5% bile salts), which was provided by Kunming Zanna Biotechnology Co., Ltd (Kunming, Yunnan, China). The other conditions of the raising environment (temperature, humidity, light time, and other feeding conditions) are mentioned in our previous study.9,13 The experiment was performed for 12 weeks, and the gavage volume was 5.0 mL kg−1. Groups C and M were given the corresponding volume of distilled water, whereas groups EL and EH were given the corresponding volume of the sample. The criteria for selecting the doses of the extract were based on our previous study and preliminary experiment.9 All animal procedures performed in this work were in strict accordance with the National Institutes of Health Guide for the Care and Use of Laboratory Animals and were confirmed by the Ethical Committee for Animal Experimentation of Kunming University of Science and Technology.
2.4 Biochemical indicators of plasma and the liver
After 12 weeks of feeding, all rats were anesthetized. Blood was collected through the celiac artery by using a tube that contained lithium heparin and was centrifuged at 2200g and 4 °C for 5 min to collect the plasma. The plasma biochemical parameters, including total cholesterol (TC), TG, alanine aminotransferase (ALT), and aspartate transaminase (AST), were measured using the corresponding kit based on the manufacturer's instructions. The liver tissue of each rat was weighed to calculate the liver coefficient. The homogenate of each liver tissue was prepared to analyze TG, TC, catalase (CAT), superoxide dismutase (SOD), reduced glutathione (GSH), and MDA. Liver tissue (0.1 g) was mixed with 0.9 mL NaCl solution (0.9%), and the mixture was homogenized using a Scientz-II D ultrasonic cell crusher (Ningbo Scientz, Ningbo, China). The tissue homogenate was centrifuged at 11
000g and 4 °C for 5 min. Finally, the supernatant was collected and stored at −80 °C for further use.
2.5 Histopathological evaluation
Histopathological evaluation of the hepatic lobule was performed through hematoxylin–eosin (H&E) staining. The detailed steps are as follows. The hepatic lobule was separated and fixed with 4% paraformaldehyde. Then, the tissue was embedded in paraffin (wax block). The stained section of the liver was observed using an Olympus IX83 microscope (Tokyo, Japan) at 200× and 400× magnifications.
2.6 Immunohistochemical analysis
Immunohistochemistry was used to assess the expression levels of phosphorylated AMP-activated protein kinase (p-AMPK), PPAR-α, and fatty acid synthase (FAS) proteins in the left lobe of the liver. The liver was separated and fixed with 4% paraformaldehyde for 24 h. After fixing, the tissue was embedded in paraffin (wax block). The slice of the wax block was mixed with dimethyl benzene to deparaffinize, rehydrated with graded alcohol, and blocked with 5% bovine serum albumin for 10–30 min. Then, the tissue section was incubated overnight with the corresponding primary antibody and washed using phosphate buffer saline (PBS) before incubation with a secondary antibody. Thereafter, all sections were observed under the microscope.14
2.7 Immunofluorescence microscopy
Immunofluorescence microscopy was used to assess the expression of p-NF-κB protein in the liver's left lobe. The wax block of the liver was dewaxed and rehydrated with graded alcohol. Thereafter, the liver slice was incubated overnight at 4 °C with primary antibodies. Subsequently, the liver section was washed thrice using PBS and incubated with a CY3-conjugated anti-rabbit IgG secondary antibody. The nuclei were stained with 6-diamidino-2-phenylindole (DAPI). Finally, all sections were observed under the microscope.15
2.8 TUNEL assay
Hepatocyte apoptosis was detected by TUNEL staining. The TUNEL assay was performed as follows. The wax block was dewaxed and rehydrated as mentioned in the “2.6 Immunohistochemical analysis”. Then, the liver slice was incubated with TUNEL reagents at 37 °C for approximately 2 h in the dark. The nuclei of hepatocytes were loaded with DAPI. Finally, the TUNEL-positive signals were observed using the microscope.16
2.9 Western blot analysis
The liver lobule was placed in lysis buffer containing inhibitors of protease and phosphatase, homogenized with the Scientz-II D ultrasonic cell crusher (Ningbo Scientz, Ningbo, China), and lysed in an ice bath for 30 min. The tissue homogenate was centrifuged at 10
000g and 4 °C for 5 min. The protein content in the supernatant was detected using a BCA kit. Each sample (30 μg) was loaded on 10% or 12.5% SDS–PAGE gel. The targeted protein was transferred onto a nitrocellulose (NC) membrane (Gelman Laboratory, Ann Arbor, USA), which was incubated overnight with the corresponding primary antibody at 4 °C. Afterward, the NC membrane was incubated with a secondary antibody. After incubation with the antibodies, the protein was measured with an enhanced chemiluminescent detection reagent (Millipore, USA) by using a VILBER Fusion FX7 imaging system (Marne-la-Vallée, France).9
2.10 Statistical analysis
Study results were expressed as mean ± standard error (SE) and analyzed using the OriginLab software (OriginLab, Northampton, MA, USA) by one-way analysis of variance. The Tukey test was used to examine significant differences (p < 0.05). The Image-Pro plus 6.0 software (Media Cybernetics Inc.; Rockville, MD, USA) was used to evaluate the results of immunohistochemistry.9
3. Results
3.1 Biochemical indicators of plasma
The results of biochemical indicators in plasma (TG, TC, AST, and ALT) are summarized in Table 1. At the end of the experiment, the contents of TG, TC, AST, and ALT in group M increased significantly compared with those in group K (p < 0.05). Administration of the sample evidently improved these biochemical parameters in plasma. The contents of TG, AST, and ALT in group EL significantly decreased compared with those in group M (p < 0.05), whereas the TC content in group EL exhibited no significant difference from that in group M (p > 0.05). The levels of TG and TC in group EH were significantly lower than those in group M (p < 0.05) and were similar to those in group C (p > 0.05). AST and ALT contents in group EH remarkably decreased compared with those in group M (p < 0.05). However, all the biochemical parameters of plasma in groups EH and EL were not significantly different (p > 0.05).
Table 1 Biochemical indicators in plasma and liver of NAFLD rats fed with high-fat diet
Parameters |
C |
M |
EL |
EH |
Values were expressed as the mean ± SE (n = 6); different letters in the same row indicate significant differences (p < 0.05). C: Group C (control), M: group M (model), EL: group EL (200 mg kg−1, ethanol extract), EH: group EH (600 mg kg−1, ethanol extract). |
Plasma |
TG (mg dL−1) |
99.59 ± 4.72a |
176.38 ± 5.00b |
118.56 ± 4.08c |
106.29 ± 3.96ac |
TC (mg dL−1) |
145.60 ± 10.72a |
211.22 ± 19.11b |
169.12 ± 9.09ab |
150.56 ± 4.50a |
AST (U L−1) |
89.67 ± 4.04a |
166.72 ± 9.25b |
114.37 ± 4.25c |
106.00 ± 3.10c |
ALT (U L−1) |
34.32 ± 2.94a |
104.41 ± 4.79b |
68.19 ± 5.85c |
51.15 ± 1.86c |
|
Liver tissue |
Liver coefficient (g per 100 g) |
2.63 ± 0.08a |
3.51 ± 0.11b |
3.03 ± 0.23bc |
2.86 ± 0.14ac |
TG (mmol g−1 prot.) |
8.16 ± 1.48a |
19.42 ± 1.55b |
13.24 ± 0.99a |
11.66 ± 1.25a |
TC (mmol g−1 prot.) |
3.49 ± 0.17a |
5.73 ± 0.28b |
4.27 ± 0.37a |
3.94 ± 0.21a |
CAT (U mg−1 prot.) |
85.67 ± 2.55a |
56.65 ± 3.47b |
64.49 ± 2.05bc |
73.84 ± 4.14ac |
SOD (U mg−1 prot.) |
277.34 ± 6.65a |
154.62 ± 5.74b |
178.35 ± 7.81bc |
235.21 ± 6.61ac |
GSH (mU mg−1 prot.) |
269.91 ± 11.32a |
178.11 ± 6.60b |
210.85 ± 6.10c |
225.41 ± 5.77c |
MDA (nmol mg−1 prot.) |
11.46 ± 1.48a |
28.63 ± 0.68b |
22.60 ± 1.41c |
20.05 ± 1.22c |
3.2 Biochemical indicators of the liver
The results of biochemical parameters of the liver tissue (liver coefficient, TG, TC, CAT, SOD, GSH, and MDA) are listed in Table 1. These biochemical parameters in group M exhibited significant differences compared with those in group C (p < 0.05). Administration of the sample, especially at a high dose, significantly improved most of these parameters compared with group M (p < 0.05). The levels of TG, TC, GSH, and MDA in group EL significantly decreased compared with those in group M (p < 0.05). The contents of TG and TC in group EL were close to those in group C (p > 0.05). Meanwhile, the results of the liver coefficient, CAT, and SOD in group EL showed no significant difference from those in group M (p > 0.05). These biochemical parameters were all significantly improved in group EH compared with those in group M (p < 0.05). Moreover, these parameters except GSH and MDA in group EH were similar to those in group C (p > 0.05). Although the values of these parameters in group EH were better than those in group EL, a statistically significant difference in the parameters was not observed between the two groups (p > 0.05).
3.3 Histopathological analysis
Histopathological analysis of the liver was achieved by observing the appearance of the liver and H&E staining. The results of histopathological examination are shown in Fig. 1. The livers in group C were bright red and smooth (Fig. 1A). Meanwhile, in group M, the whole liver appeared creamy white with visible white particles on the surface. The appearance of the liver in groups EL and EH evidently improved, and the color of the liver was reddish and similar in appearance to the liver in group C. The H&E staining results of the liver observed at 200× and 400× magnifications are presented in Fig. 1B and C, respectively. As shown in Fig. 1B, the Diss cavity of the liver in group C was normal with no obvious abnormality. However, many lipid droplets and cytoplasmic vacuoles were observed in the liver of group M. Lipid droplets and cytoplasmic vacuoles were significantly improved in the sample-treated groups, especially in group EH. Besides lipid droplets and cytoplasmic vacuoles, other histopathological details of the liver were observed under high magnification (400×) (Fig. 1C). In group C, the hepatocytes were intact with a clear outline, and the Diss cavity space was normal. In contrast, group M showed the crowding of hepatocyte nuclei to the edge of the cell due to accumulation of lipid droplets. Group M also had unclear hepatocyte outlines, and the Diss cavity of liver tissues disappeared. After being treated with the sample, group M's abnormalities were all alleviated, especially in group EH, which was treated with the sample at a high dose.
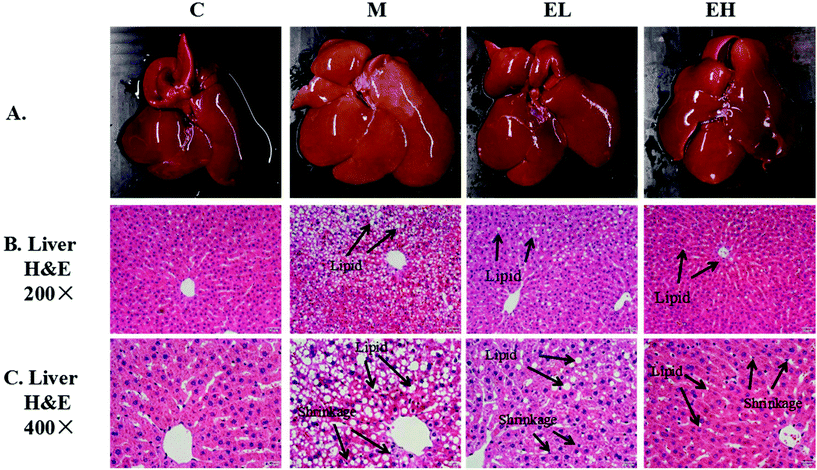 |
| Fig. 1 Results of liver appearance and H&E staining; A: picture of rat liver; B and C: results of liver H&E staining section ×200 and ×400, respectively. C (top): group C (control), M: group M (model), EL: group EL (200 mg kg−1, ethanol extract), and EH: group EH (600 mg kg−1, ethanol extract). | |
3.4 Immunohistochemical analysis
Immunohistochemistry was used to detect p-AMPK (Fig. 2A), PPAR-α (Fig. 2B), and FAS (Fig. 2C) proteins in the liver. The results of the relative expression levels of different proteins by immunohistochemistry are presented in Fig. 2D. As seen in Fig. 2A and D, the p-AMPK expression in the liver of group M decreased remarkably compared with that in group C (p < 0.05). In contrast, the p-AMPK expression in extract-treated groups (groups EL and EH) improved significantly (p < 0.05), especially in group EH, the results of which were close to those in group C (p > 0.05). PPAR-α expression results in Fig. 2B and D illustrate a similar phenomenon to p-AMPK expression. PPAR-α protein expression in group M significantly decreased compared with that in group C (p < 0.05). However, its expression was significantly increased (p < 0.05) after treatment with the extract, especially in group EH, which was similar to that in group C (p > 0.05). The results for FAS protein are shown in Fig. 2C and D. The FAS protein expression in group M was higher than that in group C (p < 0.05). However, extract treatment significantly inhibited the level of FAS protein. The FAS expression levels in groups EL and EH were significantly lower than that in group M (p < 0.05).
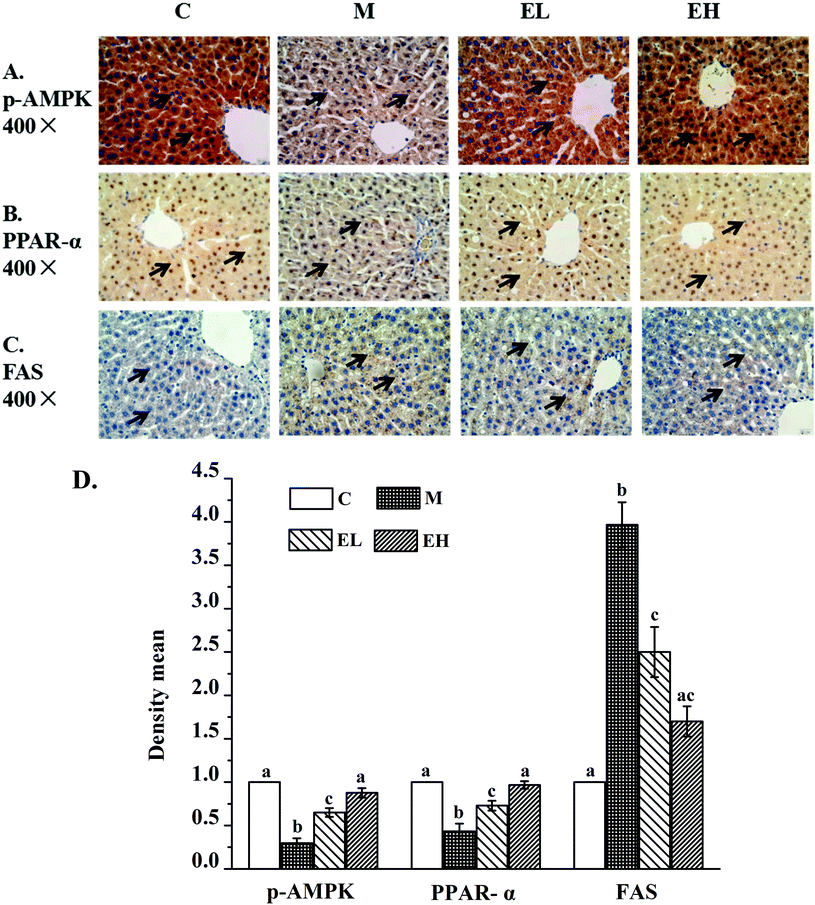 |
| Fig. 2 Immunohistochemical results of p-AMPK (A), PPAR-α (B) and FAS (C) in rat livers. A: Results of p-AMPK protein; the claybank part represents p-AMPK protein; B: results of PPAR-α protein; the yellow part represents PPAR-α protein; C: results of FAS, the yellow area indicates FAS protein; and D: the relative protein expression of p-AMPK, PPAR-α and FAS. The relative protein expression in immunohistochemistry was quantified by using Image-Pro Plus software. The quantitative result is expressed in terms of density mean (density mean = density sum/area sum) and the above proteins were quantified by normalizing with group C. C (top): group C (control), M: group M (model), EL: group EL (200 mg kg−1, ethanol extract), and EH: group EH (600 mg kg−1, ethanol extract). All the values are expressed as mean ± SE (n = 4). Different letters of the same protein indicate significant differences (p < 0.05). | |
3.5 Analysis of protein expression
Western blot analysis was used to detect the expression levels of proteins involved in lipid metabolism, inflammation, and cell apoptosis, and the results are shown in Fig. 3, 4, and 5, respectively. The expression levels of AMPK, p-AMPK, PPAR-α, CPT1, and PPAR-γ, which were involved in lipid metabolism, were determined in this work. Fig. 3 shows that the expression levels of these lipid metabolism regulatory proteins significantly changed in group M compared with those in group C (p < 0.05). Treatment with the extract at a low dose did not significantly affect the expression levels of these proteins compared with those in group M (p > 0.05) except PPAR-γ, whose expression remarkably decreased (p < 0.05). Compared with group M, the administration of the high-dose extract significantly improved the expression levels of all proteins (p < 0.05). The expression levels of all proteins were similar to those in group C (p > 0.05).
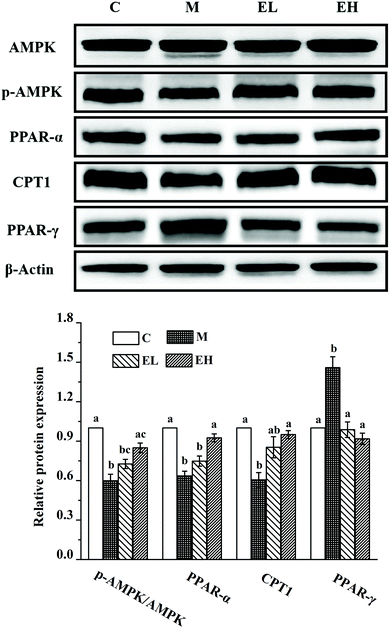 |
| Fig. 3 Results of the phenolic-rich extract from Chinese sumac fruits on the expression of several key proteins related to lipid metabolism in the liver, including amp-activated protein kinase (AMPK), phosphorylated amp-activated protein kinase (p-AMPK), peroxidase proliferative receptor-α (PPAR-α), carnitine palmitoyl transferase-1 (CPT1) and peroxidase proliferative receptor-γ (PPAR-γ). The relative expression levels of the above proteins were quantified by normalizing with group C and compared with β-actin. C: Group C (control), M: group M (model), EL: group EL (200 mg kg−1, ethanol extract), and EH: group EH (600 mg kg−1, ethanol extract). All the values are expressed as mean ± SE (n = 4). Different letters of the same protein indicate significant differences (p < 0.05). | |
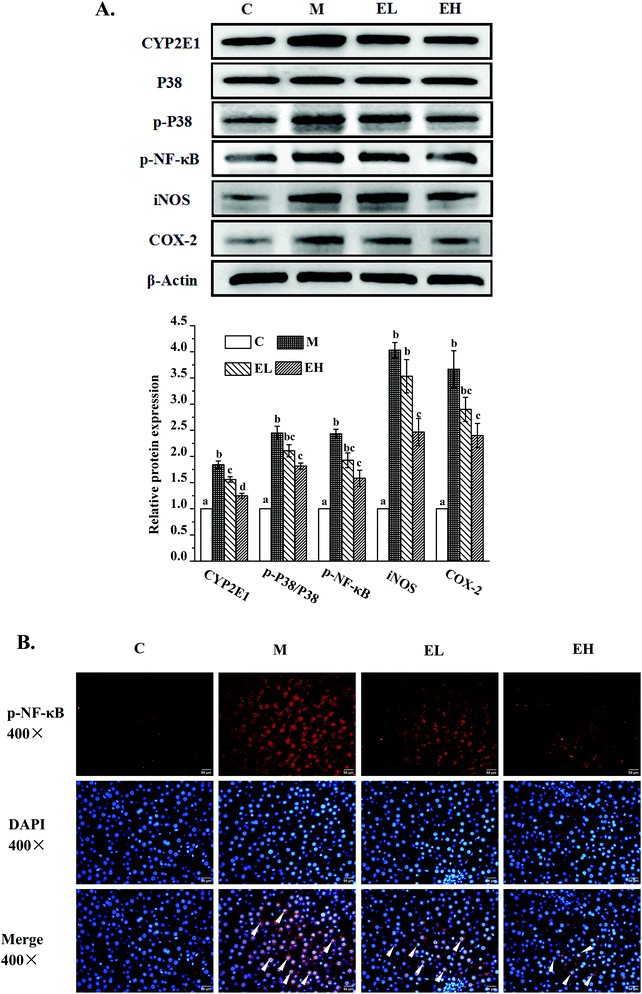 |
| Fig. 4 Results of the phenolic-rich extract from Chinese sumac fruits on the expression of several key proteins (A) related to inflammation in the liver, including cytochrome p450-2e1 (CYP2E1), P38 mitogen-activated protein kinase (P38), phosphorylated P38 mitogen-activated protein kinase (p-P38), phosphorylated nuclear factor κB (p-NF-κB), inducible nitric oxide synthase (iNOS) and cyclooxygenase-2 (COX-2), and p-NF-κB immunofluorescence (B). A: The relative expression levels of the above proteins were quantified by normalizing with group C and compared with β-actin. B: The immunofluorescence results of NF-κB: the red fluorescence part is the protein of p-NF-κB; the blue fluorescence is the nucleus; and the overlap of red and blue fluorescence indicates that p-NF-κB protein enters the nucleus to exert the effect of inducing inflammation. C: Group C (control), M: group M (model), EL: group EL (200 mg kg−1, ethanol extract), and EH: group EH (600 mg kg−1, ethanol extract). All the values are expressed as mean ± SE (n = 4). Different letters of the same protein indicate significant differences (p < 0.05). | |
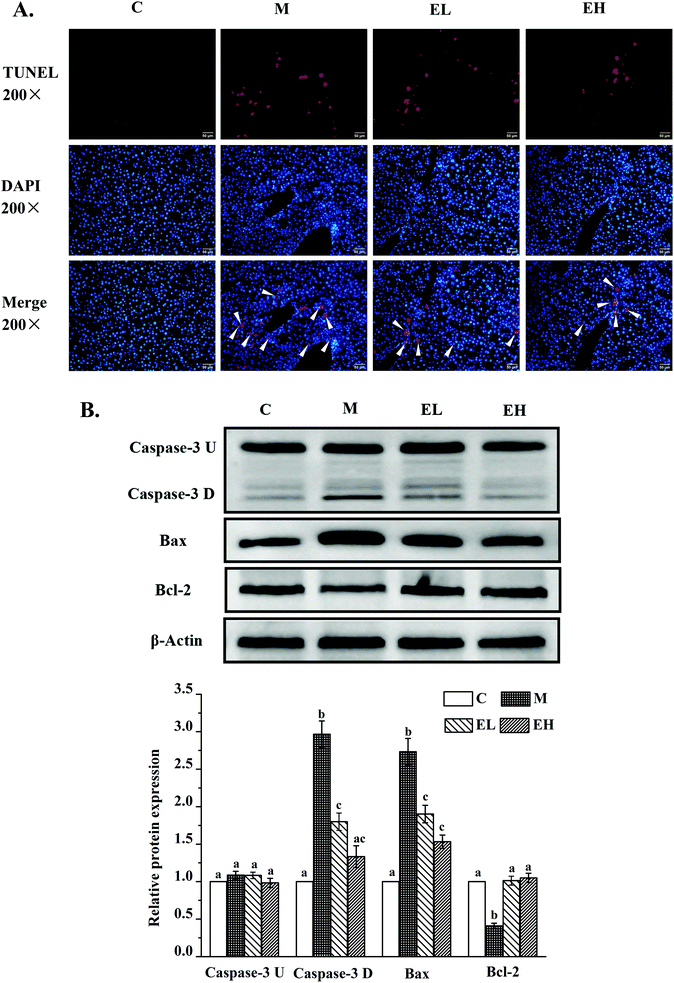 |
| Fig. 5 Results of TUNEL assay (A) and the phenolic-rich extract from Chinese sumac fruits on the expression of several key proteins related to apoptosis in the liver (B), including cysteinyl aspartate specific proteinase-3 (caspase-3 U), cleaved cysteinyl aspartate specific proteinase-3 (caspase-3 D), BCL2-associated X (Bax) and B-cell lymphoma-2 (Bcl-2). A: the results of TUNEL assay; the red fluorescence part is the broken DNA; the blue fluorescence is the nucleus; and the overlap of red and blue fluorescence indicates the cell apoptosis. B: The relative expression levels of the above proteins were quantified by normalizing with group C and compared with β-actin. C: Group C (control), M: group M (model), EL: group EL (200 mg kg−1, ethanol extract), and EH: group EH (600 mg kg−1, ethanol extract). All the values are expressed as mean ± SE (n = 4). Different letters of the same protein indicate significant differences (p < 0.05). | |
The expression levels of several liver inflammation-related proteins, including cytochrome p450-2e1 (CYP2E1), P38, phosphorylated P38 (p-P38), p-NF-κB, inducible nitric oxide synthase (iNOS), and cyclooxygenase-2 (COX-2), are presented in Fig. 4A. The levels of these six proteins in group M were significantly upregulated compared with those in group C (p < 0.05). Only the relative expression of CYP2E1 in group EL was significantly lower than that in group M (p < 0.05), whereas the levels of the other five proteins in group EL did not exhibit significant differences from those in group M (p > 0.05). The expression levels of all these proteins in group EH significantly improved compared with those in group M (p < 0.05). p-NF-κB, as a key protein in regulating the transcription of cellular inflammation-related genes, must enter the nucleus to exert its bioactivity.17 As shown in Fig. 4B, a few p-NF-κB proteins entered the nucleus in group C, whereas many p-NF-κB proteins entered the nucleus in group M. The extract treatment evidently reduced the number of p-NF-κB proteins entering the nucleus.
Apoptosis was first detected using TUNEL assay. As shown in Fig. 5A, almost no TUNEL-positive signal was observed in group C, whereas many TUNEL-positive signals appeared in group M. After treatment with the extract, groups EL and EH showed a downward trend. Thereafter, the expression levels of three key proteins involved in cell apoptosis, namely, caspase-3, BCL2-associated X (Bax) and B-cell lymphoma-2 (Bcl-2), were investigated. The results are shown in Fig. 5B. Caspase-3 protein was observed with two strips, which were pro-caspase-3 (caspase-3 U; top strip) and cleaved caspase-3 (caspase-3 D; bottom strip). This result was consistent with previous findings.18 There was no significant difference in the expression level of caspase-3 U protein in all groups (p > 0.05). The expression levels of caspase-3 D, Bax, and Bcl-2 proteins significantly changed in group M compared with those in group C (p < 0.05), and the expression levels of these proteins in groups EL and EH were significantly different from those in group M (p < 0.05). Regardless of the dose, extract treatment significantly downregulated the expression levels of caspase-3 D and Bax and upregulated the expression level of Bcl-2 in comparison with those in group M (p < 0.05). Moreover, although the expression of caspase-3 D was not significantly different in the groups EL and EH (p > 0.05), the expression of caspase-3 D in group EH showed no significant difference from that in group C (p > 0.05), whereas the expression level of this protein in group EL was significantly higher than that in group C (p < 0.05).
4. Discussion
Excessive high-fat diet can lead to disorders of lipid metabolism in the liver and abnormal accumulation of lipids in the liver.8,19 The main characteristics of abnormal lipid accumulation in the liver with NAFLD are increased liver coefficient and fat color of the liver, and increased TG and TC contents in the body. Table 1 and Fig. 1A show that the liver coefficient of group M was significantly increased (p < 0.05), and the appearance of the liver became creamy white. Meanwhile, the liver coefficient of group EH decreased significantly compared with that of group M (p < 0.05), and the liver became rosy and smooth. As seen in Table 1, the TG and TC levels in the plasma and the liver of group M significantly increased (p < 0.05). The TG and TC contents in groups EH and EL significantly improved (p < 0.05). AST and ALT are common indicators that are used for evaluating liver damage and liver function and are also indicators of NAFLD.2 Elevated AST and ALT levels indicate that liver damage occurred in NAFLD rats. Compared with AST, ALT is a more sensitive indicator of liver injury.13,20 The ALT content in group M was about thrice higher than that in group C. Therefore, the liver of group M was speculated to have undergone severe damage. However, the AST and ALT levels in group EH were significantly improved (p < 0.05), thereby suggesting that the hepatocyte damage of NAFLD rats was improved effectively by the extract. These results were also proven by H&E staining. H&E staining can help observe the characteristics of NAFLD and more intuitively evaluate the protective effect of the extract on the liver. The results in Fig. 1B and C show that the liver tissues of group C were normal with clear cytoplasm, a normal nuclear morphology, and a suitable Diss cavity. The cytoplasm is the main site of physiological and biochemical reactions. The presence of cytoplasmic vacuoles may result in the loss of most cellular functions.13,21 Nuclear shrinkage is a precursor of apoptosis.22 The Diss cavity is the main structure for the exchange of liver cells with external nutrients, and if the cavity becomes smaller or disappears, it means that the exchange of materials is seriously hindered.9,23 The results in Fig. 1B and C show that severe damage of the liver cells and overaccumulation of lipid droplets in hepatocytes are observed in group M, whereas all abnormal conditions of hepatocytes in group EH significantly improved. According to these data, the Chinese sumac fruit extract can effectively improve the overaccumulation of lipid droplets in the liver and protect the liver from damage, thereby preventing NAFLD induced by the high-fat diet.
An excessive high-fat diet induces excessive ROS in the liver of NAFLD rats. Excessive ROS can induce lipid peroxidation, deplete the endogenous antioxidant enzymes, damage the cell membrane, and eventually lead to severe liver cell damage.7,9,24,25 One of the main products of lipid peroxidation is MDA, which exacerbates cell membrane damage and induces crosslinking polymerization of proteins and nucleic acids, resulting in the loss of the active function of these biomacromolecules.26 The endogenous antioxidant system is composed of CAT, GSH, and SOD. These antioxidants can effectively inhibit the damage caused by excessive ROS and MDA to the cells, thereby protecting the cells.24–26Table 1 shows that group M had a significantly higher MDA content and significantly lower endogenous antioxidant enzymes (CAT, GSH, and SOD) than group C (p < 0.05). A large amount of ROS may have been produced in the NAFLD rats, thereby depleting a large amount of endogenous antioxidant enzymes and producing a large amount of lipid peroxidation MDA. Group EH had a significantly decreased MDA content and significantly increased endogenous antioxidant enzymes (CAT, GSH, and SOD) compared with group M (p < 0.05). Based on the above data, it is found that the Chinese sumac fruit extract may partly strengthen the endogenous antioxidant system to reduce MDA and to resist the attack of ROS in liver cells, thereby eventually protecting liver cells and preventing NAFLD.
The pathogenesis of NAFLD, as a complex metabolic disease, is often related to abnormal lipid metabolism, inflammation, and apoptosis.27–29 The present work investigated the potential mechanisms of the Chinese sumac fruit extract in preventing NAFLD by analyzing the expression levels of the main proteins involved in lipid metabolism, inflammation, and apoptosis. A long-term high-fat diet can lead to changes in the expression levels of AMPK, p-AMPK, PPAR-α, CPT1, FAS, and PPAR-γ proteins.2,9 AMPK is a key protein in energy regulation, and p-AMPK can affect liver lipid metabolism by increasing fatty acid oxidation to reduce lipid synthesis.30–32 Previous studies have found that PPAR-α can also increase fatty acid oxidation by inducing CPT1 expression.33 CPT1 and FAS are downstream proteins of the AMPK signalling pathway.34 CPT1, which transports fatty acids into the mitochondria for β-oxidation, is a rate-limiting enzyme in the β-oxidation of free fatty acids.35 The protein of FAS is a key enzyme in the de novo synthesis of fatty acids, and its overexpression results in the synthesis of large amounts of fatty acids.9,36 The results of Fig. 2 and 3 showed that group M had significantly decreased p-AMPK, PPAR-α, and CPT expression levels and a significantly increased FAS content (p < 0.05), which indicated that the synthesis of a large amount of fatty acids was accompanied by low oxidation of fatty acids and resulted in the overaccumulation of lipid droplets in liver cells to cause NAFLD in group M. However, the administration of the extract, especially at a high dose (group EH), significantly improved the expression levels of these proteins to reduce the accumulation of lipid droplets in hepatocytes. PPAR-γ is an important cell differentiation transcription factor that regulates the expression of cluster of differentiation 36, sterol regulatory element-binding protein 1c, and FAS. It increases lipid absorption and synthesizes fat.2,37,38 It ultimately promotes lipid accumulation in the liver. Fig. 3 shows that the expression level of PPAR-γ may be partly responsible for overaccumulation of fat droplets in group M as shown in the histopathological results in Fig. 1, with a significant increase in the liver of group M (p < 0.05). The relative expression levels of PPAR-γ in groups EL and EH significantly decreased (p < 0.05), thereby indicating that the extract may also effectively suppress the expression of PPAR-γ to inhibit the accumulation of fat droplets in the liver cells. Previous studies have shown that the formation of fat droplets in the liver was closely related to the expression of PPAR-γ protein.39–41 However, some studies of NAFLD found that the expression of PPAR-γ protein in the model group decreased evidently.42 These seemingly contradictory results may be due to the different stages of NAFLD. In the early stage of NAFLD, hepatocytes need to protect themselves from excess free fatty acids by synthesizing large amounts of TG, but in the late stage, inflammation responses and/or the process of liver fibrosis greatly downregulate the expression of the PPAR-γ protein.43,44 It can be inferred that the Chinese sumac fruits effectively improved the lipid metabolism disorder of NAFLD by reducing fatty acid synthesis, increasing fatty acid oxidation, and inhibiting lipogenesis.
Excessive inflammation is considered one of the causes of NAFLD, and suppressing inflammation has a beneficial effect in improving NAFLD.27,28 Inflammation was caused by oxidative stress (ROS) induced by a high-fat diet.28 Many fatty acids that accumulate due to the lipid metabolism disorder serve as inducers of CYP2E1.45 As seen in group M (Fig. 4), when CYP2E1 was overexpressed, the cells produced large amounts of ROS. Biochemical results showed that significantly reduced endogenous antioxidant enzymes could not effectively scavenge ROS in group M.27,28 The large amount of ROS stimulates liver cells to induce a series of inflammation in the liver of NAFLD rats.24,25 The p-P38 protein is involved in ROS-stimulated cellular inflammatory responses and plays an important role in regulating p-NF-κB, COX-2, and iNOS. The expression of this protein increased significantly in group M (Fig. 4A). The transfer of the p-NF-κB protein in cells from the cytoplasm to the nucleus can regulate the expression of downstream proteins COX-2 and iNOS.46Fig. 4A clearly shows that the content of p-NF-κB protein was significantly increased in group M (p < 0.05). However, p-NF-κB must enter the nucleus to bind to nuclear DNA to promote the expression levels of downstream inflammatory proteins. The expression level of p-NF-κB protein observed from the western blot experiment did not accurately reflect whether p-NF-κB protein entered the nucleus. The immunofluorescence result in Fig. 4B accurately shows whether the p-NF-κB protein metastasized to the nucleus to aggravate the inflammatory response, thereby showing that a high number of p-NF-κB protein in group M entered the nucleus. COX-2 is an inducible enzyme. The activity of COX-2 in normal tissue is extremely low, and when stimulated by external and/or internal factors, the expression level of COX-2 is extremely high, eventually leading to inflammation and tissue damage.46 Meanwhile, iNOS overexpression increases the synthesis of NO, which can promote the inflammation and expression of COX-2.46 As shown in Fig. 4, the long-term high-fat diet significantly stimulated the expression levels of COX-2 and iNOS proteins in the livers of rats, which may eventually lead to serious inflammation of the liver and exacerbate the progression from NAFLD to liver fibrosis, cirrhosis, and liver cancer, in group M. All these inflammatory regulatory proteins in group EH were significantly suppressed compared with those in group M (p < 0.05). The effective suppression of p-NF-κB, iNOS, and COX-2 may be beneficial in improving NAFLD.7,29 Therefore, it is speculated that the Chinese sumac fruit extract can effectively inhibit the occurrence of inflammation to ameliorate NAFLD in rats.
Recent studies have also pointed out that lipid-induced apoptosis of hepatocytes is associated with the pathogenesis of NAFLD.7,27,29 When cells undergo apoptosis, DNA is cleaved to expose 3′-OH, which can be labeled with fluorescein under the catalysis of the terminal deoxynucleotidyl transferase.16Fig. 5A shows that there was a large amount of the TUNEL-positive signal in the nucleus of group M. However, the TUNEL-positive signal in the nucleus of the extract-administered group, especially in that of the EH group, was significantly reduced, indicating that the extract improved hepatocyte apoptosis in NAFLD. The expression levels of several apoptosis-related proteins were determined to investigate the underlying mechanism of the antiapoptotic effect of the phenolic-rich extract in NAFLD. Bax protein, as a proapoptotic protein, transfers to the mitochondrial outer membrane to open the mitochondrial permeability transition pore to induce cell apoptosis.47 However, Bcl-2 can inhibit Bax-mediated mitochondrial membrane protein channel formation to suppress cell apoptosis.47 Moreover, caspase-3, whose expression indicates the occurrence of apoptosis, is also the most important terminal cleavage enzyme in the process of apoptosis, and only cleaved caspase-3 can promote cell apoptosis.6,18 The results in Fig. 5 show that the relative level of Bax protein in group M was remarkably increased (p < 0.05), whereas the content of Bcl-2 protein decreased significantly (p < 0.05). Meanwhile, the expression of caspase-3 D protein, which was cleaved from caspase-3, increased remarkably in group M (p < 0.05). Severe lipid-induced apoptosis occurred in group M. Meanwhile, after being administered with the extract, group EH had significantly decreased expression levels of caspase-3 D and Bax proteins compared with group M (p < 0.05), and the expression levels of Bcl-2 protein in groups EL and EH increased remarkably (p < 0.05). Therefore, the Chinese sumac fruit extract can effectively protect hepatocytes against apoptosis induced by a high-fat diet to alleviate NAFLD. Many previous studies have also reported that improved lipid-induced apoptosis of hepatocytes can effectively ameliorate NAFLD.29,48 The main metabolic pathways of NAFLD investigated in the present work are outlined in Fig. 6.
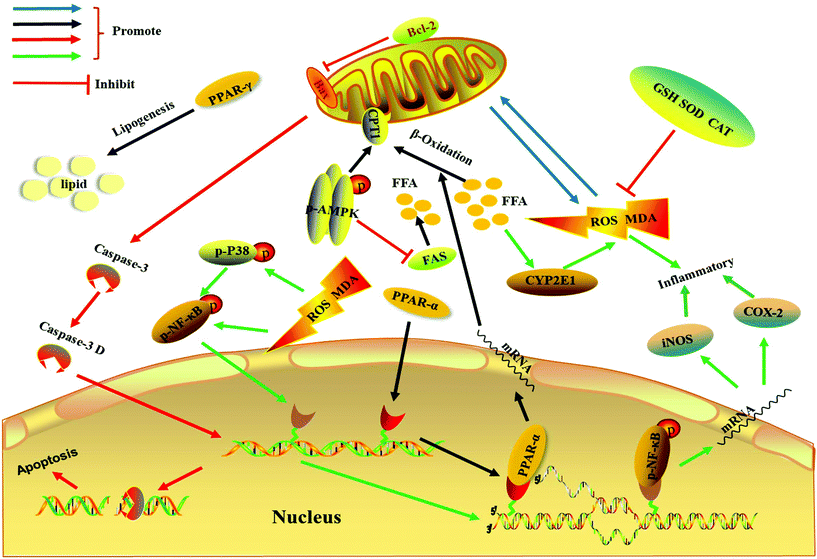 |
| Fig. 6 Schematic diagram of the main metabolic pathways of NAFLD investigated in the present work. SOD: superoxide dismutase; CAT: catalase; GSH: reduced glutathione; FFA: free fatty acid; ROS: reactive oxygen species; MDA: malonaldehyde; p-AMPK: phosphorylated amp-activated protein kinase; PPAR-α: peroxidase proliferative receptor-α; PPAR-γ: peroxidase proliferative receptor-γ; CYP2E1: cytochrome p450-2e1; CPT1: carnitine palmitoyl transferase-1; p-P38: phosphorylated P38 mitogen-activated protein kinase; p-NF-κB: phosphorylated nuclear factor κB; iNOS: inducible nitric oxide synthase; COX-2: cyclooxygenase-2; caspase-3: cysteinyl aspartate specific proteinase-3; caspase-3 D: cleaved cysteinyl aspartate specific proteinase-3; Bax: BCL2-associated X; Bcl-2: B-cell lymphoma-2; and FAS: fatty acid synthase. | |
It is found that the bioavailability, distribution and metabolism of polyphenols in the body play an important role in exerting their bioactivities.49,50 Thus, further studies are needed to comprehensively investigate the bioavailability, distribution and metabolism of different polyphenols in the extract of R. chinensis Mill. fruits. Our previous study on the toxicology of the extract has reported that the phenolic-rich extract of R. chinensis Mill. fruits did not show toxicity at a daily intake of 625 mg kg−1 when evaluated from subchronic toxicity in SD rats.13 According to this result, the high dose (600 mg kg−1) used in this study is safe for rats. However, the safe dose for humans needs to be further determined. Moreover, the present results indicate that the high dose of the extract was more effective at preventing NAFLD in rats. However, due to species differences, whether this dose is fully effective in humans in preventing NAFLD also requires further large-scale human experiments to confirm.
5. Conclusions
The phenolic-rich extract from Chinese sumac fruits can effectively prevent NAFLD in rats induced by a high-fat diet via regulating several key protein expression levels of multiple signalling pathways, especially at a high dose (EH, 600 mg of extract per kg of body weight and about 251.23 mg of TPC per kg of body weight). The phenolic-rich extract greatly improved the endogenous antioxidant system, including GSH, CAT, and SOD, to protect the hepatocytes from excessive oxidation caused by the high-fat diet in NAFLD rats. Immunofluorescence and TUNEL assay results suggested that the phenolic-rich extract evidently inhibited hepatocyte apoptosis and reduced the content of p-NF-κB in hepatocyte nuclei to suppress the inflammatory response. The extract may improve lipid metabolism, inflammation, and apoptosis of hepatocytes to prevent NAFLD. Lipid metabolism was controlled by upregulating the expression levels of p-AMPK, PPAR-α, and CPT1 proteins and by downregulating the levels of PPAR-γ protein. Inflammation was manipulated by downregulating the expression levels of CYP2E1, p-P38, p-NF-κB, iNOS, and COX-2 proteins. Hepatocyte apoptosis was prevented by upregulating the level of Bcl-2 and downregulating the expression levels of caspase-3D and Bax. Therefore, the extract may be used as a new natural source for exploiting functional foods and/or nutraceuticals to effectively prevent NAFLD.
Abbreviation
NAFLD | Non-alcoholic fatty liver disease |
TG | Triglyceride |
TC | Total cholesterol |
ALT | Alanine aminotransferase |
MDA | Malondialdehyde |
BCA | Bicinchoninic acid |
AST | Aspartate transaminase |
SOD | Superoxide dismutase |
CAT | Catalase |
GSH | Reduced glutathione |
p-AMPK | Phosphorylated amp-activated protein kinase |
AMPK | Amp-activated protein kinase |
SREBP-1 | Sterol regulatory element-binding protein1 |
FAS | Fatty acid synthase |
PPAR-α | Peroxidase proliferative receptor-α |
CPT1 | Carnitine palmitoyl transferase-1 |
PPAR-γ | Peroxidase proliferative receptor-γ |
CYP2E1 | Cytochrome p450-2e1 |
ROS | Reactive oxygen species |
p-P38 | Phosphorylated P38 mitogen-activated protein kinase |
P38 | P38 mitogen-activated protein kinase |
p-NF-κB | Phosphorylated nuclear factor κB |
iNOS | Inducible nitric oxide synthase |
COX-2 | Cyclooxygenase-2 |
Caspase-3 | Cysteinyl aspartate specific proteinase-3 |
Bax | BCL2-associated X |
Bcl-2 | B-cell lymphoma-2 |
Conflicts of interest
There are no conflicts of interest to declare.
Acknowledgements
The present work was financially supported by the National Natural Science Foundation of China (Grant No. 31660461 and 81760812) and the Yunnan Provincial Science and Technology Department-Applied Basic Research joint Special Funds of Yunnan University of Chinese Medicine (2017FF117(-035) and 2018FF001(-021)).
References
- T. Ren, L. Zhu, Y. Shen, Q. Mou, T. Lin and H. Feng, Protection of hepatocyte mitochondrial function by blueberry juice and probiotics via SIRT1 regulation in non-alcoholic fatty liver disease, Food Funct., 2019, 10, 1540–1551 RSC.
- J. Gao, J. Song, M. Du and X. Mao, Bovine α-lactalbumin hydrolysates (α-LAH) attenuate high-fat diet induced nonalcoholic fatty liver disease by modulating hepatic lipid metabolism in C57BL/6J mice, J. Funct. Foods, 2019, 54, 254–262 CrossRef CAS.
- M. Kwon, S. J. Lim, E. J. Joung, B. Lee, C. W. Oh and H. R. Kim, Meroterpenoid-rich fraction of an ethanolic extract from Sargassum serratifolium alleviates obesity and non-alcoholic fatty liver disease in high fat-fed C57BL/6J mice, J. Funct. Foods, 2018, 47, 288–298 CrossRef CAS.
- J. S. Li, W. J. Wang, Y. Sun, Y. H. Zhang and L. Zheng, Ursolic acid inhibits the development of nonalcoholic fatty liver disease by attenuating endoplasmic reticulum stress, Food Funct., 2015, 6, 1643–1651 RSC.
- Q. Qin, J. Niu, Z. Wang, W. Xu, Z. Qiao and Y. Gu, Astragalus membranaceus Inhibits inflammation via phospho-P38 mitogen-activated protein kinase (MAPK) and nuclear factor (NF)-κB pathways in advanced glycation end product-stimulated macrophages, Int. J. Mol. Sci., 2012, 13, 8379–8387 CrossRef CAS PubMed.
- F. F. Ahmed, A. A. El-Hafeez, S. H. Abbas, D. Abdelhamid and M. Abdel-Aziz, New 1,2,4-triazole-chalcone hybrids induce caspase-3 dependent apoptosis in A549 human lung adenocarcinoma cells, Eur. J. Med. Chem., 2018, 151, 705–722 CrossRef CAS PubMed.
- J. Zhou, C. T. Ho, P. Long, Q. Meng and X. Wan, Preventive efficiency of green tea and its components on non-alcoholic fatty liver disease, J. Agric. Food Chem., 2019, 67, 5306–5317 CrossRef CAS PubMed.
- M. Xu, C. Ge, Y. Qin, T. Gu, J. Lv, S. Wang, Y. Ma, D. Lou, Q. Li and L. Hu, Activated TNF-α/RIPK3 signaling is involved in prolonged high fat diet-stimulated hepatic inflammation and lipid accumulation: inhibition by dietary fisetin intervention, Food Funct., 2019, 10, 1302–1316 RSC.
- Z. Wu, Y. Ma, X. Gong, Y. Zhang, L. Zhao, G. Cheng and S. Cai,
Rhus chinensis Mill. fruits prevent high-fat/ethanol diet-induced alcoholic fatty liver in rats via AMPK/SREBP-1/FAS signaling pathway, J. Funct. Foods, 2019, 61, 103498 CrossRef CAS.
- O. Djakpo and W. Yao,
Rhus chinensis and Galla Chinensis–folklore to modern evidence, Phytother. Res., 2010, 24, 1739–1747 CrossRef CAS PubMed.
- C. Zhang, Y. Ma, F. Gao, Y. Zhao, S. Cai and M. Pang, The free, esterified, and insoluble-bound phenolic profiles of Rhus chinensis Mill. fruits and their pancreatic lipase inhibitory activities with molecular docking analysis, J. Funct. Foods, 2018, 40, 729–735 CrossRef.
- C. Zhang, Y. Ma, Y. Zhao, Y. Hong, S. Cai and M. Pang, Phenolic composition, antioxidant and pancreatic lipase inhibitory activities of Chinese sumac (Rhus chinensis Mill.) fruits extracted by different solvents and interaction between myricetin-rhamnoside and quercetin-rhamnoside, Int. J. Food Sci. Technol., 2018, 53, 1045–1053 CrossRef CAS.
- Z. Wu, Y. Ma, L. Zhao, S. Cai and G. Cheng, Acute and sub-chronic toxicities of the ethanol and hot-water extracts from Chinese sumac (Rhus chinensis Mill.) fruits by oral administration in rats, Food Chem. Toxicol., 2018, 119, 14–23 CrossRef CAS PubMed.
- C. C. Tang, W. L. Lin, Y. J. Lee, Y. C. Tang and C. J. Wang, Polyphenol-rich extract of Nelumbo nucifera leaves inhibits alcohol-induced steatohepatitis via reducing hepatic lipid accumulation and anti-inflammation in C57BL/6J mice, Food Funct., 2014, 5, 678–687 RSC.
- W. W. Franke, B. Appelhans, E. Schmid, C. Freudenstein, M. Osborn and K. Weber, Identification
and characterization of epithelial cells in mammalian tissues by immunofluorescence microscopy using antibodies to prekeratin, Differentiation, 2010, 15, 7–25 CrossRef PubMed.
- A. Tekcan, S. Tural, M. Elbistan, T. Guvenc, B. Ayas and N. Kara, Evaluation of apoptotic cell death on liver and kidney tissues following administration of levetiracetam during prenatal period, J. Matern.-Fetal Neonat. Med., 2016, 30, 1–4 Search PubMed.
- L. X. Du, Y. Q. Wang, G. Q. Hua and W. L. Mi, IL-33/ST2 pathway as a rational therapeutic target for CNS diseases, Neuroscience, 2018, 369, 222–230 CrossRef CAS PubMed.
- J. J. Lin, C. C. Huang, Y. L. Su, H. L. Luo, N. L. Lee, M. T. Sung and Y. J. Wu, Proteomics analysis of tangeretin-induced apoptosis through mitochondrial dysfunction in bladder cancer cells, Int. J. Mol. Sci., 2019, 20, 1017 CrossRef CAS PubMed.
- M. Katsumura, S. Takagi, H. Oya, S. Tamura, T. Saneyasu, K. Honda and H. Kamisoyama, Effects of dietary heme iron and exercise training on abdominal fat accumulation and lipid metabolism in high-fat diet-fed mice, Anim. Sci. J., 2017, 88, 1100–1106 CrossRef CAS PubMed.
- D. X. Lin, D. Parks, J. Painter, C. M. Hunt, H. A. Stirnadel-Farrant, J. Cheng, A. Menius and K. Lee, Validation of multivariate outlier detection analyses used to identify potential drug-induced liver injury in clinical trial populations, Drug Saf., 2012, 35, 865–875 CrossRef PubMed.
- C. M. Crump, C. Yates and T. Minson, Herpes simplex virus Type 1 cytoplasmic envelopment requires functional Vps4, J. Virol., 2007, 81, 7380–7387 CrossRef CAS PubMed.
- T. Oami, E. Watanabe, M. Hatano, Y. Teratake, L. Fujimura, A. Sakamoto, C. Ito, K. Toshimori, P. E. Swanson and S. Oda, Blocking liver autophagy accelerates apoptosis and mitochondrial Injury in hepatocytes and reduces time to mortality in a murine sepsis model, Shock, 2017, 50, 427–434 CrossRef PubMed.
- Y. S. Liao, L. W. Lee, P. H. Yang, L. M. Kuo, L. Y. Kuan, W. Y. Tseng and D. Hwang, Assessment of liver cirrhosis for patients with Child's A classification before hepatectomy using dynamic contrast-enhanced MRI, Clin. Radiol., 2019, 74, 407 CrossRef PubMed.
- C. P. Oliveira, L. C. Gayotto, C. Tatai, B. I. Della Nina, E. S. Lima, D. S. Abdalla, F. P. Lopasso, F. R. Laurindo and F. J. Carrilho, Vitamin C and vitamin E in prevention of nonalcoholic fatty liver disease (NAFLD) in choline deficient diet fed rats, Nutr. J., 2003, 2, 9 CrossRef PubMed.
- S. Krifka, G. Spagnuolo, G. Schmalz and H. Schweikl, A review of adaptive mechanisms in cell responses towards oxidative stress caused by dental resin monomers, Biomaterials, 2013, 34, 4555–4563 CrossRef CAS PubMed.
- M. Su, C. Y. Li, L. Zhou, Y. Yan, L. Ao, G. J. Wang, W. Fang and Y. M. Li, Anti-ulcerogenic effect of KFP-H008 against ethanol-induced gastric ulcer via p38 MAPK/NF-κB pathway, RSC Adv., 2017, 7, 49423–49435 RSC.
- A. Alisi, G. Carpino, F. L. Oliveira, N. Panera, V. Nobili and E. Gaudio, The role of tissue macrophage-mediated inflammation on NAFLD pathogenesis and its clinical implications, Mediators Inflammation, 2017, 2017, 8162421 CrossRef PubMed.
- C. Pirozzi, A. Lama, R. Simeoli, O. Paciello, T. B. Pagano, M. P. Mollica, F. Di Guida, R. Russo, S. Magliocca and R. B. Canani, Hydroxytyrosol prevents metabolic impairment reducing hepatic inflammation and restoring duodenal integrity in a rat model of NAFLD, J. Nutr. Biochem., 2016, 30, 108–115 CrossRef CAS PubMed.
- E. Buzzetti, M. Pinzani and E. A. Tsochatzis, The
multiple-hit pathogenesis of non-alcoholic fatty liver disease (NAFLD), Metabolism, 2016, 65, 1038–1048 CrossRef CAS PubMed.
- S. Ducommun, M. Deak, D. Sumpton, R. J. Ford, A. N. Galindo, M. Kussmann, B. Viollet, G. R. Steinberg, M. Foretz and L. Dayon, Motif affinity and mass spectrometry proteomic approach for the discovery of cellular AMPK targets: identification of mitochondrial fission factor as a new AMPK substrate, Cell. Signalling, 2015, 27, 978–988 CrossRef CAS PubMed.
- B. K. Smith, K. Marcinko, E. M. Desjardins, J. S. Lally, R. J. Ford and G. R. Steinberg, Treatment of nonalcoholic fatty liver disease: role of AMPK, Am. J. Physiol.: Endocrinol. Metab., 2016, 311, E730–E740 CrossRef PubMed.
- K.-T. Lin, S.-W. Hsu, F.-Y. Lai, T.-C. Chang, L.-S. Shi and S.-Y. Lee, Rhodiola crenulata extract regulates hepatic glycogen and lipid metabolism via activation of the AMPK pathway, BMC Complementary Altern. Med., 2016, 16, 127 CrossRef PubMed.
- Z. J. Brown, Q. Fu, C. Ma, M. Kruhlak, H. Zhang, J. Luo, B. Heinrich, S. J. Yu, Q. Zhang and A. Wilson, Carnitine palmitoyltransferase gene upregulation by linoleic acid induces CD4+T cell apoptosis promoting HCC development, Cell Death Discovery, 2018, 9, 620 CrossRef PubMed.
- X. Hu, L. Liu, Z. Song, A. Sheikhahmadi, Y. Wang and J. Buyse, Effects of feed deprivation on the AMPK signaling pathway in skeletal muscle of broiler chickens, Comp. Biochem. Physiol., Part B: Biochem. Mol. Biol., 2016, 191, 146–154 CrossRef CAS PubMed.
- H. Sudeep, K. Venkatakrishna, D. Patel and K. Shyamprasad, Biomechanism of chlorogenic acid complex mediated plasma free fatty acid metabolism in rat liver, BMC Complementary Altern. Med., 2016, 16, 274 CrossRef PubMed.
- Q. O. Yang, X. M. Lin, Y. J. Zhu, B. Zheng, L. Li, Y. C. Yang, G. J. Hou, X. Chen, G. J. Luo and F. Huo, Distinct role of nuclear receptor corepressor 1 regulated de novo fatty acids synthesis in liver regeneration and hepatocarcinogenesis in mice, Hepatology, 2018, 67, 1071–1087 CrossRef PubMed.
- M. Jin, Y. Wu, J. Wang, J. Chen, Y. Huang, J. Rao and C. Feng, MicroRNA-24 promotes 3 T3-L1 adipocyte differentiation by directly targeting the MAPK7 signaling, Biochem. Biophys. Res. Commun., 2016, 474, 76–82 CrossRef CAS PubMed.
- F. Salomone, J. Godos and S. Zelber, Natural antioxidants for non-alcoholic fatty liver disease: molecular targets and clinical perspectives, Liver Int., 2016, 36, 5–20 CrossRef CAS PubMed.
- C. Zhang, X. Luo, J. Chen, B. Zhou, M. Yang, R. Liu, D. Liu, H. F. Gu, Z. Zhu and H. Zheng, Osteoprotegerin Promotes Liver Steatosis by Targeting the ERK–PPAR-γ-CD36 Pathway, Diabetes, 2019, 68, 1902–1914 CrossRef PubMed.
- C. G. Dossi, C. Cadagan, M. San Martín, A. Espinosa, D. González-Mañán, D. Silva, R. A. Mancilla and G. S. Tapia, Effects of rosa mosqueta oil supplementation in lipogenic markers associated with prevention of liver steatosis, Food Funct., 2017, 8, 832–841 RSC.
- S.-H. Liu, C. Y. Chiu, L. H. Huang and M. T. Chiang, Resistant maltodextrin ameliorates altered hepatic lipid homeostasis via activation of AMP-activated protein kinase in a high-fat diet-fed rat model, Nutrients, 2019, 11, 291 CrossRef CAS PubMed.
- J. Zhong, W. Gong, L. Lu, J. Chen, Z. Lu, H. Li, W. Liu, Y. Liu, M. Wang and R. Hu, Irbesartan ameliorates hyperlipidemia and liver steatosis in type 2 diabetic db/db mice via stimulating PPAR-γ, AMPK/Akt/mTOR signaling and autophagy, Int. Immunopharmacol., 2017, 42, 176–184 CrossRef CAS PubMed.
- P. Guruvaiah, H. Guo, D. Li and Z. Xie, Preventive effect of flavonol derivatives abundant sanglan tea on long-term high-fat-diet-induced obesity complications in C57BL/6 Mice, Nutrients, 2018, 10, 1276 CrossRef PubMed.
- H. Y. Li, M. Yang, Z. Li and Z. Meng, Curcumin inhibits angiotensin II-induced inflammation and proliferation of rat vascular smooth muscle cells by elevating PPAR-γ activity and reducing oxidative stress, Int. J. Mol. Med., 2017, 39, 1307–1316 CrossRef CAS PubMed.
- T. Jian, X. Ding, Y. Wu, B. Ren, W. Li, H. Lv and J. Chen, Hepatoprotective effect of loquat leaf flavonoids in PM2. 5-induced non-alcoholic fatty liver disease via regulation of IRs-1/Akt and CYP2E1/JNK pathways, Int. J. Mol. Sci., 2018, 19, 3005 CrossRef PubMed.
- L. Tan, J. Li, Y. Wang and R. Tan, Anti-Neuroinflammatory Effect of alantolactone through the suppression of the NF-κB and MAPK signaling pathways, Cells, 2019, 8, 739 CrossRef PubMed.
- J. Q. Kwong and J. D. Molkentin, Physiological and pathological roles of the mitochondrial permeability transition pore in the heart, Cell Metab., 2015, 21, 206–214 CrossRef CAS PubMed.
- Y. H. Zhong, L. Y. Huan, W. Z. Yuan, Y. C. Huan and Y. Bing, Dietary quercetin ameliorates nonalcoholic steatohepatitis induced by a high-fat diet in gerbils, Food Chem. Toxicol., 2013, 52, 53–60 CrossRef PubMed.
- T. Bohn, G. J. McDougall, A. Alegría, M. Alminger, E. Arrigoni, A. M. Aura, C. Brito, A. Cilla, S. N. El, S. Karakaya, M. C. Martínez-Cuesta and C. N. Santos, Mind the gap-deficits in our knowledge of aspects impacting the bioavailability of phytochemicals and their metabolites-a position paper focusing on carotenoids and polyphenols, Mol. Nutr. Food Res., 2015, 59(7), 1307–1323 CrossRef CAS PubMed.
- K. Kawabata, R. Mukai and A. Ishisaka, Quercetin and related polyphenols: new insights and implications for their bioactivity and bioavailability, Food Funct., 2015, 6(5), 1399–1417 RSC.
|
This journal is © The Royal Society of Chemistry 2020 |