DOI:
10.1039/C9FO02240F
(Paper)
Food Funct., 2020,
11, 483-492
Solanum nigrum polyphenols reduce body weight and body fat by affecting adipocyte and lipid metabolism
Received
25th September 2019
, Accepted 20th November 2019
First published on 13th December 2019
Abstract
Obesity, being overweight and deposition of body fat are critically associated with metabolic disorders. The number of adipocytes and their lipid content, and the molecules involved in lipid metabolism are involved in obesity comorbidity. The food, Solanum nigrum L. (SN), has medical benefits in many aspects. In our recent report, SN was shown to reduce hepatic fat accumulation and oxidative stress, thus attenuating liver damage. However, it has not yet been explored whether SN is effective for weight loss and body fat reduction. Hence, we aimed to investigate if SN water extract (SWE) and the derived polyphenols (SNPE) are able to prevent obesity. Mice fed a high fat diet (HFD) and 3T3L1 cells model were used. The in vivo experiments showed SWE decreased serum triacylglyceride, cholesterol, and low-density lipoprotein (LDL)-cholesterol induced by a HFD. SWE promoted hepatic lipolysis by increasing PPARα and CPT-1, and inhibited lipogenesis by decreasing FaS and HMG-CoR. The expression of AMPK was enhanced, but sterol regulatory element binding proteins (SREBPs) were reduced by SWE, especially at 5%. In vitro analysis revealed that SNPE decreased the amount and lipid content of adipocytes. SNPE, especially at 0.5 mg mL−1, promoted lipolysis while inhibiting lipogenesis. In comparison with the doses applied in vivo and in vitro, the effect of SN could be attributed to the composition of the polyphenols. The results showed that SNPE is suggested to be an anti-obesity agent that is able to reduce body weight and body fat, by decreasing the amount and lipid content of adipocytes, and regulating lipid metabolism.
Introduction
Being overweight and obese, defined as having accumulated excess body fat which may impair health,1 are associated with metabolic syndrome and a great range of illnesses, including diabetes, cardiovascular disease, and non-alcoholic fatty liver. In recent years, it has been recognized that deposition of body fat plays a critical role in the pathogenesis of metabolic-related disorders.2 In particular, the regulation of liver lipids should be emphasized to prevent illnesses that accompany obesity. Lipid metabolism is biphasic-regulated via lipogenesis and lipolysis. Fatty acid synthase (FaS), glycerol-3-phosphate acyltransferase (GPAT) and 3-hydroxy-3-methylglutaryl-coenzyme A (HMG-CoA) reductase (HMG-CoR), the enzymes that regulate fatty acids, glycerolipids and cholesterol synthesis, respectively, have been indicated as markers of lipogenesis.3 On the other hand, carnitine palmitoyltransferase-1 (CPT-1) and peroxisome proliferator-activated receptor α (PPARα), are involved in the process of lipolysis.4 Furthermore, the number of adipocytes and their lipid content could be viewed as an indicator associated with inflammation, insulin resistance and obesity comorbidities.5,6
Sterol regulatory element binding protein (SREBP), which has the isoforms SREBP-1a, -1c and -2, is a transcription factor that regulates lipid metabolism.7 Inhibiting the maturation of SREBP-1 reduces the transcription of FaS and HMG-CoA reductase, while SREBP-2 is selectively involved in cholesterol synthesis.7,8 Many previous reports have suggested that SREBP is regulated by the AMP-activated protein kinase (AMPK). Alpha-lipoic acid increased the phosphorylation of AMPK, thus preventing insulin-stimulated SREBP-1c expression and development of NAFLD.9 Metformin, a type 2 DM therapeutic, decreases hepatic SREBP-1 via the regulating of AMPK.10
Solanum nigrum L. (SN) is a native plant that is abundantly grown in Asia. Used as an edible vegetable in China and India, SN has anti-inflammatory properties11 and can bring down a fever, protect the liver, reduce blood pressure, and enhance glucose tolerance.12,13 It has been demonstrated that SN reduces serum glucose and lipids in streptozotocin-induced diabetic rats.14 Recently, we reported that SN reduces serum triacylglycerides and cholesterol, attenuating hepatic fat accumulation and oxidative stress in mice fed a high fat diet (HFD. The SN polyphenol decreases FaS, HMG-CoA reductase, AMPK and SREBPs, while increasing CPT1 and PPARα in oleic acid-treated hepatocytes.15 According to the literature, SN is supposed to regulate lipid metabolism against related metabolic disorders. However, to the best of our knowledge, whether SN is effective for weight loss and body fat reduction has not yet been investigated.
Hence, in the present study, we aim to investigate whether a SN water extract (SWE) and its derived polyphenols (SNPE) are able to reduce body weight and body fat, and the putative mechanisms involved. The in vivo experiments using mice fed a HFD and in vitro 3T3L1 cell models were used to test the ability of SN.
Materials and methods
Preparation of SWE and SNPE
Solanum nigrum L. was collected from central Taiwan. The entire plant (including the stem, leaf, and root) was cut into pieces and immersed in water overnight. Afterwards, SN was collected, rinsed with boiled water, and then extracted using the following steps. SN was extracted by continuous heating at 100 °C for 40 min, and the process was repeated three times. The resulting water extract was then filtered, concentrated in a water bath at 90 °C, and dried in an oven at 70 °C. The obtained product is indicated as SWE. The composition of SWE was analyzed, and 18 components were identified in our previous report. Among them miquelianin (13.18 ± 0.99 μg mg−1), catechin (9.99 ± 0.53 μg mg−1), rutin (6.57 ± 0.52 μg mg−1), gallic acid (6.20 ± 0.53 μg mg−1), isoquercetin (4.28 ± 0.37 μg mg−1), and peltatoside (4.08 ± 0.35 μg mg−1) were found to be the major compounds. In addition, nuciferine, epicatechin, quercetin, and epigallocatechin gallate were also detected.15 To prepare the SNPE, 100 g of SN powder was mixed with 300 mL of ethanol, followed by heating at 50 °C for 3 h. The extract was filtered and lyophilized under reduced pressure at room temperature. The residue was then re-suspended in 500 mL of distilled water, extracted with 180 mL of ethyl acetate three times, re-dissolved in 250 mL of distilled water, stored at −70 °C overnight, and lyophilized. The composition of SNPE was analyzed. The contents (μg/0.1 mg) of catechin, rutin, and gallic acid were 2.03 ± 0.73, 1.81 ± 0.57, and 1.14 ± 0.47, respectively. Quercetin (2.60 ± 0.33), epicatechin (1.59 ± 0.26), and epigallocatechin gallate (1.43 ± 0.13) were also found in the composition of SNPE.
Animal experiments
Male C57BL/6 mice (25–30 g; aged 42 d) were purchased from Lasco Laboratory Animal Co. (Yilan County, Taiwan) and acclimatized for 7 d. All mice were housed under standard laboratory conditions (18–23 °C, humidity 55–60%, and a 12 h light/dark cycle) in agreement with animal protocols approved by the Institutional Animal Care and Use Committee of the Chung Shan Medical University (IACUC approval no. 740), Taichung, Taiwan. The animals were divided into five groups according to the diet (n = 12 per group): (A) control, (B) HFD, (C) HFD with 0.5% SNE, (D) HFD with 1% SNE, and (E) HFD group with 2% SNE. As shown in our previous report, lard and cholesterol were contained in the HFD at 20% and 2%, respectively.15 The body weights were recorded every week up to 10 weeks, thereafter the mice were sacrificed. The livers and body fat were collected for further examination. The serum sample was collected using ethylenediaminetetraacetic acid (EDTA) tubes and centrifuged at 3000 rpm (1400g) for 10 min at 4 °C. The concentrations of triglycerides (TGs), total cholesterol, low-density lipoprotein (LDL) cholesterol (LDL-C), high-density lipoprotein (HDL) cholesterol (HDL-C), aspartate aminotransferase (AST), alanine aminotransferase (ALT) were measured using enzymatic colorimetric methods using commercial kits (Randox Laboratories, Ltd, Antrim, UK). The analysis of the serum was carried out using an automatic analyzer (Olympus AU2700, Olympus Co., Tokyo, Japan).
Hematoxylin and eosin staining of the liver
Liver tissues were fixed in 4% paraformaldehyde overnight and embedded in paraffin using a routine procedure. Consecutive 4 mm paraffin sections were made, and processed for histological examination using conventional methods with hematoxylin and eosin (H&E) staining.
Cell culture and differentiation of adipocytes
The cell line 3T3-L1 (obtained from the American Type Culture Collection) was cultured in a proliferation medium composed of Dulbecco's modified Eagle's medium (4.5 g L−1 of glucose, pH 7.18–7.20), with 10% bovine calf serum (BCS), 1.5 g L−1 sodium bicarbonate, 2 mM L-glutamine, 1 mM sodium pyruvate, and an antibiotic/antimycotic mixture (100 U mL−1 penicillin G, 100 μg mL−1 streptomycin and 0.25 μg mL−1 amphotericin B) at 37 °C with 5% CO2. Cells were expanded in the 10 cm dish until they were sub-confluent (about 60%), and then harvested and seeded in a 6 well-plate (1 × 106 per well) until confluent.
The induction of differentiation took 12 d. Day 1–4: Cells were cultured in the proliferation medium with 4.5 μM rosiglitazone (RSG), 1.7 μM insulin, 0.25 μM dexamethasone (DEX) and 0.5 mM isobutylmethylxanthine (IBMX). Day 5–8: Cells were cultured in the proliferation medium with 1.7 μM insulin only. Day 9–12: Cells were cultured in the proliferation medium. After completing the process, the cells were found to be round and large under a microscope.
Cytotoxicity assay
Cells were seeded at a density of 2 × 105 cells per mL in a 24-well plate. After attachment, cells were incubated with SNPE at various concentrations for 24 or 48 h. The medium was then changed and cells were incubated with 3-(4,5-dimethylthiazol-2-yl)-2,5diphenyltetrazolium bromide (MTT, 0.5 mg mL−1) for 4 h. The viable cells were directly proportional to the production of formazan. Following the dissolution in isopropanol (1 mL per well), and centrifugation (at 12
000 rpm), each sample was added (200 μL per well) into a 96-well plate, then read with a spectrophotometer at 563 nm (Hitachi, U-3210, Tokyo, Japan).
Oil Red O staining
Cells were seeded at a density of 1 × 106 into a 10 cm dish. After attachment, cells were treated with different concentrations of SNPE for 24 h, washed with phosphate buffered saline (PBS), and fixed with 4% paraformaldehyde for 1 h. Thereafter, the samples were stained with 1 μg ml−1 of Oil Red O (Sigma-Aldrich, O0625) for 5 min, washed with PBS, excited at 563 nm of the laser beam (Hitachi 3210, Hitachi, Tokyo, Japan), and soon after observed using a microscope.
Western blot analysis
Liver pieces (0.5 g) were combined with radioimmunoprecipitation assay (RIPA) buffer (5 mL) and protein inhibitors, and homogenized at 4 °C. Tissue homogenates were centrifuged (10
000g for 20 min at 4 °C), and the resulting supernatant was used for western blot analysis. The total protein concentrations of the tissue extracts were determined through the Bradford protein assay kit (Bio-Rad, Hercules, CA, USA). In addition, cells were harvested into lysis buffer containing 50 mM Tris HCl (pH 6.8), 10% glycerol, 2% SDS, and 5% mercaptoethanol, and then lysed using sonication. Cell lysate was centrifuged at 9300g for 20 min at 4 °C, and the supernatant was collected as the protein sample. After quantification, equal amounts of the protein samples (50 μg) were subjected to 10% sodium dodecyl sulfate (SDS)-polyacrylamide gel electrophoresis and transferred to nitrocellulose membranes (Millipore, Bedford, MA, USA). Membranes were blocked with 5% nonfat milk powder with 0.1% Tween-20 in tris-buffered saline (TBS), and then incubated with the primary antibodies at 4 °C overnight. Antibodies against phospho-AMPK (AMPK-P) and SREBP-2 were purchased from Cell Signaling Technology (Beverly, MA, USA); antibodies against GPAT, FaS, SREBP-1, PPARα and CPT1 were obtained from Santa Cruz Biotechnology (Santa Cruz, CA, USA). The antibody for HMG-CoR was purchased from Upstate Biotechnology (Charlottesville, VA, USA). After this, membranes were washed three times with 0.1% Tween-20 in TBS and incubated with the secondary antibody (1
:
5000) conjugated to horseradish peroxidase (GE Healthcare, Little Chalfont, Buckinghamshire, UK). Band detection was thereafter revealed by enhanced chemiluminescence using ECL western blotting detection reagents and exposed in FUJFILM Las-3000 (Tokyo, Japan). The protein quantity was determined by densitometry using FUJFILM-Multi Gauge V2.2 software.
Statistical analysis
Data are reported as the mean ± standard deviation from three independent experiments. The statistical software SPSS v.12.0 was used to analyze the data. One-way analysis of variance (ANOVA) was performed (p < 0.05), while Bonferroni's multiple comparison was used for the post-test.
Results
Effect of SWE on body weight reduction
At two weeks, the body weight of the HFD mice was significantly higher compared with the control mice, and thereby consistently increased until the end of the experiment. At 8 and 10 weeks, the body weight of the HFD mice was almost 20% greater than the control group. Treatment with SWE significantly attenuated the increase in the body weight, especially at 2%, which reduced the body weight to almost the same level as the control group. Further analysis revealed the change in body weight was eliminated by SWE. At 2 weeks, only 2% of SWE was valid. At 8 and 10 weeks, all of the applied doses of SWE were effective at eliminating the HFD-induced body weight change (Fig. 1).
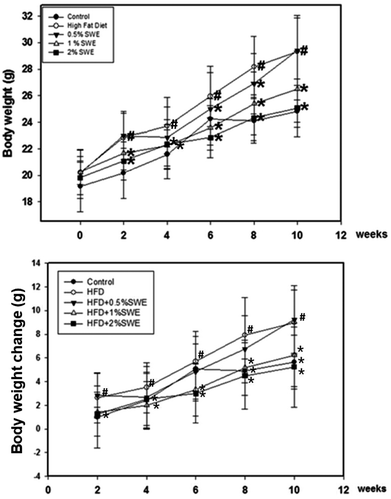 |
| Fig. 1 SWE reduces body weight in HFD-induced obesity. Data is presented as means ± SDs (n = 12 for each group). #p < 0.05, compared with control. *p < 0.05 compared with an HFD. | |
Effect of SWE on body fat reduction
The HFD increased the perirenal fat. Treatment with 1% and 2% SWE significantly reduced the perirenal fat by more than 40% and 55%, respectively. In addition, compared with the HFD group, the epididymis fat was reduced by 45% by 2% of SWE, even to the level of the control group. The body fat estimation revealed that the HFD increased the total body fat to approximately three-fold. Dose-dependent treatment of SWE was found to reduce the body fat (Fig. 2).
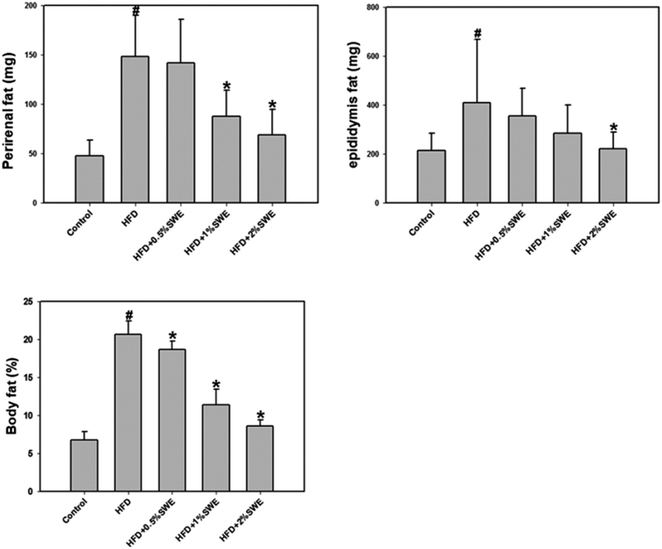 |
| Fig. 2 SWE decreases body fat in HFD-induced obesity. Data is presented as means ± SDs (n = 12 for each group). #p < 0.05, compared with control. *p < 0.05 compared with an HFD. | |
Effect of SWE on the serum lipid profile
Table 1 shows that an HFD increased serum triacylglyceride, cholesterol, and LDL-cholesterol. Treatment with SWE significantly improved the hyperlipidemia especially at a dose of 2%. The HFD also increased the serum HDL-cholesterol, while SWE recovered the level. It should be noted that although AST was not altered, treatment with SWE significantly recovered the HFD-increased ALT. Although the levels of AST and ALT are generally viewed as indicators for hepatic function, ALT is mainly generated by the liver.
Table 1 Serum lipid profile
|
Normal |
HFD |
HFD + 0.5%SWE |
HFD + 1.5%SWE |
HFD + 2%SWE |
Data are shown as means ± SD. #, P < 0.05, compared with the control *, P < 0.05, compared with a HFD. TG, triglyceride; TC, total cholesterol; HDL-C, high density lipoprotein cholesterol; LDL-C, low density lipoprotein cholesterol; AST, Aspartate aminotransferase; ALT, alanine transaminase. |
TG (mg dL−1) |
42.67 ± 2.5 |
74.22 ± 6.03# |
63.13 ± 11.78* |
64.33 ± 8.26* |
58 ± 3.46* |
TC (mg dL−1) |
105.2 ± 4.07 |
140.5 ± 9.99# |
134 ± 10.38 |
115.17 ± 11.7* |
103.75 ± 1.79* |
HDL-C (mg dL−1) |
63.33 ± 3.2 |
82.7 ± 5.1# |
84.91 ± 5.65 |
70.56 ± 3.69* |
55.86 ± 4.49* |
LDL-C (mg dL−1) |
8.67 ± 1.25 |
14.5 ± 1.66# |
12 ± 1.26* |
11.43 ± 1.4* |
9.57 ± 2.72* |
AST (IU L−1) |
118.33 ± 14.52 |
192.5 ± 34.85# |
171.83 ± 15.4 |
163.33 ± 24.14 |
157 ± 52.12 |
ALT (IU L−1) |
36 ± 1.14 |
52 ± 3.79# |
49.17 ± 4.41 |
41.57 ± 6.61* |
40.6 ± 6.22* |
Effect of SWE on hepatic fat accumulation
The histological examination revealed that HFD induced the occurrence of fatty liver, but SWE attenuated the pathogenesis in a dose-dependent manner (Fig. 3).
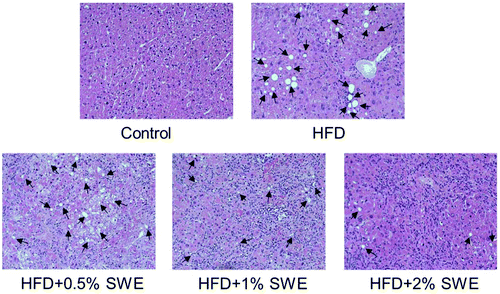 |
| Fig. 3 SWE decreases the hepatic lipid content. Mice were fed with different diets. After sacrifice, the livers were fixed, embedded, sectioned, and then stained with hematoxylin and eosin (original magnification: 200×). Arrows indicate lipid deposition. | |
Effect of SWE on in vivo lipid metabolism
Compared with the HFD, 1% and 2% of SWE significantly decreased the hepatic expression of GPAT by 40% and 45%, respectively. The HFD-induced expression of FaS and SREBP-1 were also attenuated by SWE. In contrast, the activation of AMPK was reduced by an HFD. Treatment with 2% of SWE significantly recovered the phosphorylation of AMPK, even above the level of the control (Fig. 4). Fig. 5 shows that the HFD increased the level of HMG-CoR to 1.25 fold, and 1% and 2% of SWE reduced the expression of HMG-CoR by 12% and 16%, respectively. In addition, treatment with SWE, especially at 2%, inhibited the level of SREBP-2 effectively. The hepatic expression of CPT-1 and PPARα revealed that all of the applied doses of SWE were able to promote lipolysis. As shown in Fig. 6, 2% of SWE stimulated the expression of CPT-1 to 1.4 fold. The HFD decreased PPARα by 40%. Treatment with 2% of SWE, not only recovered the level of PPARα, but it reached 1.3 fold compared with the control.
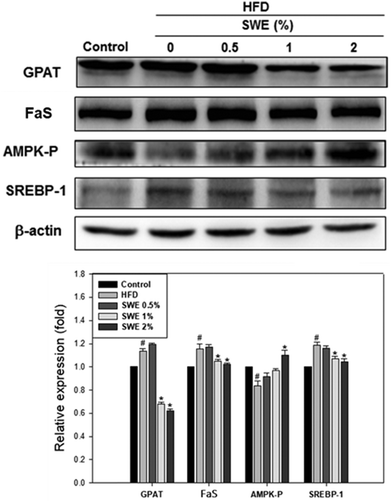 |
| Fig. 4 SWE decreases the hepatic expressions of GPAT, FaS and SREBP-1, while increasing the levels of AMPK-P. The protein samples were detected using western blot analysis and quantified by densitometric analysis. The results from three independent experiments are expressed as mean ± SD. #p < 0.05, compared with the control. *p < 0.05 compared with an HFD. | |
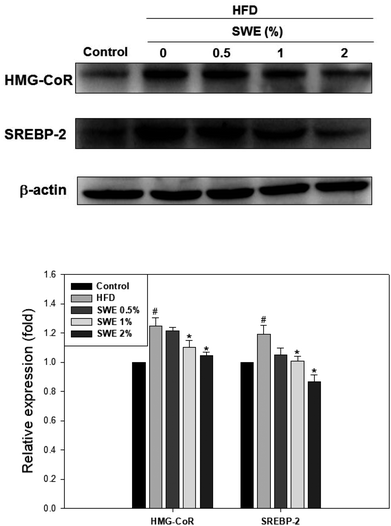 |
| Fig. 5 SWE decreases the hepatic expressions of HMG-CoR and SREBP-2 in the liver. The protein samples were detected using western blot analysis and quantified by densitometric analysis. The results from three independent experiments are expressed as mean ± SD. #p < 0.05, compared with control. *p < 0.05 compared with an HFD. | |
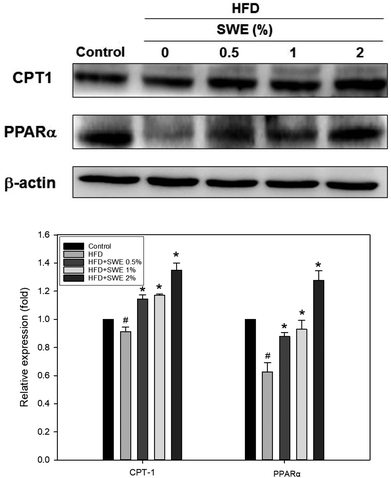 |
| Fig. 6 SWE increases the hepatic expressions of CPT-1 and PPARα in the liver. The protein samples were detected using western blot analysis and quantified by densitometric analysis. The results from three independent experiments are expressed as mean ± SD. #p < 0.05, compared with control. *p < 0.05 compared with an HFD. | |
Effect of SNPE on the viability of the adipocytes
The MTT analysis revealed that SNPE reduced the cell viability in a dose-dependent manner. It was shown that 0.3, 0.4, and 0.5 mg mL−1 of SNPE reduced the numbers of 3T3 cells to approximately 75%, 60% and 55%, respectively, after treatment for 24 h. Similar results were also found after SNPE treatment for 48 h (Fig. 7). To maintain the appropriate cell numbers for operation, 0.5 mg mL−1 was thus determined as the maximum dose and applied in the following experiment.
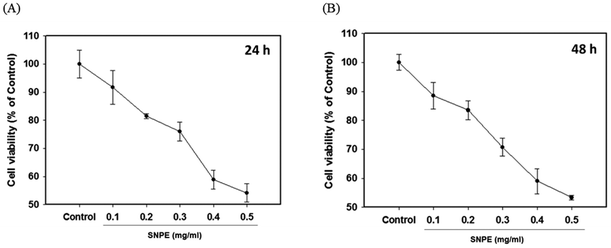 |
| Fig. 7 SNPE inhibits the viability of adipocytes. 3T3L1 cells were treated with various concentrations of SNPE for (A) 24 h and (B) 48 h, then analyzed using MTT. The data are expressed as mean ± SD from four samples for each group. | |
Effect of SNPE on in vitro lipid accumulation and lipid metabolism
Analysis using Oil Red O staining revealed that SNPE reduced the lipid accumulation in a dose-dependent manner in differentiated adipocytes (Fig. 8). In particular, at a dose of 0.5 mg mL−1, SNPE ameliorated all of the lipid contents to the level of pre-3T3L1. Compared with the pre-3T3L1, the protein level of FaS increased to four-fold in the 3T3L1 cells. Treatment of 0.3, 0.4, and 0.5 mg mL−1 of SNPE, attenuated the level of FaS and the expression of SREBP-1 in a dose-dependent manner. In contrast, the phosphorylation of AMPK was significantly elevated by SNPE, especially at the dose of 0.5 mg mL−1 (Fig. 9). In addition, treatment with SNPE reduced the level of HMG-CoR and SREBP-2 (Fig. 10). The expression of CPT-1 and PPARα were significantly enhanced by SWE, especially at 0.5 mg mL−1 (Fig. 11).
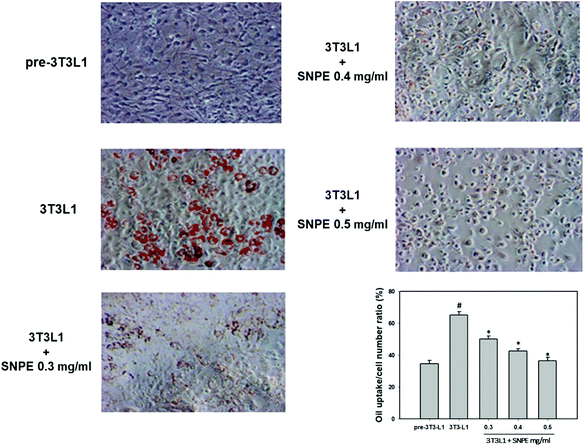 |
| Fig. 8 SNPE attenuates the lipid accumulation of adipocytes. Cells were treated with the indicated concentrations of SNPE, and then stained with Oil Red O. The data are mean ± SD from three samples for each group. #p < 0.05 compared with pre-3T3L1; *p < 0.05 compared with 3T3L1. | |
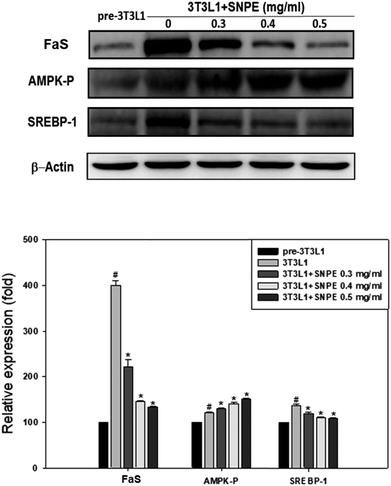 |
| Fig. 9 SNPE decreases the expression of FaS and SREBP-1, while increasing the level of AMPK-P in adipocytes. Proteins were detected using western blot analysis and quantified by densitometric analysis. The result from three independent experiments are expressed as mean ± SD. #p < 0.05 compared with pre-3T3L1; *p < 0.05 compared with 3T3L1. | |
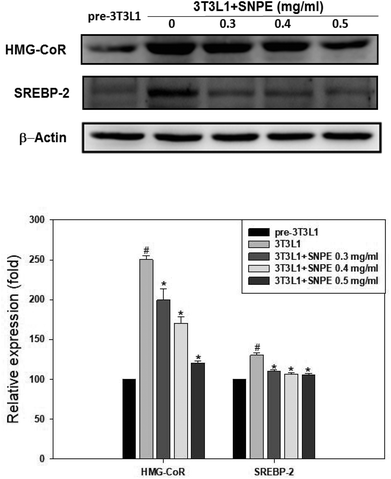 |
| Fig. 10 SNPE decreases the expression of HMG-CoR and SREBP-2 in adipocytes. Proteins were detected using western blot analysis and quantified by densitometric analysis. The result from three independent experiments are expressed as mean ± SD. #p < 0.05 compared with pre-3T3L1; *p < 0.05 compared with 3T3L1. | |
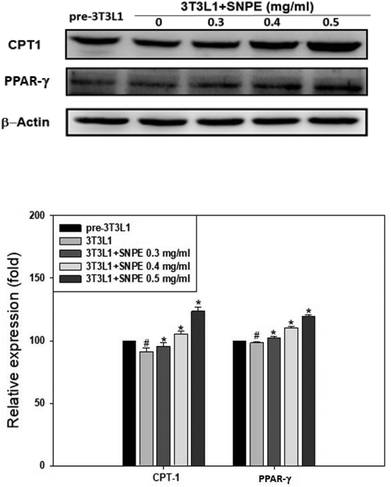 |
| Fig. 11 SNPE increases the expressions of CPT-1 and PPARα in adipocytes. Proteins were detected using western blot analysis and quantified by densitometric analysis. The results from three independent experiments are expressed as mean ± SD. #p < 0.05 compared with pre-3T3L1; *p < 0.05 compared with 3T3L1. | |
Discussion
Our in vivo experiments reveal that SWE is able to reduce body weight and body fat. SWE promotes lipolysis by increasing the level of PPARα and CPT-1, while inhibiting lipogenesis by decreasing the level of FaS and HMG-CoR. Being the critical molecules involved in the signals regulating lipid metabolism, the AMPK are enhanced, but the SREBPs are reduced by SWE. The in vitro experiments suggest that SNPE decreases the viability and lipid content of the adipocytes. Treatment with SNPE, especially at the dose of 0.5 mg mL−1 promotes lipolysis and inhibits lipogenesis. Compared with the in vivo findings, the effects of SN could be attributed to the composition of the polyphenols. All findings are illustrated in Fig. 12.
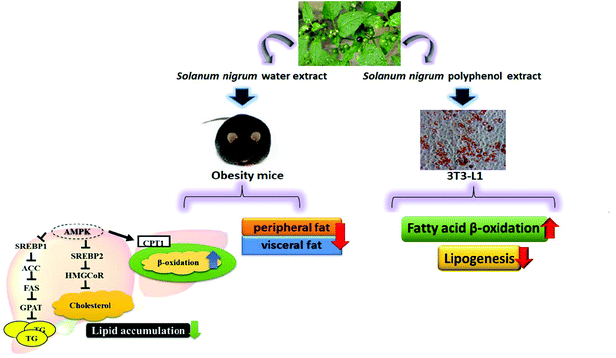 |
| Fig. 12 SN reduces body weight and body fat via regulating lipid accumulation, lipid metabolism and the viability of adipocytes. | |
With regard to the distribution of body fat, a controversy exists with regards to whether visceral or subcutaneous fat contributes to the related metabolic disorder. Some reports indicate that as a cause of peripheral insulin resistance, the abnormality in subcutaneous lipolysis could be more important than that in visceral lipolysis, because visceral fat accounts for a small percentage of the total body fat.16 However, in monosodium glutamate-obese rats, Kim et al. measured the fasting levels of serum insulin, glucose disappearance rate, and hepatic glucose production after surgical removal of visceral or subcutaneous fat tissue. The results suggested that visceral fat affects the level of FFA and insulin sensitivity more than subcutaneous fat.17 The redistribution of body fat by reducing the visceral fat, but increasing subcutaneous fat, could be more protective against metabolic syndrome.18 In the present study, we measured the alteration of visceral fat including the perirenal and epididymal fat. One previous report suggested that the perirenal and epididymal fat has deleterious effects and acts as a source to induce leptin, which is viewed as the insulin resistance adipokine. In contrast, removal of the perirenal and epididymal fat decreased the HFD-induced hepatic accumulation of triacylglyceride, and increased the hepatic expression of p-acetyl-CoA-carboxylase and the phosphorylation of Akt.19 Furthermore, SN improved hyperinsulinemia and insulin resistance, accompanied with downregulated lipogenesis.20 Hence the effect of SN on reducing body fat could have the potential to improve metabolic syndrome and its related complications.
In fact, SN was shown to reduce the serum triacylglyceride and cholesterol in HFD-fed rats. SN reduces the liver lipid, the hepatic expression of TNF-α and IL-6, and the oxidative burden. It has been suggested that SN could prevent the morbidity of nonalcoholic fatty liver-induced hepatitis because of its antihyperlipidemia, antioxidation, and anti-inflammatory properties.15 In the present study, we demonstrated that SWE ameliorated the hepatic lipid and hepatic expressions of enzymes which were altered by an HFD. By increasing the lipolysis and decreasing the lipogenesis, SN is expected to be the adjuvant to prevent non-alcoholic fatty liver.15
Viewed as a marker of lipid degradation, PPARα is actually one of the three isoforms of PPAR, a family of ligand-activated transcription factors. Among the isoforms α, β, and γ, PPARα is abundantly expressed in the liver, kidneys, and skeletal muscles.21 Although the activation of PPARα promoted mitochondrial fatty acid oxidation, it was also reported that PPARα mediated the hepatic uptake and esterification of fatty acids, affect the metabolism of lipoproteins.22 In the present study, the in vivo and in vitro expression of PPARα was dose-dependently increased by SN. Although it should mediate the oxidation synergistically with CPT-1, the other aspects regulated by PPARα should still not be excluded.
Noticeably, although both SWE and SNPE are potent regulators of lipid metabolism, SWE at 0.5% is not very useful while SNPE at 0.5 mg mL−1 works effectively. These results imply that the polyphenols composed of SN should be regarded as indispensable against obesity and body fat. Our previous analysis indicated that catechin, rutin, gallic acid, and isoquercetrin are rich in SN. Isoquercetrin inhibited the differentiation and adipogenesis of 3T3-L1 cells by activating the Wnt/β-catenin pathway.23 In the FFA/LPS-induced coculture model, isoquercetrin reversed the proinflammatory cytokines and oxidative stress. The antioxidative activity of isoquercetrin correlates with its lipid reducing potentials.24 Gallic acid significantly improved the glucose and insulin homeostasis in diet-induced obese mice. The administration of gallic acid protected diet-induced body weight gain without a change in food intake. The biochemical analyses revealed that gallic acid plays a beneficial role by activating the AMPK/Sirt1/PGC1α pathway, and changing the brown adipose tissue genes related to thermogenesis.25 In addition to improve the glucose tolerance and triacylglyceride concentration, gallic acid reduces the size of adipocytes in epididymal white adipose tissue.26 It was demonstrated that rutin suppressed the pro-inflammatory cytokines and oxidative stress-responsible genes. Treatment with rutin blocked the occurrence of obesity and fatty liver in HFD-fed mice, and was associated with reduced markers for macrophages in white adipose tissue.27 By decreasing the lipogenesis and oxidative stress, rutin inhibited oleic acid-induced lipid accumulation in hepatocytes.28 The tea cultivar rich methylated catechins lowered plasma TG, decreased adipose tissue weights, and reduced the hepatic expression of lipogenic genes, thus preventing diet-induced lipid metabolism disorder.29 Moreover, supplementation with catechin-rich grape seed extract suppresses the increase of body weight and body fat induced by a HFD.30
Conclusion
In conclusion, SN and its derived polyphenols are suggested to be feasible for use as anti-obese agents to reduce body weight and body fat, by inhibiting the survival and lipid accumulation of adipocytes, and regulating lipid metabolism.
Conflicts of interest
The authors declare no competing financial interests.
Abbreviations
AMPK | AMP-activated protein kinase |
CPT-1 | Carnitine palmitoyltransferase-1 |
DEX | Dexamethasone |
FaS | Fatty acid synthase |
GPAT | Glycerol-3-phosphate acyltransferase |
HFD | High fat diet |
HMG-CoA | 3-Hydroxy-3-methylglutaryl-coenzyme A |
HMG-CoR | HMG-CoA reductase |
IBMX | Isobutylmethylxanthine |
SN |
Solanum nigrum L |
SNPE | SN derived polyphenols |
SREBP | Sterol regulatory element binding protein |
SWE | SN water extract |
PPARα | Peroxisome proliferator-activated receptor α |
RSG | Rosiglitazone |
Acknowledgements
This study was supported by the Chung Shan Medical University and the Hungkaung University, Taichung, Taiwan, NSC-100-28110042-003.
References
- N. J. Temple and A. Conklin, Prevalence of overweight and obesity in Western countries: discrepancies in published estimates, Eur. J. Epidemiol., 2019, 34, 711–713 CrossRef PubMed.
- E. E. Calle, M. J. Thun, J. M. Petrelli, C. Rodriguez and C. W. Heath Jr., Body-mass index and mortality in a prospective cohort of US adults, N. Engl. J. Med., 1999, 341, 1097–1105 CrossRef CAS PubMed.
- M.-Y. Yang, C.-H. Peng, K.-C. Chan, Y.-S. Yang, C.-N. Huang and C.-J. Wang, The hypolipidemic effect of Hibiscus sabdariffa polyphenols via inhibiting lipogenesis and promoting hepatic lipid clearance, J. Agric. Food Chem., 2009, 58, 850–859 CrossRef PubMed.
- T. H. Kang, J. Y. Hur, H. B. Kim, J. H. Ryu and S. Y. Kim, Neuroprotective effects of the cyanidin-3-O-β-d-glucopyranoside isolated from mulberry fruit against cerebral ischemia, Neurosci. Lett., 2006, 391, 122–126 CrossRef CAS PubMed.
- P. Fischer-Posovszky, V. Kukulus, D. Tews, T. Unterkircher, K.-M. Debatin, S. Fulda and M. Wabitsch, Resveratrol regulates human adipocyte number and function in a Sirt1-dependent manner, Am. J. Clin. Nutr., 2010, 92, 5–15 CrossRef CAS PubMed.
- T. Bose, J. C. L. Alvarenga, M. E. Tejero, V. S. Voruganti, J. M. Proffitt, J. H. Freeland-Graves, S. A. Cole and A. G. Comuzzie, Association of monocyte chemoattractant protein-1 with adipocyte number, insulin resistance and liver function markers, J. Med. Primatol., 2009, 38, 418–424 CrossRef CAS PubMed.
- H. J. KIM, M. Miyazaki, W. C. Man and J. M. Ntambi, Sterol regulatory element-binding proteins (SREBPs) as regulators of lipid metabolism: polyunsaturated fatty acids oppose cholesterol-mediated induction of SREBP-1 maturation, Ann. N. Y. Acad. Sci., 2002, 967, 34–42 CrossRef CAS PubMed.
- B. H. Ali, N. A. Wabel and G. Blunden, Phytochemical, pharmacological and toxicological aspects of Hibiscus sabdariffa L.: a review, Phytother. Res., 2005, 19, 369–375 CrossRef CAS PubMed.
- K. G. Park, A. K. Min, E. H. Koh, H. S. Kim, M. O. Kim, H. S. Park, Y. D. Kim, T. S. Yoon, B. K. Jang and J. S. Hwang, Alpha-lipoic acid decreases hepatic lipogenesis through adenosine monophosphate-activated protein kinase (AMPK)-dependent and AMPK-independent pathways, Hepatology, 2008, 48, 1477–1486 CrossRef CAS PubMed.
- G. Zhou, R. Myers, Y. Li, Y. Chen, X. Shen, J. Fenyk-Melody, M. Wu, J. Ventre, T. Doebber and N. Fujii, Role of AMP-activated protein kinase in mechanism of metformin action, J. Clin. Invest., 2001, 108, 1167–1174 CrossRef CAS PubMed.
- Z. A. Zakaria, H. K. Gopalan, H. Zainal, N. H. M. Pojan, N. A. Morsid, A. Aris and M. R. Sulaiman, Antinociceptive, anti-inflammatory and antipyretic effects of Solanum nigrum chloroform extract in animal models, Yakugaku Zasshi, 2006, 126, 1171–1178 CrossRef CAS PubMed.
- C.-C. Hsieh, H.-L. Fang and W.-C. Lina, Inhibitory effect of Solanum nigrum on thioacetamide-induced liver fibrosis in mice, J. Ethnopharmacol., 2008, 119, 117–121 CrossRef PubMed.
- H.-M. Lin, H.-C. Tseng, C.-J. Wang, C.-C. Chyau, K.-K. Liao, P.-L. Peng and F.-P. Chou, Induction of autophagy and apoptosis by the extract of Solanum nigrum Linn in HepG2 cells, J. Agric. Food Chem., 2007, 55, 3620–3628 CrossRef CAS PubMed.
- S. Sohrabipour, F. Kharazmi, N. Soltani and M. Kamalinejad, Effect of the administration of Solanum nigrum fruit on blood glucose, lipid profiles, and sensitivity of the vascular mesenteric bed to phenylephrine in streptozotocin-induced diabetic rats, Med. Sci. Monit. Basic Res., 2013, 19, 133 CrossRef.
- J.-J. Chang, D.-J. Chung, Y.-J. Lee, B.-H. Wen, H.-Y. Jao and C.-J. Wang, Solanum nigrum polyphenol extracts inhibit hepatic inflammation, oxidative stress, and lipogenesis in high-fat-diet-treated
mice, J. Agric. Food Chem., 2017, 65, 9255–9265 CrossRef CAS PubMed.
- C. Koutsari and M. D. Jensen, Thematic review series: patient-oriented research. Free fatty acid metabolism in human obesity, J. Lipid Res., 2006, 47, 1643–1650 CrossRef CAS PubMed.
- Y. W. Kim, J. Y. Kim and S. K. Lee, Surgical removal of visceral fat decreases plasma free fatty acid and increases insulin sensitivity on liver and peripheral tissue in monosodium glutamate (MSG)-obese rats, J. Korean Med. Sci., 1999, 14, 539–545 CrossRef CAS PubMed.
- S. A. Porter, J. M. Massaro, U. Hoffmann, R. S. Vasan, C. J. O'Donnel and C. S. Fox, Abdominal subcutaneous adipose tissue: a protective fat depot?, Diabetes Care, 2009, 32, 1068–1075 CrossRef PubMed.
- S. Ben-Shlomo, F. H. Einstein, I. Zvibel, D. Atias, A. Shlomai, Z. Halpern, N. Barzilai and S. Fishman, Perinephric and epididymal fat affect hepatic metabolism in rats, Obesity, 2012, 20, 151–156 CrossRef CAS PubMed.
- C.-J. Tai, C.-Y. Choong, Y.-C. Shi, Y.-C. Lin, C.-W. Wang, B.-H. Lee and C.-J. Tai, Solanum nigrum protects against hepatic fibrosis via suppression of hyperglycemia in high-fat/ethanol diet-induced rats, Molecules, 2016, 21, 269 CrossRef PubMed.
- I. Gouni-Berthold and W. Krone, Peroxisome Proliferator-Activated Receptor alpha (PPAR alpha) and Atherosclerosis, Curr. Drug Targets: Cardiovasc. & Haematol. Disord., 2005, 5, 513–525 Search PubMed.
- J.-C. Fruchart and P. Duriez, Mode of action of fibrates in the regulation of triglyceride and HDL-cholesterol metabolism, Drugs Today, 2006, 42, 39–64 CAS.
- S. H. Lee, B. Kim, M. J. Oh, J. Yoon, H. Y. Kim, K. J. Lee, J. D. Lee and K. Y. Choi, Persicaria hydropiper (L.) spach and its flavonoid components, isoquercitrin and isorhamnetin, activate the Wnt/β-catenin pathway and inhibit adipocyte differentiation of 3T3-L1 cells, Phytother. Res., 2011, 25, 1629–1635 CrossRef CAS PubMed.
- W. Hassan, G. Rongyin, A. Daoud, L. Ding, L. Wang, J. Liu and J. Shang, Reduced oxidative stress contributes to the lipid lowering effects of isoquercitrin in free fatty acids induced hepatocytes, Oxid. Med. Cell. Longevity, 2014, 10, 313602 Search PubMed.
- K. V. Doan, C. M. Ko, A. W. Kinyua, D. J. Yang, Y.-H. Choi, I. Y. Oh, N. M. Nguyen, A. Ko, J. W. Choi and Y. Jeong, Gallic acid regulates body weight and glucose homeostasis through AMPK activation, Endocrinology, 2015, 156, 157–168 CrossRef PubMed.
- E.-J. Bak, J. Kim, S. Jang, G.-H. Woo, H.-G. Yoon, Y.-J. Yoo and J.-H. Cha, Gallic acid improves glucose tolerance and triglyceride concentration in diet-induced obesity mice, Scand. J. Clin. Lab. Invest., 2013, 73, 607–614 CrossRef CAS.
- M. Gao, Y. Ma and D. Liu, Rutin suppresses palmitic acids-triggered inflammation in macrophages and blocks high fat diet-induced obesity and fatty liver in mice, Pharm. Res., 2013, 30, 2940–2950 CrossRef CAS.
- C. H. Wu, M. C. Lin, H. C. Wang, M. Y. Yang, M. J. Jou and C. J. Wang, Rutin inhibits oleic acid induced lipid accumulation via reducing lipogenesis and oxidative stress in hepatocarcinoma cells, J. Food Sci., 2011, 76, T65–T72 CrossRef CAS PubMed.
- T. Suzuki, M. Kumazoe, Y. Kim, S. Yamashita, K. Nakahara, S. Tsukamoto, M. Sasaki, T. Hagihara, Y. Tsurudome and Y. Huang, Green tea extract containing a highly absorbent catechin prevents diet-induced lipid metabolism disorder, Sci. Rep., 2013, 3, 2749 CrossRef PubMed.
- K. Ohyama, C. Furuta, Y. Nogusa, K. Nomura, T. Miwa and K. Suzuki, Catechin-rich grape seed extract supplementation attenuates diet-induced obesity in C57BL/6J mice, Ann. Nutr. Metab., 2011, 58, 250–258 CrossRef CAS PubMed.
Footnote |
† These authors contributed equally. |
|
This journal is © The Royal Society of Chemistry 2020 |
Click here to see how this site uses Cookies. View our privacy policy here.