DOI:
10.1039/C9FO01980D
(Paper)
Food Funct., 2020,
11, 1146-1154
A new continuous system of enzymatic hydrolysis coupled with membrane separation for isolation of peptides with angiotensin I converting enzyme inhibitory capacity from defatted corn germ protein
Received
29th August 2019
, Accepted 26th November 2019
First published on 26th November 2019
Abstract
In this study, separation of peptides with Angiotensin I converting enzyme (ACE)-inhibitory capacity obtained from ultrasonically pretreated defatted corn germ protein (DCGP) by using a new continuous system of enzymatic hydrolysis coupled with membrane separation (EHC-MS) was investigated. Ultrasonic pretreatment was applied to enhance the enzymatic hydrolysis rate of DCGP, as proved in our previous study. The EHC-MS system was operated in two modes which included the batch system and continuous system with continuous water and substrate feeding and was compared with the EH-offline-MS system. The selection of the membrane was based on the hydrolysate fraction which had the highest activity for inhibition of ACE. The results showed that the 1–3 kDa fraction of DCGP hydrolysates had the lowest IC50 value (0.124 mg mL−1) for inhibition of ACE. The degree of conversion (%) of DCGP and output of peptides per unit of the enzyme were significantly (P < 0.05) increased by 55.3% and 55% in the EHC-MS batch process and 79% and 473% in the EHC-MS continuous operation compared to the EH-offline-MS system. The EHC-MS using continuous water and substrate feeding operation was noted to be the best in terms of a high degree of DCG protein conversion (75.68 ± 1.34) and the output of peptides per unit of the enzyme (78.65 ± 1.13). The results revealed that the EHC-MS method with constant water and substrate feeding could show a better application in peptide production in the food industry.
Introduction
One of the most crucial endogenous enzymes that can cause an increase in blood pressure in humans is Angiotensin I converting enzyme (ACE).1 Therefore, substances such as peptides that can inhibit ACE are categorized as ACE inhibitors through in-vitro determination. These categorized peptides are a crucial indicator of inhibition activity for ACE in hypertensive patients. An investigation showed a significant (p < 0.05) reduction in blood pressure after peptide ingestion as a result of ACE inhibition in Calpis sour milk.3 Peptides with ACE-inhibitory characteristics are ineffective when located among complex proteins. Their efficacy is only shown when isolated by enzymatic hydrolysis. In the literature, enzymatic hydrolysis techniques were used to produce ACE-inhibitory peptides from milk,4 gluten meal of corn5 and germ of wheat.6
Defatted corn germ (DCG) is a by-product of corn obtained after the extraction of oil in the industry. DCG is rich in minerals, protein and dietary fiber.7 As a result of its nutritional quality, DCG is presently used mostly as animal feed. An increase in DCG demand globally has warranted its exploitation for human consumption. DCG is considered as a rich source for peptide production with antihypertensive activity because of its substantial protein content. Enzymatic hydrolysis is used for peptide production, and it is beneficial in respect of production rate and high capacity.4,8 In addition, recent reports have explored the acceleration of hydrolysis of food by-products in the enzymatic reaction using ultrasound.2,9 The use of ultrasound technology has increased the efficiency of enzymes in converting proteins into peptides and polypeptides with useful functional characteristics.10 This is because the treatment of proteins with sonication makes a change in the protein structure, which enables the enzymes to access the peptide bond quickly and smoothly, leading to rapid hydrolysis.11 The difference in the protein structure, stimulated by ultrasound pretreatment, indicates the generation of cavitation shear stress and vigorous agitation, leading to the breaking of covalent bonds between protein molecules which results in protein unfolding.12–14 Membrane separation (MS) is widely applied in isolation of peptides using their molecular weight from the hydrolysates, and it has an affinity for high selectivity and max fluid permeability.15–19 Most often, the enzymatic hydrolysis and membrane separation methods are individually operated, which results in lower desired peptide yield and a minimal rate of protein conversion. Therefore, to enhance the peptide yield and the capacity of operation, the use of the enzymatic hydrolysis linked with membrane separation technique could be explored. The synergy of MS and enzymatic hydrolysis (EH) in one system could mostly use the avails of biotechnology and membrane separation technology to increase the production rate and enhance the separation of bioactive peptides. The EHC-MS technique could upgrade the substrate conversion rate and enzyme capacity and increase the yield of the product. Again the method could lead to the recovery of the enzyme and product isolation better than offline MS and traditional-EH methods.20 In previous studies, the use of the EHC-MS system was confronted with some difficulties. For instance, Qu et al.21 indicated that the use of the EHC-MS system with the constant addition of the substrate led to a decrease in the degree of protein conversion due to the reduction in the mobility of reactants within the reactor as a result of the continuous water loss. In this paper, the EHC-MS system was designed to ensure that the movement of reactants is maintained within the reactor and they continue to react with high efficiency for as long as possible. This design is based on the addition of water and a substrate to the reactor in different proportions by using an advance feeding pump which could be adjusted to add water and a substrate to the reactor with a constant flow rate. The objective of this work was to develop an innovative continuous EHC-MS system for ACE inhibitory peptide production from DCGP pretreated with ultrasound (multi-frequency counter-current S type device).
Materials and methods
Materials
Defatted corn germ (DCG) (protein content, 18.93%) was obtained from Shandong Sanxing Corn Industry and Technology Co., Ltd (Shandong, China). Alcalase enzyme with 190
000 U g−1 activity at 50 °C and 9.0 pH was obtained from Novozymes Co., Ltd (Shanghai, China). ACE was isolated from the pig lung using a protocol reported by Qu et al.21 and one unit of enzyme activity was expressed as 0.1 U mL−1.
Ultrasonic pretreatment
The ultrasound pretreatment of 50 g L−1 defatted corn germ (DCG) solution was conducted under the following conditions: DCG solution volume of 2.5 L, 113.6 W L−1 ultrasonic power density, 30 °C and ultrasound pretreatment time of 15 min using a multi-frequency counter-current S type device (Jiangsu University) equipped with five frequency generators, which were 20, 28, 35, 40 and 52 kHz, with maximum output acoustic powers of 80, 80, 48, 48 and 28 W respectively. The DCG solution was adjusted to 9.0 pH with 4 mol L−1 NaOH before the ultrasonic pretreatments.
Production of the DCG protein hydrolysate
Fig. 1 shows the schematic diagram of the enzymatic hydrolysis of DCGP. Prior to the hydrolysis reaction, DCG was pretreated with a multi-frequency counter-current S type ultrasound device under the optimum conditions which included a treatment time of 15 min, a power density of 113 W L−1 and a substrate concentration of 5%, identified previously (data not shown). After ultrasonic pretreatment, enzymatic hydrolysis was conducted under the following optimum conditions of Alcalase enzyme concentration (2000 U mL−1), pH (9), temperature (50 °C), and hydrolysis time (90 min). The obtained hydrolysate from corn germ protein was cooled and centrifuged for 10 min at 4000g and 25 °C. The DCG protein hydrolysate was then used for the subsequent experiments.
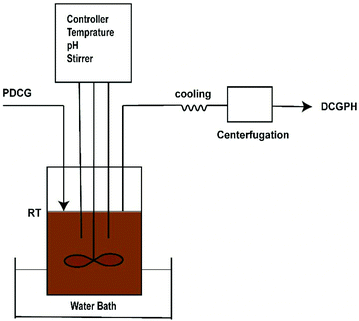 |
| Fig. 1 Schematic diagram of enzymatic hydrolysis of DCGP: PDCG, ultrasonically pretreated DCGP; RT, reaction tank; DCGPH, defatted corn germ protein hydrolysate. | |
Offline MS process
The DCG protein hydrolysate was fractionated by using the offline membrane separation technique. The offline membrane separation technique is composed of an ultra-filtration (UFU) device (P., M. Corporate, B., MA, USA) linked to molecular weight cut-off (MWCO) membranes (0.5 m2 working area) of 100, 5, 3, and 1 kDa. The fractionation process was operated as follows: firstly, the supernatant of the DCG protein hydrolysate was passed through the membranes in the order of 100 kDa, 5 kDa, 3 kDa and 1 kDa to remove starch and subsequently produce peptide sizes of >5, 3–5, 1–3 and <1 kDa, respectively. The inhibitory activities of each fraction of the DCGP hydrolysate to ACE were subsequently examined.
ACE inhibitory activity
The ACE inhibitory activities of the DCGP hydrolysate (peptides) were determined using the colorimetric method.22 The samples were 20-times diluted with distilled water. Then a mixture of the diluted sample (100 μL) and ACE (50 μL) was added to 1.0 mM N-[3-(2-furylacryloyl)]-L-phenylalanine-glycyl-glycine (FAPGG) (50 μL) for 30 min in a 96-well plate and kept in an incubator (SPX-250B, C. G. Co., Ltd, Jiangsu, China) at 37 °C. The reduction in absorbance read at 340 nm using a UV–Vis spectrophotometer (TU1810; Purkinje General Instrument Co., Ltd, Beijing, China) was noted as a1 and a2 for a sample before and after the reaction respectively. The control was prepared by following the same method, but instead of the sample, 100 μL of 0.08 M 4-(2-hydroxyethyl)-1-piperazine ethane sulfonic acid (HEPES) solution was added. The reduction in absorbance of the control was noted as b1 and b2. ACE inhibitory activity (expressed in %) was computed with eqn (1): |  | (1) |
where a1 is the absorbance at t = 0 min of sample reaction, a2 is the absorbance at 30 min of reaction, and b1 and b2 are the absorbances of the control at zero and 30 min of reaction, respectively.
The IC50 value expressed in g L−1 refers to the concentration of the peptide that can inhibit 50% of ACE and was calculated according to a previous report.21
The batch operation process of the EHC-MS system
Peptides with ACE inhibitory function were produced from the DCGP hydrolysate by using the batch EHC-MS system. The following procedure was used to operate the EHC-MS system. First, the DCGP hydrolysate with Mw > 5 kDa was produced and isolated using the described method (offline MS process). Afterwards, Alcalase enzyme was added to the DCGP hydrolysate to react with a known concentration in a reactor tank (RT) at 50 °C and 9.0 pH. The recycle pump (BT00-300, C. C. S. A. Factory, Jiangsu, China) was operated at a flow rate of 9 mL min−1. The peptides with desired Mw were collected after permeating through the selected membrane. The un-hydrolyzed protein, enzyme and other ingredients with molecular weights larger than that of the selected membrane were pumped into the RT again. The impact of the substrate concentration, processing time, enzyme concentration and flow rate on the process yield was optimized using the single-factor test to achieve the optimal operation conditions of the batch EHC-MS system. The DCGP conversion degree was evaluated using the following equation: |  | (2) |
where Pt is the peptide concentration in the permeate at a given time and was evaluated by using the method reported by Qu et al.,23t is the time, s is the flow rate, P is the initial protein concentration, and v is the volume of the reaction mixture.
Orthogonal array experimental design
The L9 orthogonal array of the Taguchi method24 was used in this study to optimize the operating conditions of the EHC-MS batch system involving various variables and levels. The L9 orthogonal array experiment was designed on the basis of the parameters and levels listed in Table 1. Four parameters were considered: time of operation, flow rate, enzyme concentration and substrate concentration. Three levels were selected for each parameter and conducted through 9 runs. The conversion degree (%) of DCGP was used as the response item in the Taguchi experimental design.
Table 1 The L9(34) orthogonal experimental design for the EHC-MS batch process
Level |
Time |
Flow rate (mL min−1) |
Enzyme concentration (U g−1) |
Substrate concentration (g L−1) |
1 |
30 |
9 |
1000 |
3 |
2 |
60 |
7 |
500 |
4 |
3 |
45 |
11 |
1500 |
5 |
The S/N ratio was calculated based on eqn (3):25
| 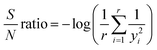 | (3) |
where
r is the number of the run and
yi is the experimental response (the conversion degree (%)).
EHC-MS reactor continuous operation process
The continuous EHC-MS system (as depicted in Fig. 2) was operated for 5 h, with constant water and DCGP hydrolysate feeding, under operation conditions of DCGP concentration (4 g L−1), enzyme concentration (1000 U mL−1 of reaction), at 50 °C, 9.0 pH and flow rate (9 mL min−1). Water and the DCGP hydrolysate were added continuously to the reactor tank at a different ratio of 25
:
75% of water
:
DCGP hydrolysate beginning from 75
:
25% water to DCGP hydrolysate ratio using a feeding pump (FP) (JIHPUMP 253Yx, Chongqing Jieheng Peristaltic Pumps Co., Ltd) at a flow rate of 9 mL min−1. A constant volume (500 mL) was maintained in the reactor tank, by operating the feeding pump (FP) and recycle pump (RP) at the same flow rate (9 mL min−1).
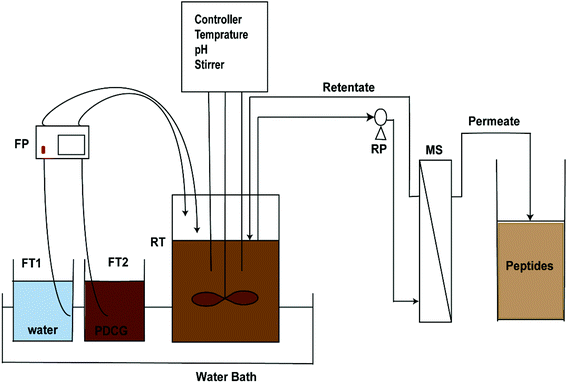 |
| Fig. 2 Schematic diagram of the EHC-MS continuous process: DCGP, defatted corn germ protein; FT1,2, feeding tanks; FP, feeding pump; RT, reaction tank; RP, recycle pump; MS, membrane separation. | |
Statistical analysis
All experiments were conducted in triplicate. Under the significance level of P < 0.05, the ANOVA test was performed using SPSS version 18.0 (IBM Corporation, NY, USA).
Results and discussion
Selection of the ultrafiltration membrane
The ACE inhibitory activity of each fraction of DCGP hydrolysates was investigated and expressed as IC50. The ACE IC50 values of DCGP hydrolysate fractions are depicted in Fig. 3. The result indicated that the DCGP hydrolysate fractions with molecular weights of <1 kDa, 1–3 kDa, 3–5 kDa and >5 kDa have ACE inhibitory properties, and their IC50 values were 0.233, 0.124, 0.384 and 1.14 mg mL−1, respectively. The 1–3 kDa fractions had the lowest ACE inhibitory IC50 value when compared with the other DCGP hydrolysate fractions, which was smaller than what was reported by Jian et al.9 Also, our finding of the ACE inhibitory IC50 value for the 1–3 kDa fraction was lower than that achieved by Qu et al.23 who observed peptide activity for ACE-inhibition by hydrolysates of defatted wheat germ protein with an offline membrane separation value of 0.523 g L−1. Thus, the 3 kDa ultrafiltration membrane was chosen for producing a peptide with the highest ACE inhibitory activity from DCGP hydrolysates in the EHC-MS batch process.
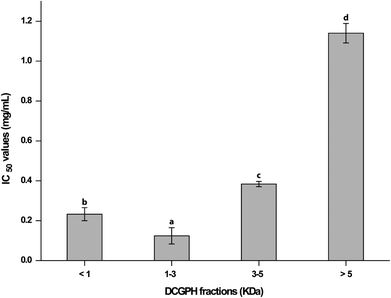 |
| Fig. 3 The ACE IC50 values of peptide fractions separated from DCGP hydrolysates. | |
EHC-MS batch process
Impact of processing time on conversion degree.
The effect of time on the conversion degree % of DCGP in the EHC-MS batch process system was evaluated. From the result presented in Fig. 4, one can deduce that the conversion degree % of DCGP increased gradually with an increase in reaction time and reached equilibrium after 60 min of operation. A similar result was also noted by Qu et al.21 The increase in the conversion degree % of DCGP could be attributed to the separation of peptides with less than 3 kDa molecular weight through the continuous online MS system, which can facilitate the work of Alcalase enzyme in the hydrolysis reaction. Another reason for the increase of conversion degree % in online MS was suggested by Sousa et al. and Tardioli et al.,26,27 who suggested that small peptides produced during traditional enzymatic hydrolysis of protein could cause inhibition of protease enzyme. From the result, the 60 min operation time was chosen for the subsequent experiments.
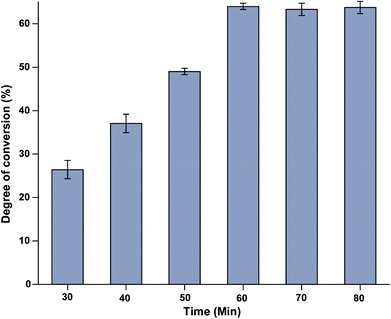 |
| Fig. 4 The effect of time of processing on the degree of conversion % of DCGP in the EHC-MS batch process system. | |
Impact of the concentration of the substrate on the conversion degree %.
Fig. 5 illustrates the effect of the substrate concentration on the conversion degree of DCGP in the EHC-MS batch process system. Five DCGP levels of 1, 2, 3, 4 and 5 g L−1 were used. The results showed that the conversion degree % of DCGP gradually increased with an increase in substrate concentration from 9.02% ± 0.1 and 39.02% ± 0.1 at a DCGP level of 1 g L−1 to 14.48% ± 0.2 and 65.11% ± 0.12 at a DCGP level of 4 g L−1 after 15 and 60 min of reaction respectively. Also, the result demonstrated that the conversion degree % of DCGP began to decrease when the substrate concentration exceeded 4 g L−1 after 60 min of reaction. The 4 g L−1 DCGP concentration was adjudged the best concentration based on the highest conversion degree % of DCG protein, among the five DCGP levels investigated.
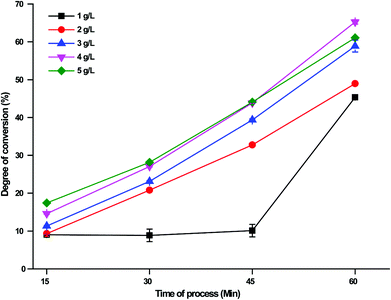 |
| Fig. 5 The effect of DCGP concentration on the degree of conversion % in the EHC-MS batch process system. | |
Impact of the concentration of enzyme on conversion degree %.
The effect of enzyme concentration on the conversion degree % of DCGP in the EHC-MS batch process system using various levels of the enzyme concentration (500, 1000 and 1500 U mL−1) is depicted in Fig. 6. The results showed that the conversion degree % of DCGP in the EHC-MS batch process system increased with increasing concentration of enzyme and peaked at a maximal conversion degree % value of 63.37% ± 0.14 at 1000 U mL−1 enzyme concentration. The result also indicated that the conversion degree % started to decrease from the peak at 1000 U g−1 enzyme concentration to 50.28% ± 0.25 at 1500 U mL−1 enzyme concentration. A similar observation was reported by Qu et al.,21 who observed that under given reaction conditions, increasing the enzyme concentration more than the optimum level had no increasing effect on the conversion rate of protein into desired peptides. Therefore, the enzyme concentration of 1000 U mL−1 was chosen as the best concentration.
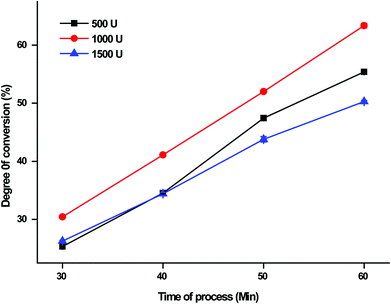 |
| Fig. 6 The effect of concentration of enzyme on the degree of conversion % from DCGP in the EHC-MS batch process system. | |
Impact of flow rate on the conversion degree %.
The effect of flow rates on the conversion degree % of DCGP in the EHC-MS batch process system using different flow rates of 7, 9, 11 and 13 mL min−1 was also investigated. From the results presented in Fig. 7, it became clear that the conversion degree % increased with increasing the flow rate until a specific flow rate (9 mL min−1). Beyond 9 mL min−1 flow rate, a decrease in conversion degree was also noted. For instance, protein conversion decreased from 51.56% ± 0.25166 at 9 mL min−1 flow rate to 49.44% ± 0.07071 at 13 mL min−1 flow rate. This might be due to water loss that occurred with increasing flow rate, which led to a reduction in mobility of the substrate and enzyme molecules in the reaction mixture. This meant that the conversion degree % decreased with increasing flow rate. Our finding was in agreement with Qu et al.,21 who reported a gradual decrease of conversion rate in Porphyra yezoensis protein with increasing speed of the pump.
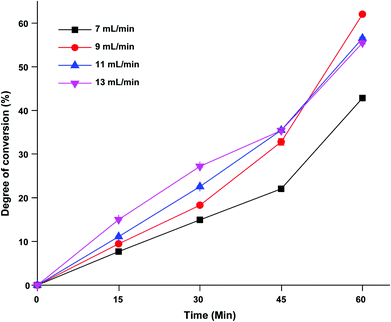 |
| Fig. 7 The effect of flow rates on the degree of conversion % from DCGP in the EHC-MS batch process system. | |
Optimization of the EHC-MS batch process operation conditions by the L9 (34) orthogonal design
The orthogonal experimental design is one of the most sufficient and fast procedures for studies involving many parameters in order to examine which variables had the most affecting characteristics of the desired product.24 In this paper, the L9 (34) orthogonal design table was used for the optimization of the EHC-MS batch process, and the corresponding conversion degree (%) of DCGP was used as a response variable under the nine operating conditions shown in Tables 2 and 3. The signal-to-noise (S/N) ratio, which indicates the effect of each parameter on the conversion degree (%) of DCGP, was estimated using the criterion of the-larger-the-better for each parameter since a higher conversion degree (%) of DCGP from the EHC-MS batch process was required. The S/N ratio and the mean response were determined from the orthogonal experimental data shown in Table 2. The results are presented in Tables 3 and 4. The result of the mean response and the S/N ratio in Tables 3 and 4 showed that increasing process time increases the conversion degree (%) of DCGP. Considering the enzyme concentration, an initial increase in the conversion degree (%) of DCGP occurred with increasing enzyme concentration i.e. from 500 U g−1 to 1000 U g−1 which was closely followed by a decrease in the conversion degree (%) of DCGP in a higher enzyme concentration (1500 U g−1). In the cases of flow rate and substrate concentration, a noticeable increase in the conversion degree (%) of DCGP was observed when the flow rate and substrate concentration increased from 7 to 11 mL min−1 and 3 to 4 g L−1 respectively. In addition, the difference between the levels (Delta) in Tables 3 and 4 revealed that the process time was the most significant factor affecting the conversion degree (%) of DCGP. This was closely followed by flow rate and substrate concentration in terms of impact on the conversion degree (%) of DCGP whilst enzyme concentration had the least effect on the conversion degree (%) of DCGP.
Table 2 Test results of the L9 (34) orthogonal experiment for the EHC-MS batch process
Run no. |
Time |
Flow rate |
Enzyme concentration |
Substrate concentration |
Conversion degree (%) |
1 |
1 |
1 |
1 |
1 |
26.42 ± 0.31 |
2 |
1 |
2 |
2 |
2 |
25.33 ± 0.28 |
3 |
1 |
3 |
3 |
3 |
34.53 ± 0.13 |
4 |
2 |
1 |
2 |
3 |
55.48 ± 1.13 |
5 |
2 |
2 |
3 |
1 |
42.47 ± 0.20 |
6 |
2 |
3 |
1 |
2 |
61.66 ± 0.52 |
7 |
3 |
1 |
3 |
2 |
43.83 ± 0.19 |
8 |
3 |
2 |
1 |
3 |
44.09 ± 0.08 |
9 |
3 |
3 |
2 |
1 |
39.36 ± 0.34 |
Table 3 Response table for signal to noise ratios in the EHC-MS batch process
Level |
Time |
Flow rate |
Enzyme concentration |
Substrate concentration |
k1 |
29.09 |
32.05 |
32.37 |
30.97 |
k2 |
34.41 |
31.17 |
31.62 |
32.24 |
k3 |
32.54 |
32.82 |
32.05 |
32.84 |
Delta |
5.32 |
1.65 |
0.76 |
1.88 |
Rank |
1 |
3 |
4 |
2 |
Table 4 Response table for a mean of protein conversion degree (%) in the EHC-MS batch process
Level |
Time |
Flow rate |
Enzyme concentration |
Substrate concentration |
k1 |
28.76 |
41.91 |
44.06 |
36.09 |
k2 |
53.20 |
37.30 |
40.06 |
43.61 |
k3 |
42.43 |
45.18 |
40.28 |
44.70 |
Delta |
24.44 |
7.89 |
4.00 |
8.61 |
Rank |
1 |
3 |
4 |
2 |
EHC-MS reactor continuous operation process with water and substrate feeding.
The work by Qu et al.21 reported about 13% and 260% increase in peptide output when the EHC-MS system was run with a constant addition of water and continuous addition of a substrate respectively. The novelty of this research mainly depends on the constant addition of the water and a substrate in different proportions to the EHC-MS system by using a FP, to avoid the loss of water (a problem associated with the EHC-MS system) and increase of the substrate concentration in the reactor. In addition this approach prevented the clogging of the membrane, a common challenge associated with the EHC-MS system. The water and substrate were added to the reactor at a different portion (25
:
75% to 75
:
25%) by using an advance FP. This pump could be reset to add the solutions as a gradient with a constant flow rate which is similar to that of HPLC. Continuous production of the peptide with useful characteristics from DCGP hydrolysates was performed through the continual system of EHC-MS and is presented in Fig. 2. The degree to which the DCGP was converted to peptides and the DCGP residue in the reactor are demonstrated in Fig. 8. The purpose of the assessment of the degree of DCGP conversion during the process was to measure the efficiency of the EHC-MS system with continuous water and substrate feeding. Also, the DCGP residue within the reactor was monitored to maintain the balance between the enzyme and substrate during the operation. The results revealed that the degree of DCGP conversion to peptides increased gradually during the reaction period. This proved the effectiveness of the EHC-MS system with continuous water and substrate feeding in the production of bioactive-peptides. This outcome presented a solution to the problem reported in the literature;21 that is, a continuous addition of the substrate led to a gradual decrease in the degree of protein conversion when the EHC-MS system with constant feeding of the substrate was applied. Also, the results indicated that the ratio of residual protein in the reactor started to increase at the beginning of the process and then reached a state of stability after 30 min of reaction, which then began to decrease slightly toward the end of the process. This was as a result of feeding at the beginning of the process, consisting of a substrate
:
water ratio of 75
:
25, resulting in an increase in the ratio of residual protein within the reactor, whilst a substrate
:
water reaction ratio of 25
:
75 was observed at the end of the reaction.
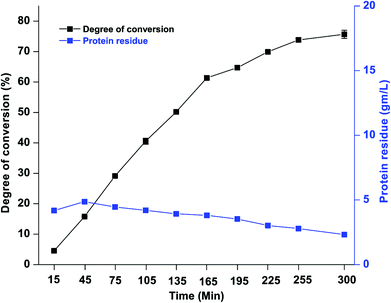 |
| Fig. 8 The degree of conversion % of DCGP to peptides and the DCGP hydrolysate residue in the reactor, in the EHC-MS continuous process. | |
Comparison between the EHC-MS process (batch and continuous) and EH-offline-MS
Table 5 presents the performance data of EH-offline-MS and EHC-MS systems (batch and continuous). The degree of conversion % and the peptide output (per unit of enzyme) of EH-offline-MS were 42.24% ± 0.041 and 13.72 ± 0.0131, respectively. In comparison with EH-offline-MS, the EHC-MS batch process increased significantly (P < 0.05) the conversion degree % of DCGP by 55.3% and the output of peptides per unit of the enzyme by 55%. The continuous operation of EHC-MS with constant water and substrate feeding gave the best results for the degree of protein conversion and the peptide output per unit of enzyme by 75.68% ± 1.34 and 78.65 ± 1.13, respectively. This also led to an increase in the degree of protein conversion by 79.2% and peptide output per unit of enzyme by 473%. Our finding was significantly (P < 0.05) higher than what was observed by Qu et al.,23 who observed that the batch operation of the EHC-MS reactor significantly (p < 0.05) caused an increase in the conversion of Porphyra yezoensis protein by 43.6% when compared with the traditional process and continuous operation of the EHC-MS system. Thus, the EHC-MS with constant water and substrate feeding is useful as it can produce peptides with high activity for ACE inhibition and enhance the enzyme utilization rate through recycling whilst eliminating the inhibition effect caused by the product and protecting target products from excessive degradation.
Table 5 Comparison of the degree of conversion % in the EH-offline-MS, EHC-MS batch process and EHC-MS continuous process with constant water and substrate feeding
Methods |
The conversion degree % |
Output of peptides per unit of enzyme (gm U−1) |
Lower case letters in the same columns represent significant difference at P < 0.05. |
EH-offline-MS |
42.24 ± 0.04c |
13.72 ± 0.01c |
EHC-MS batch process |
65.48 ± 1.12b (55.3%) |
21.26 ± 0.21b (55%) |
EHC-MS continuous process with constant water and substrate feeding |
75.68 ± 1.34a (79.2%) |
78.65 ± 1.13a (473%) |
Conclusions
In conclusion, this research demonstrated that the membrane separation technique (EHC-MS) coupled with enzymatic hydrolysis was noted to be a promising method for peptide production with high ACE-inhibitory activity from DCGP pretreated ultrasonically. The results indicated that the 1–3 kDa fractions had the lowest IC50 value (0.124 mg mL−1) for ACE inhibition when compared with the other DCGP hydrolysate fractions. The optimized operating conditions of the EHC-MS batch system were used to perform the continuous EHC-MS with constant substrate and water feeding, which gave the best results for the degree of protein conversion and the peptide output when compared with the EH offline MS and the batch process of EHC-MS systems. On the basis of the above-mentioned results, the EHC-MS method with constant water and substrate feeding could be considered to have a potential application in bioactive peptide production in the food industry.
Conflicts of interest
The authors declare no competing financial interest.
Acknowledgements
This work was financially supported by the Provincial Postdoctoral Funds of Jiangsu Province (1701026A) and the National Key Research and Development Programme (2016YFD0400303).
References
- A. Musa, M. A. A. Gasmalla, H. Ma, F. Sarpong, A. Wali, F. N. Awad and Y. Duan, Effect of a multi-frequency counter-current S-type ultrasound pretreatment on the defatted corn germ protein: enzymatic hydrolysis, ACE inhibitory activity and structural characterization, Food Funct., 2019, 10, 6020–6029 RSC.
- A. Musa, H. Ma, M. A. A. Gasmalla, F. Sarpong, F. N. Awad and Y. Duan, Effect of multi-frequency counter-current S type ultrasound pretreatment on the enzymatic hydrolysis of defatted corn germ protein: Kinetics and thermodynamics, Process Biochem., 2019, 87, 112–118 CrossRef CAS.
- Y. Hata, M. Yamamoto, M. Ohni, K. Nakajima, Y. Nakamura and T. Takano, A placebo-controlled study of the effect of sour milk on blood pressure in hypertensive subjects, Am. J. Clin. Nutr., 1996, 64, 767–771 CrossRef CAS PubMed.
- N. Yamamoto and T. Takano, Antihypertensive peptides derived from milk proteins, Food/Nahrung, 1999, 43, 159–164 CrossRef CAS.
- Y. Yang, G. Tao, P. Liu and J. Liu, Peptide with angiotensin I-converting enzyme inhibitory activity from hydrolyzed corn gluten meal, J. Agric. Food Chem., 2007, 55, 7891–7895 CrossRef CAS.
- T. Matsui, C.-H. Li, T. Tanaka, Y. OSAJIMA and K. MATSUMOTO, Depressor effect of wheat germ hydrolysate and its novel angiotensin I-converting enzyme inhibitory peptide, Ile-Val-Tyr, and the metabplism in rat and human plasma, Biol. Pharm. Bull., 2000, 23, 427–431 CrossRef CAS.
- C. J. Huang and J. F. Zayas, Aroma quality of corn germ protein flours determined by sensory and gas chromatographic profiles, J. Food Qual., 1991, 14, 377–390 CrossRef CAS.
- A. Jang, C. Jo, K.-S. Kang and M. Lee, Antimicrobial and human cancer cell cytotoxic effect of synthetic angiotensin-converting enzyme (ACE) inhibitory peptides, Food Chem., 2008, 107, 327–336 CrossRef CAS.
- S. Jian, T. Wenyi and C. Wuyong, Ultrasound-accelerated enzymatic hydrolysis of solid leather waste, J. Cleaner Prod., 2008, 16, 591–597 CrossRef.
- A. Clemente, Enzymatic protein hydrolysates in human nutrition, Trends Food Sci. Technol., 2000, 11, 254–262 CrossRef CAS.
- X. Yang, Y. Li, S. Li, A. O. Oladejo, Y. Wang, S. Huang, C. Zhou, Y. Wang, L. Mao, Y. Zhang, H. Ma and X. Ye, Effects of multi-frequency ultrasound pretreatment under low power density on the enzymolysis and the structure characterization of defatted wheat germ protein, Ultrason. Sonochem., 2017, 38, 410–420 CrossRef CAS.
- D. Güzey, İ. Gülseren, B. Bruce and J. Weiss, Interfacial properties and structural conformation of thermosonicated bovine serum albumin, Food Hydrocolloids, 2006, 20, 669–677 CrossRef.
- A. R. Jambrak, T. J. Mason, V. Lelas, Z. Herceg and I. L. Herceg, Effect of ultrasound treatment on solubility and foaming properties of whey protein suspensions, J. Food Eng., 2008, 86, 281–287 CrossRef CAS.
- D. Knorr, M. Zenker, V. Heinz and D.-U. Lee, Applications and potential of ultrasonics in food processing, Trends Food Sci. Technol., 2004, 15, 261–266 CrossRef CAS.
- E. Darnon, E. Morin, M. Belleville and G. Rios, Ultrafiltration within downstream processing: some process design considerations, Chem. Eng. Process., 2003, 42, 299–309 CrossRef CAS.
- T. Gotoh, H. Iguchi and K.-I. Kikuchi, Separation of glutathione and its related amino acids by nanofiltration, Biochem. Eng. J., 2004, 19, 165–170 CrossRef CAS.
- C. Martin-Orue, S. Bouhallab and A. Garem, Nanofiltration of amino acid and peptide solutions: mechanisms of separation, J. Membr. Sci., 1998, 142, 225–233 CrossRef CAS.
- Y. Pouliot, M. Wijers, S. Gauthier and L. Nadeau, Fractionation of whey protein hydrolysates using charged UF/NF membranes, J. Membr. Sci., 1999, 158, 105–114 CrossRef CAS.
- A. Saxena, B. P. Tripathi, M. Kumar and V. K. Shahi, Membrane-based techniques for the separation and purification of proteins: an overview, Adv. Colloid Interface Sci., 2009, 145, 1–22 CrossRef CAS.
- G. Rios, M. Belleville, D. Paolucci and J. Sanchez, Progress in enzymatic membrane reactors–a review, J. Membr. Sci., 2004, 242, 189–196 CrossRef CAS.
- W. Qu, H. Ma, W. Li, Z. Pan, J. Owusu and C. Venkitasamy, Performance of coupled enzymatic hydrolysis and membrane separation bioreactor for antihypertensive peptides production from Porphyra yezoensis protein, Process Biochem., 2015, 50, 245–252 CrossRef CAS.
- L. Actis-Goretta, J. I. Ottaviani, C. L. Keen and C. G. Fraga, Inhibition of angiotensin converting enzyme (ACE) activity by flavan-3-ols and procyanidins, FEBS Lett., 2003, 555, 597–600 CrossRef CAS.
- W. Qu, H. Ma, W. Zhao and Z. Pan, ACE-inhibitory peptides production from defatted wheat germ protein by continuous coupling of enzymatic hydrolysis and membrane separation: modeling and experimental studies, Chem. Eng. J., 2013, 226, 139–145 CrossRef CAS.
- M. Dargahi, H. Kazemian, M. Soltanieh, M. Hosseinpour and S. Rohani, High temperature synthesis of SAPO-34: Applying an L9 Taguchi orthogonal design to investigate the effects of experimental parameters, Powder Technol., 2012, 217, 223–230 CrossRef CAS.
- Y. H. Chan, K. V. Dang, S. Yusup, M. T. Lim, A. M. Zain and Y. Uemura, Studies on catalytic pyrolysis of empty fruit bunch (EFB) using Taguchi's L9 Orthogonal Array, J. Energy Inst., 2014, 87, 227–234 CrossRef CAS.
- R. Sousa Jr., G. Lopes, P. Tardioli, R. Giordano, P. Almeida and R. Giordano, Kinetic model for whey protein hydrolysis by alcalase multipoint-immobilized on agarose gel particles, Braz. J. Chem. Eng., 2004, 21, 147–153 CrossRef.
- P. W. Tardioli, R. Sousa Jr., R. C. Giordano and R. L. Giordano, Kinetic model of the hydrolysis of polypeptides catalyzed by Alcalase® immobilized on 10% glyoxyl-agarose, Enzyme Microb. Technol., 2005, 36, 555–564 CrossRef CAS.
|
This journal is © The Royal Society of Chemistry 2020 |