DOI:
10.1039/C9FO01205B
(Review Article)
Food Funct., 2020,
11, 93-110
Health benefits of 4,4-dimethyl phytosterols: an exploration beyond 4-desmethyl phytosterols
Received
6th June 2019
, Accepted 9th November 2019
First published on 11th November 2019
Abstract
4,4-Dimethyl phytosterols possess two methyl groups at the carbon-4 atom of the aliphatic A-ring. The methyl groups are crucial for the molecular recognition of endogenous and exogenous bioactive compounds. Phytosterols have received worldwide attention owing to their recognized health benefits. However, 4,4-dimethyl phytosterols are less appreciated. Recent research studies revealed that 4,4-dimethyl phytosterols exert numerous beneficial effects on disease prevention, and are particularly involved in the endogenous cannabinoid system (ECS). The purpose of this review is to summarize and highlight the currently available information regarding the structures and sources of 4,4-dimethyl phytosterols, and to provide detailed preclinical studies performed to evaluate their potential for treating various diseases. Future research on 4,4-dimethyl phytosterols is warranted to confirm their relationship with the ECS, and to elucidate the mechanism directly toward clinical trials.
1. Introduction
Plant sterols share a fused polycyclic structure and differ in the number or position of unsaturated bonds, and the length of the substitutents and side chains. Based on the location of methyl groups at the carbon-4 position, phytosterols are classified into 4,4-dimethyl phytosterols, 4-monomethyl phytosterols, and 4-desmethyl phytosterols.1 More than 250 unique phytosterols have been identified in recent years.2 The 4,4-dimethyl phytosterols are quantitatively minor in nature, but are typically found in plants and vegetable oils. Some belonging to the triterpene family also contain two methyl substituents, thus falling into the group of 4,4-dimethyl phytosterols that includes lupeol, α-amyrin, β-amyrin, cycloartanol, taraxerol, etc.3,4 The 4-desmethyl phytosterols have received worldwide attention due to their efficient cholesterol-lowering activity. The most common 4-desmethyl phytosterols are β-sitosterol, stigmasterol, and campesterol. Many reviews address the progress of the structure and variation of 4-desmethyl phytosterols,5 as well as the metabolism and function in vivo.6–8 However, a comprehensive review about the benefits of 4,4-dimethyl phytosterols has not appeared.
The 4,4-dimethyl phytosterols are naturally occurring bioactive compounds, and resemble 4-desmethyl phytosterols in many functions.9,10 According to the World Health Organization (WHO), noncommunicable diseases (NCDs) play a key role in the global mortality. Previous studies demonstrated that the 4,4-dimethyl phytosterols can mitigate NCDs and associated disorders. Examples are obesity suppressed by α,β-amyrin,9,11 diabetes prevented by taraxerol,12,13 as well as hyperlipidemia ameliorated by lupeol, 24-methylene cycloartanol, and cycloartenol in animals or humans.14 In addition to regulating lipid metabolism, 4,4-dimethyl phytosterols also exhibited anti-inflammatory, anticancer, and antinociceptive activities.
Though diverse factors account for the death rate worldwide, there seems to be a relationship between 4,4-dimethyl phytosterol intake and the death rate from NCDs (Fig. 1). As shown in Fig. 1, inside the black dotted box, the regions with a higher intake of 4,4-dimethyl phytosterols seem to have lower mortality from NCDs, such as North America, Mediterranean, East Asia, and Australia. In-depth studies ascertained the linkages between the 4,4-dimethyl phytosterols and the endogenous cannabinoid system (ECS), whose dysregulation could cause various NCDs.18 This review highlights the structures and sources of naturally occurring 4,4-dimethyl phytosterols, and provides a detailed account of the preclinical studies performed to evaluate the effectiveness of 4,4-dimethyl phytosterols as functional compounds for disease treatments.
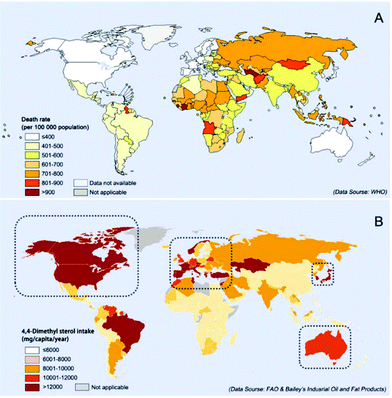 |
| Fig. 1 Comparison of death rates due to NCDs (A) and 4,4-dimethyl phytosterol intake in the world (B). Data of the age-standardized death rate (2015) due to NCDs are from the World Health Organization (WHO), produced by Information Evidence and Research (IER).15 Based on the 4,4-dimethyl phytosterols being mainly consumed from vegetable oils, the 4,4-dimethyl phytosterol intake is calculated from their content in vegetable oils. The consumption data of different vegetable oils in 2014 worldwide was reported by the Food and Agriculture Organization (FAO).16 The content of 4,4-dimethyl phytosterols in each kind of vegetable oil is obtained from Bailey's Industrial Oil and Fat Products (sixth edition).17 | |
2. Diversity of natural 4,4-dimethyl phytosterols
2.1. Structure of 4,4-dimethyl phytosterols
The key characteristics and structural peculiarities of 4,4-dimethyl phytosterols are shown in Fig. 2, which includes a 3β-hydroxyl (3β-OH) group and a side chain with variable numbers of carbons.
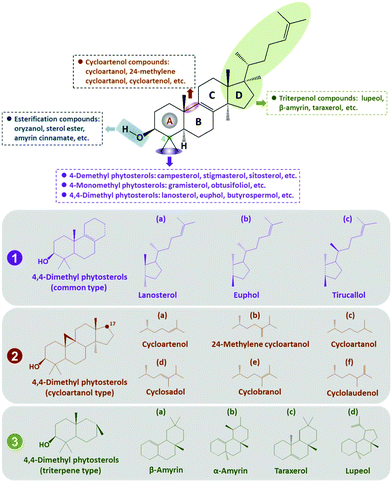 |
| Fig. 2 Summary of the structure–compound relationship of the major 4,4-dimethyl phytosterols. The 4,4-dimethyl phytosterols typically possess two methyl groups at the carbon-4 atom position of the aliphatic A-ring. Due to the structural differences in the aliphatic rings, 4,4-dimethyl phytosterols are further classified into a common type (1), a cycloartanol type (2), and a triterpene type (3). The dotted line and round spot in the ring indicated the linkage positions between the two parts. | |
The distinctive feature of 4,4-dimethyl sterols is the existence of two methyl substituents at the carbon-4 position in the aliphatic fragment. Similar to 4-desmethyl phytosterols, 4,4-dimethyl phytosterols also contain a 3β-OH group and one or several unsaturated bonds in the ring or the side chain. Esterification compounds of 4,4-dimethyl phytosterols are formed by coupling the 3β-OH group with free fatty acids, carbohydrates, or phenolic acid, thus forming the corresponding esters, such as oryzanol.
Until now, many different types of 4,4-dimethyl phytosterols have been identified, which vary mostly in their substitutents at carbon-9 and carbon-17 positions, and their hydrophobic side chains (Fig. 2). The chemical difference among the common type of 4,4-dimethyl phytosterols is attributed to the numbers of carbon atoms and the presence of unsaturated bonds in the side chain linked with carbon-17, such as lanosterol (1a), euphol (1b), tirucallol (1c), etc. Considering the structural distinction of unsaturated bonds, the substituents at the carbon-24 position, and the stereochemical properties, 4,4-dimethyl phytosterols are further divided by the cyclization at the carbon-9 position or variations of the divergent ring, thus forming the cycloartenol type of 4,4-dimethyl phytosterols (2a, 2b, 2c, 2d, 2e, 2f, etc.) and the triterpene type of 4,4-dimethyl phytosterols (3a, 3b, 3c, 3d, etc.).
2.2. Sources of 4,4-dimethyl phytosterols
Plants synthesize a more diverse range of 4,4-dimethyl phytosterols than animals or microorganisms. Considerable amounts of α-amyrin, β-amyrin, taraxerol and lupeol have been reported to be present in the plant kingdom. Some of the above 4,4-dimethyl phytosterols have been reported previously.19,20 However, in the current review, other sources of the 4,4-dimethyl phytosterols are further summarized and compared (Table 1). α,β-Amyrin mainly existed in plant resins of the Bursera and Protium species in the Burseraceae family.20 Lupeol is primarily distributed in vegetables, fruits and medicinal plants.21 Compared to α,β-amyrin, lupeol is commercially available, relatively low cost, and always of high yield. Moreover, many medicinal plants are also rich in taraxerol. A polar extract of Clitoria ternatea contains taraxerol at a content of 12.4 mg g−1.22 High contents of taraxerol can be extracted from Vaccinium oldhamii (22 μg g−1 dry twigs) and Eugenia umbelliflora (35 μg g−1 dry fruits).23,24 As for euphol, the fresh leaf of Euphorbia tirucalli could yield approximately 4.06 mg g−1 of euphol.25
Table 1 Sources of naturally occurring 4,4-dimethyl phytosterols
Sources |
4,4-Dimethyl phytosterols |
Ref. |
Represent the predominant compound in the corresponding source.
|
Plant source
|
Canavalia ensiformis seeds |
Lupeola, β-amyrin |
34
|
Rhizophora racemose leaves |
Lupeola, α-amyrin, β-amyrin |
37
|
Brassica oleracea leaves |
Lupeola, α-amyrin, β-amyrin, |
38
|
Bursera cuneate resin |
Lupeola, α-amyrin, β-amyrin |
39
|
Adenophora triphylla roots
|
Lupeola, taraxerol |
40
|
Vitex trifolia fruits |
Lupeol, α-amyrin, taraxerol |
41
|
Ricinus communis leaves
|
Lupeola, α-amyrin, β-amyrin |
42
|
Coccoloba uvifera leaves |
Lupeola, α-amyrin, β-amyrin |
43
|
Betula alleghaniensis leaves |
α-Amyrina, β-amyrin |
44
|
Canarium luzonicum resin |
α-Amyrina, β-amyrin |
45
|
Capsicum annuum skin |
α-Amyrina, β-amyrin, taraxerol |
46
|
Protium heptaphyllum resin |
α-Amyrina, β-amyrin |
47
|
Protium burseraceae resin |
α-Amyrina, β-amyrin |
48
|
Hieracium pilosella roots |
α-Amyrina, β-amyrin, taraxerol |
49
|
Bursera copallifera resin |
α-Amyrina, lupeol |
50
|
Zea mays leaves |
β-Amyrina, α-amyrin |
51
|
Nelumbo nucifera pollen |
β-Amyrina, α-amyrin |
52
|
Vaccinium myrtillus leaves |
β-Amyrin, 24-methylene cycloartanol, lupeol |
53
|
Leea macrophylla leaves |
β-Amyrina, lupeol |
54
|
Euphorbia kansui roots |
Euphola, β-amyrin, α-amyrin |
55
|
Synadenium grantii barks |
Euphola, lanosterol |
56
|
Mangifera indica barks |
Taraxerola, β-amyrin, α-amyrin |
57
|
Taraxacum officinale aerial |
Taraxerola, lupeol, β-amyrin |
58
|
Dioscorea tenuipes leaves |
Cycloartenola, α-amyrin, 24-methylene cycloartanol |
59
|
Vegetable oil source
|
Carrot root oil |
24-Methylene cycloartanola, cycloartanol, β-amyrin |
60
|
Rice bran oil |
24-Methylene cycloartanola, cycloartenol, cycloartanol |
61
|
Olive oil |
24-Methylene cycloartanola, cycloartenol |
62
|
Wheat germ oil |
24-Methylene cycloartanola, β-amyrin, cycloartenol |
63
|
Eggplant seed oil |
Cycloartanola, cycloartenol |
64
|
Camellia seed oil |
Cycloartanola, cycloartenol, 24-methylene cycloartanol |
65
|
Sea buckthorn seed oil |
Lanosterola, β-amyrin, cycloartenol |
66
|
In addition to plant sources, dietary phytosterols are mostly derived from vegetable oils, cereals, nuts, and fruits. Vegetable oil is predominantly used and is estimated to represent 40% of the total intake in China,26 and about 26% in the Netherlands and Sweden.27 The 4,4-dimethyl phytosterols, mostly 24-methylene cycloartanol and cycloartanol, are presented as free or esterified forms in various vegetable oils. Rice bran oil has already been recognized for its high content of 24-methylene cycloartanol (4.94 mg g−1) and cycloartenol (4.82 mg g−1).17 Soybean oil is also rich in cycloartenol (1.68 mg g−1), while the 24-methylene cycloartanol content in sesame seed oil reaches about 1.07 mg g−1.28 Moreover, the 24-methylene cycloartanol has been reported at a higher level in olive oil as compared to hazelnut oil.29 The quantitative analysis of 4,4-dimethyl phytosterols indicated a significant difference in the content of cycloartenol between different edible oils.30 Thus, the 4,4-dimethyl phytosterols can be utilized as a possible marker in the detection of oil adulteration. However, 4,4-dimethyl phytosterols from microbial and animal sources are limited due to their different biosynthetic pathways. Previous studies adopted bioengineering techniques to clone synthase genes of 4,4-dimethyl phytosterols from plants and express on microorganisms to produce the targeted 4,4-dimethyl phytosterols.31,32 Notably, the fat of sheep wool can be developed as a suitable source of lanosterol. The fresh wool contained about 20 mg g−1 of the total sterol, and lanosterol was about 1.84 mg g−1.33
Notably, the neutral lipid extracted from the seeds of Canavalia ensiformis is a promising source of lupeol. The triterpene alcohol fraction was mainly composed of lupeol (96.3%), with the minor constituent being β-amyrin.34 Additionally, the unsaponifiable matter of shea butter yielded the 4,4-dimethyl phytosterols as the predominant constituent, which consisted of α-amyrin (26.5%), lupeol (21.7%), and β-amyrin (10.2%).35,36 Overall, the major sources of naturally occurring 4,4-dimethyl phytosterols are presented in Table 1.
3. Health benefits of 4,4-dimethyl phytosterols
3.1. Anti-inflammatory activity
The inflammatory process would raise the level of cytokines such as tumor necrosis factor-α (TNF-α), interferon-γ (IFN-γ), interleukin (IL)-1β, IL-6 and IL-17A in serum, necrotic cells or damaged tissues.67 The 4,4-dimethyl phytosterols utilized as anti-inflammatory agents have been widely reported (Table 2).
Table 2
In vivo studies of the anti-inflammatory activity of 4,4-dimethyl phytosterols
Compound |
Inflammation |
Experimental design |
Administration |
Dose |
Outcomes |
Ref. |
Not specified, N.S.; intraperitoneal injection, i.p.; tumor necrosis factor-α, TNF-α; interleukin-6, IL-6; myeloperoxidase, MPO; thiobarbituric acid-reactive substances, TBARS; dimethylnitrosamine, DMN; tissue inhibitor of metalloproteinase-1, TIMP-1; complete Freund's adjuvant, CFA; interleukin-1β, IL-1β; transcription factor-κB, NF-κB; keratinocyte-derived chemokine, KC; cyclic AMP response element binding protein, CREB; cyclooxygenase-2, COX-2; trinitrobenzene sulfonic acid, TNBS; interleukin-10, IL-10; prostaglandin E2, PGE2; 12-O-tetradecanoyl-phorbol-13-acetate, TPA; dextran sodium sulfate, DSS; inducible nitric oxide synthase, iNOS; lipopolysaccharide, LPS; interferon-γ, IFN-γ; myelin oligodendrocyte glycoprotein, MOG35-55; monocyte chemoattractant protein-1, MCP-1; monocyte chemoattractant protein-2, MIP-2; nitric oxide, NO; and phospholipase A2, PLA2. |
Lupeol |
Ear oedema |
Male Swiss mice were topically treated with TPA (2.5 μg) to the right ear |
Topical |
0.5 and 1.0 mg per ear |
Nitrite↓, PGE2↓, TNF-α↓, IL-1β↓ |
69
|
Tirucallol |
Ear oedema |
Male Swiss mice were topically treated with TPA (2.5 μg) to the right ear |
Topical |
0.25, 0.5, and 1.0 mg per ear |
Weight edema↓, NO↓, PGE2↓, iNOS↓ |
71
|
α,β-Amyrin |
Periodontitis |
Nylon ligature was placed around the maxillary right tooth of male Wistar rats |
Oral |
5 and 10 mg kg−1 |
TNF-α↓, MPO↓, TBARS↓ |
79
|
β-Amyrin |
Hepatic fibrosis |
Male Wistar rats were injected (i.p.) with DMN in saline (10 μL kg−1) |
Oral |
30 mg kg−1 |
TNF-α↓, caspase-3↓, TIMP-1↓ |
80
|
Lupeol |
Allergic airway inflammation |
Male BALB/c mice were exposed in a box with aerosolized ovalbumin (1%) |
Oral |
60 mg kg−1 |
IL-4↓, IL-5↓, IL-13↓ |
83
|
α,β-Amyrin |
Colitis |
Male Swiss mice were injected (i.p.) with TNBS (25 mg mL−1, 100 μL) |
Intraperitoneal |
0.3, 1.0, and 3 mg kg−1 |
MPO↓, IL-10↓, IL-1β↓, COX-2↓, NF-κB↓, CREB↓ |
84
|
Taraxerol |
Paw oedema |
Male Wistar rats were injected (i.p.) with carrageenan (1%, 0.1 mL) |
Intraperitoneal |
5 and 10 mg kg−1 |
IL-1β↓, IL-6↓, IL-12↓, TNF-α↓, NF-κB↓, COX-2↓, iNOS↓ |
85
|
α,β-Amyrin |
Pancreatitis |
Male Wistar rats were injected (i.p.) with L-arginine (2.5 g kg−1) |
Oral |
10, 30, and 100 mg kg−1 |
TNF-α↓, IL-6↓, MPO↓, TBARS↓, nitrate/nitrite↓ |
86
|
α,β-Amyrin |
Pancreatitis |
Male Swiss mice were injected (i.p.) with cerulean (50 μg kg−1) |
Oral |
10, 30, and 100 mg kg−1 |
TNF-α↓, IL-6↓, MPO↓, TBARS↓ |
91
|
α-Amyrin |
Skin inflammation |
Male Swiss mice were topically treated with TPA (2.5 μg per ear) |
Topical |
0.1, 0.2, and 1.0 mg per ear |
NF-κB↓, PGE2↓, COX-2↓ |
92
|
α-Amyrin |
Ear oedema |
Male Swiss mice were topically treated with TPA (2.5 μg per ear) to the right ear |
Topical |
0.1, 0.5, and 1.0 mg per ear |
MPO↓, IL-1β↓ |
93
|
Lupeol |
Colitis |
Male Swiss mice were treated with 2% DSS in drinking water |
Intraperitoneal |
40 mg kg−1 |
MPO↓, TNF-α↓, IL-2↓, IL-6↓, NF-κB↓ |
94
|
Lupeol |
Colitis |
Male C57BL/6 mice were treated with 4% DSS in drinking water |
Oral |
50 mg kg−1 |
IL-12↓, IL-6↓, IL-1β↓, TNF-α↓, IL-10↑ |
95
|
Lupeol |
Neurogenic inflammation |
Male C57BL/6 mice were injected (i.p.) with LPS (250 μg kg−1 day−1) |
Oral |
50 mg kg−1 |
TNF-α↓, iNOS↓, IL-1β↓ |
96
|
Lupeol |
Pleuritis |
Male Swiss mice were injected (i.p.) with carrageenan (1%, 0.1 mL) |
Oral |
25, 50, 100, and 200 mg kg−1 |
IL-2↓, IFN-γ↓, TNF-α↓ |
97
|
Euphol |
Colitis |
Male Swiss CD-1 mice were treated with 3% DSS or TNBS (15 mg mL−1, 100 μL) |
Oral |
3, 10, and 30 mg kg−1 |
MCP-1↓, CXCL/KC↓, IL-1β↓, MIP-2↓, TNF-α↓, IL-6↓, IFN-γ↓, IL-10↑ |
98
|
Taraxerol |
N. S. |
Male Wistar rats were fed with HFD and STZ (i.p. 35 mg kg−1) |
Oral |
20 mg kg−1 |
MCP-1↓, IL-1β↓, IL-6↓, TNF-α↓, NF-κB↓ |
99
|
Cycloartenol |
Hind-paw oedema |
Male rats were injected (i.p.) with carrageenan (0.1 mL, 1%) to the right hind paws |
Oral |
10, 20, and 40 mg kg−1 |
PLA2↓, leucocyte migration↓ |
100
|
Cycloartenol, 24-methylene cycloartanol |
Ear oedema |
Female ICR mice were topically treated with TPA (1.0 μg) to the right ear |
Topical |
0.2 and 0.3 mg per ear |
Ear thickness↓ |
101
|
Euphol |
Skin inflammation |
Male Swiss CD-1 mice were topically treated with TPA (2.5 μg) to the right ear |
Topical |
50 and 100 μg per ear |
MPO↓, NAG↓, COX-2↓, CXCL/KC↓, MIP-2↓ |
109
|
Gupta et al. firstly found that the 4,4-dimethyl phytosterols exerted an anti-inflammatory activity against arthritis and oedema.68 Since then, 4,4-dimethyl phytosterols have been extensively demonstrated to exhibit anti-inflammatory activities. Several studies conducted by Fernandez et al. indicated that the 4,4-dimethyl phytosterols were effective in alleviating the 12-O-tetradecanoyl-phorbol-13-acetate (TPA)-induced ear oedema.69–71 Lupeol pretreatment decreased the myeloperoxidase (MPO) activity, the cell infiltration in damaged tissues, and prostaglandin E2 (PGE2) in macrophagocytes.69 The related mechanism was similar to that of indomethacin, which is an effective inhibitor of cyclooxygenase (COX).70 Tirucallol also prevented the inflammation via a mechanism related to the reduction of nitric oxide (NO) and inducible nitric oxide synthase (iNOS).71 Additionally, taraxerol and β-amyrin exhibited a similar anti-inflammation effect on ear oedema. However, α-amyrin and 24-methylene cycloartanol exhibited higher inhibition capacity.72
The anti-inflammation benefits of 4,4-dimethyl phytosterols were associated with the modulation of the immune system and reduction of cytokines in an arthritis model.73,74 Lupeol could decrease the collagen, thereby increasing the activities of lysosomal enzymes.75 Though the detailed mechanism was not given, the CD4+ T cell-mediated autoimmune response is critical in the prevention of arthritis.76 Eliminating CD4+ T cells would contribute to the therapy of arthritis.77 Therefore, a subsequent study focused on CD4+ T cells indicated that lupeol could modulate the phagocytic function of T-lymphocytes and macrophages, thus inhibiting the generation of pro-inflammatory cytokines.78 The above results illustrated that lupeol was effective at suppressing T cells, which is suggestive of future utilization. Moreover, α,β-amyrin modulated the periodontal inflammation through inhibiting neutrophil infiltration and oxidative stress.79 However, β-amyrin treatment alone attenuated the altered oxidative stress, TNF-α levels, and protein expression.80 β-Amyrin acetate was found to exert a stronger anti-inflammatory activity than α-amyrin acetate.68,81 The above studies indicated that β-amyrin might exhibit a higher anti-inflammatory activity. However, another study showed an opposite result on the inhibition of TPA-induced inflammation.72 The inconsistent results can be attributed to the different inflammatory models utilized.
The 4,4-dimethyl phytosterols were also compared with some anti-inflammatory compounds (e.g. α-mangostin, dexamethasone, and methylprednisolone). The comparison of lupeol and α-mangostin demonstrated that lupeol exhibited 57.14% inhibition, while α-mangostin at a similar dose showed only 38.70% inhibition.82 Similarly, in allergic airway inflammation, lupeol was observed to be as effective as dexamethasone, and significantly decreased the generation of mucus and pro-inflammatory cytokines in the lungs.83 Another study also showed a similar result in reversing the colitis with α,β-amyrin, which indicated that the inhibition of transcription factor-κB (NF-κB) and the cyclic AMP response element binding protein (CREB) was the possible mechanism.84 However, only when treated with a higher dose at 10 mg kg−1, the inhibitory activity of taraxerol was comparable to that of dexamethasone (0.5 mg kg−1).85 Based on these results, the α,β-amyrin might exhibit a stronger activity than taraxerol, which was also confirmed in the study conducted by Akihisa et al.72 Moreover, in a pancreatitis model, the activity of α,β-amyrin was equivalent to that of methylprednisolone,86 and the esterified derivatives of 4,4-dimethyl phytosterols (acetate, palmitate and linoleate) have been reported to display higher anti-inflammatory activities.87–89 Esterification can enhance the activity by increasing the diffusion, absorption, and retention capacity, which might explain the higher anti-inflammatory activities of the esterified derivatives.
The 24-methylene cycloartanol and α-amyrin exerted the strongest activity with an IC50 dose of 0.2 mg per ear.72 Similarly, Yasukawa et al. indicated that the 24-methylene cycloartanol exhibited the highest suppression activity on tumor growth.90 In brief, these results confirmed the health benefits of 4,4-dimethyl phytosterols in various inflammatory models, suggesting that the supplementation with 4,4-dimethyl phytosterols is a potential approach to treat inflammation. However, it should be mentioned that the inconsistent results still exist, and more studies are thus needed to explore the anti-inflammatory difference between different 4,4-dimethyl phytosterols, and to determine which kind of 4,4-dimethyl phytosterol can treat the inflammation in the target.
3.2. Anticancer activity
Topical application of 4,4-dimethyl phytosterols modulated the pathways involved in growth, survival and apoptosis in several models of skin cancer.102,103 Considering that the NF-κB, phosphatidyl inositol 3-kinase (PI3K)/Akt pathway plays a critical role in cancer promotion, Saleem et al. proved that lupeol showed anti-skin cancer ability by inhibiting the activation of NF-κB and PI3K/Akt.103 Moreover, cycloartenol is another effective 4,4-dimethyl phytosterol for treating skin cancer. Cycloartenol could suppress the benzoyl peroxide and ultraviolet-B radiation-induced cellular oxidative stress, and reverse the induction of cutaneous ornithine decarboxylase (ODC) activity.104 Euphol could prevent the tumor-promoting effect of TPA initiated with 7,12-dimethylbenz[a]anthracene (DMBA) by reducing the levels of keratinocyte derived chemokine (CXCL1/KC) and macrophage inflammatory protein-2 (MIP-2), which are related to the expression of protein kinase C.90,102 Several anti-cancer effects of 4,4-dimethyl phytosterols were compared.105 Taraxerol exhibited the most potent anti-tumor activity as compared to α-amyrin, β-amyrin, and lupeol. A surprising observation is that the anticancer activity of taraxerol acetate was weaker than that of taraxerol,105 which is different from the anti-inflammatory effect and remains to be reconfirmed.
In addition to skin cancer, 4,4-dimethyl phytosterols exhibited anticancer effects in many other cancers, including oral cancer, bladder cancer, liver cancer, colon cancer, etc. Ovadje et al. investigated several 4,4-dimethyl phytosterols for their effects on colon cancer.106 Compared to β-amyrin and lupeol, α-amyrin was more effective at decreasing the viability of colon cancer cells. No synergistic interactions between different 4,4-dimethyl phytosterols were observed. Lanosterol inhibited the formation of colonic aberrant crypt foci (ACF) and crypt multiplicity.107 Prasad et al. found that lupeol significantly decreased cyclooxygenase-2 (COX-2) in the bladder and nuclear matrix protein 22 (NMP22) in urine.108 Lupeol was also effective at inhibiting stress-induced liver injury through moderating apoptosis factors. Meanwhile, lupeol could decrease the peroxidation levels and restore the antioxidant enzymes in favor of the excretion of carcinogenic metabolites, thus inhibiting tumor growth.108 Hong et al. investigated the anticancer effect of taraxerol ester in U87 human glioblastoma cells. Taraxerol acetate significantly reduced the tumor weight and size in a xenograft model.109 Therefore, the above results proved that the 4,4-dimethyl phytosterols are effective anticancer agents.
3.3. Anti-Alzheimer's and anti-Parkinsonian activities
Alzheimer's disease (AD) and Parkinson's disease (PD) are the two most prevalent and severe neurological diseases which are basically disorders in the functions of nervous systems.110 No effective drug as yet could provide complete therapy for PD and AD. The 4,4-dimethyl phytosterols have been reported to exert biological functions against AD and PD.
The inhibition of acetylcholinesterase (AChE) was believed to improve the condition of AD patients.111 The activity of AChE could be inhibited by β-amyrin and taraxerol, but not α-amyrin.112,113 However, the expression level of memory-related signal transduction proteins was significantly enhanced by both α-amyrin and β-amyrin in the hippocampus, which suggested that α-amyrin and β-amyrin ameliorated the cognition disorders through the activation of extracellular signal-regulated kinase 1/2 (ERK) and glycogen synthase kinase-3β (GSK-3β), as the ERK inhibitor (U0126) blocked this effect.114 Molecular docking studies revealed that taraxerol had a high affinity for fibrils and β-amyloid peptides, but low toxicity, and confirmed the ability of taraxerol in the inhibition of AChE activity.113,115 A recent in vivo study further proved the effects of taraxerol on the AChE activity and memory deficits. Taraxerol pretreatment significantly improved memory impairment in the hippocampus induced by scopolamine and streptozotocin.112 The mechanism was related to the activation of the receptor system of AChE.
Additionally, β-site amyloid cleaving enzyme 1 (BACE1) was also involved in the rate-limiting step in the pathogenesis of AD, which motivated researchers to target BACE1 for AD prevention.116 Lupeol was confirmed via enzymatic evaluation and molecular dynamics simulations, holding therapeutic potential for AD treatment through the inhibition of BACE1.117,118 Notably, lupeol produced higher flexibilities of the protein and local fluctuations in the active site of BACE1, which further implied that the binding activity of lupeol is stronger than that of ursolic acid.117 Recently, lupeol treatment has significantly decreased the diabetes-related disorders of AD, such as cognitive impairment, anxiety and fear-associated behaviors.119 In addition to AD, the relocation of lanosterol synthase from the endoplasmic reticulum to mitochondria, suggesting that lanosterol exhibited anti-Parkinsonian activity probably by protecting the dopaminergic neurons from death.120 Moreover, the anti-Parkinsonian mechanism of β-amyrin may be related to autophagy involving LGG-1.121 Autophagy has been reported to be a therapeutic approach to prevent PD owing to the elimination of the misfolded proteins and injured organelles.122 In the process of autophagosome formation, LGG-1 exited for structural maintenance; hence it is utilized as a potential marker.123 It was found that the β-amyrin participated in the LGG-1 related autophagy pathway through improving the LGG-1 expression and the amounts of localized LGG-1 puncta.121 Furthermore, β-amyrin exhibited a protective effect on dopaminergic neurons by reducing cell damage, and α-synuclein aggregation, which was also involved in the symptoms of PD.124
It is noteworthy that the previous studies have linked sterol-mediated cholesterol metabolism to neurological diseases. Low levels of LDL-C were usually associated with a higher occurrence of AD and PD.125 Thus, the anti-Alzheimer's and anti-Parkinsonian activities of 4,4-dimethy sterols may also be associated with their ability in regulating cholesterol metabolism. Taken together, these results confirmed the effect of anti-neurological disease and indicated that 4,4-dimethyl phytosterols had potential for therapeutic applications in memory impairment.
3.4. Anti-hyperglycaemic and anti-hypolipidemic activities
Hyperglycemia and hyperlipidemia are often present with chronic inflammation and obesity. The 4,4-dimethyl phytosterols exert anti-hyperglycemic and anti-hypolipidemic activities in obesity related metabolic disorders.
Santos et al. investigated the benefits of α,β-amyrin in high fat diet (HFD)-induced obesity.126 The α,β-amyrin significantly reduced the content of total triacylglycerol (TG), total cholesterol (TC), low-density lipoprotein cholesterol (LDL-C), and very low-density lipoprotein (VLDL), while elevating the level of high-density lipoprotein cholesterol (HDL-C) in serum. They further indicated that the beneficial activity of α,β-amyrin involved the protection of beta-cells. Subsequent research proposed a possible mechanism through investigating the effect of α,β-amyrin on the contractile and relaxant vascular responses. Treatment with α,β-amyrin prevented body weight gain, insulin resistance, dyslipidemia, and the endothelial dysfunction, probably through a restoration of the guanylate cyclase-K+ channel pathway.127 Carvalho et al. found that α,β-amyrin ameliorated HFD-induced obesity via regulating the enzymatic, hormonal, and inflammatory responses, and decreased the adipocyte area, visceral adiposity, and the blood lipase activity.9 These results indicated the potential of α,β-amyrin in the therapy of obesity and related disorders.
PPAR-α is critical for the regulation of lipids and glucose metabolism, acting as a sensor of the fatty acid load.128 Elevation in the circulating fatty acids or the metabolites results in the activation of PPAR-α, which, in turn, increases the activities of enzymes involved in β-oxidation. Prabhakar et al. found that α-amyrin significantly increased the mRNA expression and protein level of PPAR-α, leading to increased lipid metabolism and deposition in the liver.129 Ardiansyah et al. also indicated that lupeol improved lipid metabolism by decreasing the mRNA expression of the genes involved in lipid synthesis in the liver.130 But the function was independent of the benefits in blood pressure amelioration. Both lupeol and its ester ameliorated the hypercholesterolemic condition, while lupeol linoleate was more effective.14 The mechanism might be due to the reduced absorption and enhanced excretion of cholesterol.131
Daisuke et al. indicated that the 24-methylene cycloartanol and cycloartenol prevented obesity through controlling the release of glucose-dependent insulinotropic polypeptide (GIP).132 The supplementation with cycloartenol and 24-methylene cycloartanol caused less body weight gain, promoted fat utilization, and enhanced the expression of genes associated with lipid oxidation, whereas it suppressed the gene expression related to lipid synthetase and sterol regulatory element-binding protein (SREBP)-1c. A later study also revealed that the 24-methylene cycloartanol and cycloartenol decreased the postprandial hyperglycemia by decreasing glucose absorption in the intestine both in mice and humans.133 In addition to the abovementioned 4,4-dimethyl phytosterols, lanosterol at a 1% dose did not show any significant effect on serum lipid profiles, while the HDL-C level was significantly upregulated at a 2% dose.107 Moreover, taraxerol restored the abnormal changes in type 2 diabetes.99 Overall, the proposed underlying mechanism of the anti-hyperglycemic and anti-hypolipidemic effects in obesity-related metabolic disorders is presented in Fig. 3.
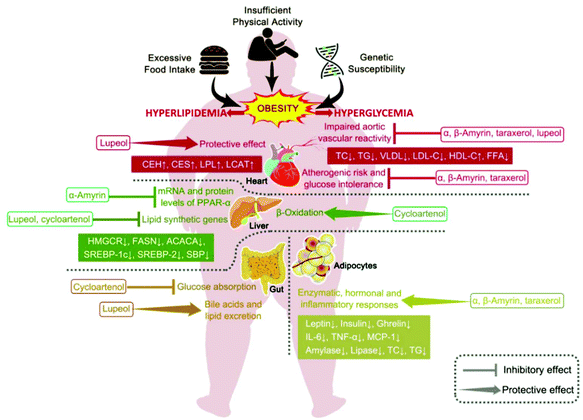 |
| Fig. 3 Proposed mechanism underlying the anti-hyperglycaemic and anti-hypolipidemic effects by 4,4-dimethyl phytosterols. Obesity is commonly caused by a combination of excessive food intake, insufficient physical activity, and genetic susceptibility. 4,4-Dimethyl sterols exert a potential efficacy against obesity through multiple mechanisms involved in heart protection, lipid metabolism, adipocyte response, and gut functions. Total cholesterol, TC; total triacylglycerol, TG; very low-density lipoprotein, VLDL; low-density lipoprotein cholesterol, LDL-C; high-density lipoprotein cholesterol, HDL-C; free fatty acid, FFA; cholesterol ester hydrolase, CEH; lecithin/cholesterol acyl transferase, LCAT; cholesterol ester synthase, CES; lipoprotein lipase, LPL; peroxisome proliferator activated receptor-α, PPAR-α; 3-hydroxy-3-methylglutaryl coenzyme A reductase, HMGCR; fatty acid synthase, FASN; acetyl-coenzyme A carboxylase α, ACACA; sterol regulatory element binding protein-1c, SREBP-1c; sterol regulatory element binding protein-2, SREBP-2; steroid binding protein, SBP; interleukin-6, IL-6; tumor necrosis factor-α, TNF-α; and monocyte chemoattractant protein-1, MCP-1. | |
3.5. Antinociceptive activity
A previous study showed that euphol prevented the mechanical nociceptive activity induced by ligation through decreasing the level of cytokines.134 The proposed mechanisms revealed by Dutra et al. include: (i) modulation of the protein kinase C epsilon (PKCε) pathway; (ii) activation of NF-κB and CREB; and (iii) production of algesic proteins and pain mediators.135 In another study, lupeol was found to exhibit antinociceptive effects that can be used against inflammatory pain, but not post-operative pain, through the reduction of IL-1β and TNF-α.136 Interestingly, toxicity studies of lupeol indicated that no systemic toxicity and no central depressant symptom or mortality were observed even at the highest effective dose (100 mg kg−1) for 7 days.137,138 However, compared to indomethacin, lupeol did not exhibit antinociceptive activity.139 This result might suggest a different antinociceptive mechanism between lupeol and indomethacin.
Oliveira et al. utilized two models of visceral nociception to evaluate the responses after oral pretreatment with α,β-amyrin. The mixture neither altered the anesthesia time, nor affected the motor coordination.140,141 Thus, the antinociceptive mechanism of α,β-amyrin is probably involved in the opioid system and the vanilloid receptor (TRPV1). Similarly, the 24-methylene cycloartanol produced a marked and dose-dependent reduction of acetic acid-induced abdominal constriction. The function was also through a coordination with the opioid system, but not an interaction with a peripheral or central depressant.142 Otuki et al. found that α,β-amyrin given by several routes prevented the capsaicin-induced neurogenic nociception, and indicated that α,β-amyrin exerted an effective antinociceptive activity, particularly the inflammatory nociception.93 The mechanism seems to be related to the regulation of the protein kinase A- or protein kinase C-sensitive pathway. These results presented herein suggest that 4,4-dimethyl phytosterols exhibit potent antinociceptive activity, hence constituting a promising perspective to pharmacological development.
3.6. Other functions
Except for the abovementioned benefits, 4,4-dimethyl phytosterols have been reported to exhibit other pharmacological activities, such as anti-cataract,143,144 anti-fertility,145,146 anti-microbial,147,148 anti-depressant,149 and anti-viral activities.150 Lupeol pretreatment could decrease the level of malonaldehyde (MDA) and reactive oxygen species (ROS) in lenses, which indicated that the anti-cataract benefit might be attributed to the antioxidant activities.143 Additionally, lanosterol was proved to be effective for the prevention of protein aggregation, and may be developed as a promising therapeutic strategy for cataract.144
In addition to cataract, Gupta et al. demonstrated that lupeol and amyrin in their acetate form caused a significant decrease in the weights of reproductive organs. Sperm motility and sperm density were also decreased, leading to a complete prevention of fertility.145,146 To further investigate the anti-fertility activity, Mannowetz et al. found that lupeol regulated the activation of the calcium channel of sperm (CatSper) via the modulation of α/β hydrolase domain-containing protein 2 (ABHD2).151 ABHD2 is an important enzyme appearing in the endogenous cannabinoid system (ECS). Recent literature reports tentively discovered that a potential linkage existed between the benefits of 4,4-dimethyl phytosterols and the ECS, which is discussed in the following section.
4. Exploring the linkage between 4,4-dimethyl sterols and the ECS
4.1. A glimpse of the ECS
Numerous studies have been conducted to clarify the biological role of the ECS, regarding its function in NCDs, and the potential of its pharmacological exploitation. The ECS comprises (i) two G protein coupled cannabinoid receptors, CB1R and CB2R; (ii) their ligands, which are lipid molecules, 2-arachidonoylglycerol (2-AG) and N-arachidonylethanolamide (AEA); and (iii) the enzymes that regulate endocannabinoid biosynthesis and degradation, such as fatty acid amide hydrolase (FAAH) and monoacylglycerol lipase (MAGL).152,153 AEA is produced via phospholipase D (PLD)-mediated hydrolysis of N-arachidonoyl phosphatidylethanolamine, and degraded by the FAAH,154 while 2-AG is synthesized via diacylglycerol lipase (DAGL)-mediated hydrolysis of diacylglycerol (DAG), and degraded by MAGL.155 Other serine hydrolases were also involved in the degradation of 2-AG, such as ABHD2.156
CB1R is intensively expressed on both glutamatergic and GABAergic interneurons in the peripheral and the central nervous systems, and has been widely followed in the maintenance of homeostasis in health. But postsynaptic localization for CB1R was also indicated.157,158 They are accountable for the psychoactive properties induced by tetrahydrocannabinol (Δ9-THC), and are critical in analgesic action and energy regulation. Furthermore, CB1R also exists in microglia, astrocytes and oligodendrocytes for the control of inflammatory reaction and further participates in the role in the nervous system.159 Recently, the alteration of the ECS or over-expression of CB1R was associated with various diseases, such as inflammatory diseases, obesity, diabetes and Alzheimer's disease.160 4,4-Dimethyl phytosterols have been attentively illustrated to regulate the ECS, thus exhibiting many beneficial functions.
4.2. ECS ameliorated by 4,4-dimethyl phytosterols
Euphol was the first 4,4-dimethyl phytosterol discovered to exert beneficial effects on the ECS. King et al. screened various commercial chemical compounds and found that euphol inhibited MAGL through interacting with a novel regulatory region in a reversible behavior.161 A later study found that euphol exerted an anti-inflammatory function by ameliorating the ECS. Euphol inhibited mechanical hyperalgesia, and it did not cause any side effects in the central nervous system.134 However, the antagonists of CB1R or CB2R, and the knockdown gene of CB1R and CB2R, significantly blocked the beneficial effect.134 Similarly, pretreated α,β-amyrin together with the antagonists or knockdown gene of the cannabinoid receptors also significantly prevented their anti-nociceptive effect.162 Thus, the literature revealed that euphol and α,β-amyrin could directly activate CB1R and CB2R. To further investigate how α-amyrin and β-amyrin were coordinated with the ECS, a colitis mouse model was utilized by Matos et al. They showed that α,β-amyrin significantly inhibited colonic damage, and the pro-inflammatory mediators.163 But different from the results obtained by Dutra et al.134 and Silva et al.,162 Matos et al. found that the pharmacological blockade of CB1R, but not CB2R, prevented the beneficial activity of α-amyrin and β-amyrin. Besides, the expression of MAGL and FAAH was significantly decreased with the pretreatment of α,β-amyrin.163 Based on these results, the study conducted by Chicca et al. examined the binding interactions between cannabinoid receptors and α,β-amyrin using several cells and purified enzymes.164 They found that β-amyrin was more potent than α-amyrin, and exerted its analgesic functions via inhibiting the endocannabinoid compound without directly interacting with endocannabinoid receptors. Meanwhile, it was deduced that the geminal methyl groups of β-amyrin in the A ring were more effective than the vicinal methyl groups of α-amyrin. β-Amyrin inhibited 2-arachidonoylglycerol (2-AG) hydrolysis without directly targeting cannabinoid receptors.164
Additionally, lupeol was discovered to inhibit ABHD2 activity. CatSper played a significant role in the motility and fertility of sperm.165 Lupeol could activate CatSper of sperm through binding with ABHD2, and thus could be utilized as a contraceptive compound for fertilization prevention.151 As for the activity evaluation, a recent study proved that α-amyrin and β-amyrin potently decreased the MAGL activity in vitro, but the difference of IC50 was not statistically significant.166 Previous studies found that α-amyrin and β-amyrin were comparable to synthetic compounds,162 and α,β-amyrin was 200–300 fold more potent than Δ9-THC.164 Based on these reports, the endowed health benefits of the ECS involved in 4,4-dimethyl phytosterols are summarized in Table 3 and Fig. 4.
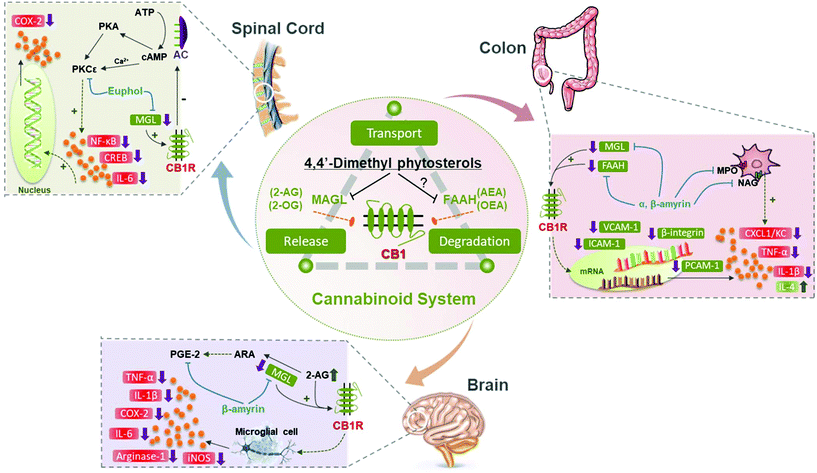 |
| Fig. 4 The ECS involved in 4,4-dimethyl phytosterols endowed healthy benefits in the body. The cannabinoid system involves the release, transport and degradation of various endocannabinoid compounds, such as 2-AG or 2-OG mediated by MAGL, and AEA or OEA mediated by FAAH. The 4,4-dimethyl sterols can interact with CB1R, thus regulating the endocannabinoids in different tissues. In the spinal cord, euphol inhibits MAGL activity and up-regulates 2-AG, which binds to CB1R further inhibiting the PKCε pathway, thus decreasing the activation of NF-κB, CREB, and COX-2 production. In the colon, α,β-amyrin inhibited the activity of MAGL and FAAH by modulating CB1R activation, thus reducing the pro-inflammatory cytokines and expression of the adhesion molecule mRNA. In the brain, β-amyrin exerts beneficial effects by inhibiting 2-AG hydrolysis, but not FAAH. Whether the 4,4-dimethyl sterols could inhibit FAAH activity remains to be confirmed. N-Arachidonylethanolamide, AEA; oleoylethanolamide, OEA; cannabinoid receptor 1, CB1R; fatty acid amide hydrolase, FAAH; monoacylglycerol lipase, MAGL; 2-arachidonoylglycerol, 2-AG; 2-oleatemonoglycerol, 2-OG; cyclooxygenase-2, COX-2; protein kinase C epsilon, PKCε; adenosine triphosphate, ATP; protein kinase A, PKA; cyclic-adenosine monophosphate, cAMP; adenylate cyclase, AC; nuclear factor-κB, NF-κB; cyclic AMP response element-binding protein, CREB; interleukin-6, IL-6; intercellular adhesion molecule 1, ICAM-1; myeloperoxidase, MPO; tumor necrosis factor-α, TNF-α; N-acetylglucosaminidase, NAG; interleukin-1β, IL-1β; interleukin-4, IL-4; interleukin-6, IL-6; platelet cell adhesion molecule 1, PCAM-1; inducible nitric oxide synthase, iNOS; keratinocyte-derived chemokine, CXCL1/KC; arachidonic acid, ARA; prostaglandin E2, PGE2. (+), promotion; (−), downregulation; (⊢), inhibition; (↑), increase; (↓), decrease. | |
Table 3 Health effects of 4,4-dimethyl phytosterols and the ECS involved
Compound |
ECS response |
Model |
Description |
Ref. |
Monoacylglycerol lipase, MAGL; cannabinoid receptors, CB; cannabinoid receptor 1, CB1R; cannabinoid receptor 2, CB2R; 2-arachidonoylglycerol, 2-AG; fatty acid amide hydrolase, FAAH; dextran sodium sulfate, DSS; and α/β hydrolase domain-containing protein 2, ABHD2. |
Euphol, 30 and 100 mg kg−1 p.o. |
Interaction with CB1R and CB2R |
CB1R and CB2R knockdown mice |
Anti-nociceptive effect was reversed by pre-treatment with either CB1R or CB2R antagonists, as well as the knockdown gene of CB1R and CB2R. |
134
|
Lupeol, IC50 109 nM |
ABHD2 activity↓ |
Purified human spermatozoa |
Significantly reduced the activation of the calcium channel of sperm by either progesterone or pregnenolone sulfate. |
151
|
Euphol, 10 μM |
MAGL activity↓ |
Rat neuron culture |
Inhibited MAGL activity with high potency through a reversible mechanism. |
161
|
α,β-Amyrin, 30 mg kg−1 p.o. |
Activation of CB1R and CB2R |
Mice and rats |
Directly bound with high affinity to CB1R and lower affinity to CB2R. |
162
|
α,β-Amyrin, 10 mg kg−1, i.p. |
MAGL activity↓ FAAH activity↓ |
DSS-induced colitis in mice |
mRNA expression for MAGL and FAAH was significantly reduced in the colon. CB1R, but not CB2R, pharmacological blockade reversed the beneficial effects. |
163
|
β-Amyrin, 1 and 10 μM |
2-AG content↑ MAGL activity↓ |
CHO-K1 cells, BV2 cells, pig brain |
Inhibited the degradation of the endocannabinoid 2-AG without interacting directly with CB receptors. |
164
|
α,β-Amyrin, lupeol |
MAGL activity↓ |
MAGL assay in vitro |
Relative potency of MAGL inhibition as determined by IC50 was as follows: α-amyrin (487.4 ng mL−1), β-amyrin (676.7 ng mL−1) and lupeol (785.4 ng mL−1). |
166
|
Overall, 4,4-dimethyl phytosterols offer broad and largely unexplored benefits for preventing various diseases and provide new insights into the underlying relationship with the ECS. The identification of the core region of MAGL provided a target for the characterization of potent inhibitors. King et al. revealed that a common pocket in euphol was found to be in the α-helical lid domain of MAGL, and it interacts reversibly with neighboring Cys201 and Cys208 present at the border of such a pocket.161 Although the observed activity through the interaction via MAGL offered an explanation of the ameliorating activity, the detailed mechanism needs further investigation. Recently, it has been demonstrated that the sterol A-ring plasticity is a critical factor for the governing hydrophobic interaction and molecular recognition substrate.
Sterol A-ring variants exhibit various substrate activities. Among all the assayed variant structures, contracted A-ring sterol exerts the highest activity.167 The 4,4-dimethyl phytosterols possess two methyl substituents at the carbon-4 position of the A-ring. The methyl substituent is attached with great significance in the rational drug design. The increased lipophilicity by methylation can drastically change the bioavailability and thus the efficacy of a bioactive molecule and its mode of interaction with the bioreceptors.168 A previous study suggested that the introduction of a methyl group to imidazolinediones increased the lipophilicity and ability to dislocate rimonabant (SR-141716A), which was a specific antagonist for CB1R.169 In addition, 4,4-dimethyl substituents could also influence the physical properties of biological membranes. The molecular structure of a sterol determined the ability to induce phase separation and the curvature of the formed liquid-ordered phase. In particular, the methylated sterols were able to induce domain formation, which might play an important role in the cell-biological processes.170 For example, increasing the methyl groups at the carbon-4 position would render the phytosterol less efficient in lowering glucose permeability.171 Another important effect of 4,4-dimethyl groups may rely on severe steric hindrance to the 3β-OH group of sterols. In the biological membrane, a 3β-OH group seems to be a prerequisite for a specific interaction in the membrane.172In vitro chemical assays indicated that the steric hindrance caused by the presence of ortho-methyl groups could influence the hydrogen-bonding interactions.173,174 The steric hindrance caused by the extra methyl group stabilized the hydrogen bonding network, and prevented the ionization of the neighboring amino acid residues. A extra methyl group forced OH to rotate and donate a hydrogen bond to other functional residues.175 Thus, the methyl groups in the A-ring might play an important role in the function of 4,4-dimethyl phytosterols, while further confirmation is still necessary.
5. Clinical trials
To test the clinical utility of 4,4-dimethyl phytosterols in treating diseases in humans, the unsaponifiable fraction from unripe fruits of Olea europaea, containing the maximal content of 4,4-dimethyl phytosterols (lanosterol, cycoartanol, and α,β-amyrin), was utilized in a small pilot clinical study with five patients affected by osteoarthrosis. According to the clinical results, treatment with the ointment prevented the hand and knee inflammatory symptoms in all patients without any adverse reactions.176 In another trial, Okahara et al. proved that the consumption of brown rice with a high content of cycloartenol and 24-methylene cycloartanol led to a lower postprandial hyperglycemia.133 The postprandial blood glucose level was significantly decreased by treatment with 24-methylene cycloartanol- and cycloartenol-enriched rice. Additionally, lupeol has been widely studied in clinical trials. Twenty-eight patients were enrolled in a non-randomized pilot study to evaluate lupeol in treating actinic keratosis, which may develop into skin cancer if left untreated. More than 75% of the injuries were cured by a lupeol-containing birch bark ointment.177 Currently, clinical trials conducted by Seoul National University Hospital,178 have already indicated that 2% of lupeol cream could treat patients suffering from mild–moderate face acne. In another case, lupeol promoted the focal lysis of tumors when injected at primary sites. Administration of lupeol treated malignant melanomas successfully.179
However, excess accumulation of 4,4-dimethyl phytosterols might cause various malformation syndromes. For example, lanosterol is the precursor substance of cholesterol, which is the first sterol in the cholesterol synthesis pathway. Malformation syndromes caused by inborn errors of cholesterol synthesis have been reviewed in detail.180,181 Additionally, Fang et al. proved that 2 μg ml−1 lanosterol not only failed to support the growth of auxotrophic mammalian cells, but also killed wild type mammalian cells.182 A recent study also suggested a moderate toxic potential for lupeol by targeting to the estrogen-receptor (ERα) involved in fertility, which could trigger undesired adverse effects.183 Therefore, the safe dose and long-term side effects warrant further investigation before these 4,4-dimethyl phytosterols can be recommended as a treatment option.
Recent work showed that the ATP-binding cassette transporter (ABCA1) can efficiently efflux lanosterol. Lanosterol could be chosen as a preferred substrate for ABCA1. However, lanosterol was hardly a substrate of the acyl-coenzyme A:cholesterol acyltransferase (ACAT) because lanosterol contains the gem dimethyl groups, which could cause a severe steric hindrance to 3β-OH.184 This property incapacitates the ability of ACAT1 to transport lanosterol in the endoplasmic reticulum. Considering that we can only take in phytosterols orally, there has been almost no literature about the absorption mechanism of other 4,4-dimethyl phytosterols. Therefore, further investigation is necessary to study the related mechanisms.
6. Future directions
The clinical trials demonstrated that the 4,4-dimethyl phytosterols can be exploited as prospective targets for the prevention of various diseases. However, many considerations still require further exploration, such as the appropriate dose, duration of exposure, and relative bioavailability. Most of the studies have attempted to show the benefits by using animal models. It seems that there still exists a research gap in investigating in humans when applied as functional foods or nutraceuticals. Therefore, in addition to animal models, future studies should be directed towards the clinical evaluation of 4,4-dimethyl phytosterols. A collaboration between food scientists and medical researchers is recommended. Furthermore, research is still needed to confirm the potential relationship between 4,4-dimethyl phytosterols and the ECS, as well as the detailed mechanisms. For example, the 4,4-dimethyl phytosterols are effective against PD, and future studies should focus on whether the anti-Parkinsonian effect is a direct consequence of ECS-mediated mechanisms, or simply the result of other functions, such as anti-inflammatory activity.
7. Summary
4,4-Dimethyl phytosterols are a class of bioactive compounds commonly found in plants and vegetable oils, which are suitable sources for convenient extraction or dietary consumption. From the above discussion, it is obvious that the naturally occurring 4,4-dimethyl phytosterols show great promise in the fight against various diseases such as inflammation, Parkinsonism and Alzheimer's disease, cancer, cataract, etc. The in-depth mechanism is likely to involve the ECS. Moreover, the clinical studies of 4,4-dimethyl phytosterols were further reviewed to support their potential development as therapeutic agents for multiple diseases. However, there still exists a research gap in exploring the benefits of 4,4-dimethyl phytosterols in humans when applied as functional foods or nutraceuticals. Thus, more clinical studies should be considered in the future.
Author contributions
Qingzhe Jin and Hui Zhang contributed to the conception of the manuscript. Tao Zhang and Ruijie Liu drafted the manuscript. Ming Chang and Xingguo Wang critically revised the manuscript and gave the final approval.
Conflicts of interest
There are no conflicts to declare.
Acknowledgements
The National Natural Science Foundation of China (Grant No. 31972037 and 31872895), and the National First-Class Discipline Program of Food Science and Technology (JUFSTR20180202) are acknowledged. We thank Prof. J. Thomas Brenna for his linguistic assistance during the preparation of this manuscript. Dr Tao Zhang particularly desires to thank Ms Yingying Wang for her meticulous and continuous support.
References
- W. S. He, H. Zhu and Z. Y. Chen, Plant Sterols: Chemical and Enzymatic Structural Modifications and Effects on Their Cholesterol-Lowering Activity, J. Agric. Food Chem., 2018, 66, 3047–3062 CrossRef CAS PubMed.
- R. A. Moreau, L. Nyström, B. D. Whitaker, J. K. Winkler-Moser, D. J. Baer, S. K. Gebauer and K. B. Hicks, Phytosterols and their derivatives: Structural diversity, distribution, metabolism, analysis, and health-promoting uses, Prog. Lipid Res., 2018, 70, 35–61 CrossRef CAS PubMed.
- P. Jogchum, J. A. Nichols and R. P. Mensink, Plant sterols and stanols: effects on mixed micellar composition and LXR (target gene) activation, J. Lipid Res., 2005, 46, 2468–2476 CrossRef PubMed.
- S. Azadmard-Damirchi and P. C. Dutta, Novel solid-phase extraction method to separate 4-desmethyl-, 4-monomethyl-, and 4,4′-dimethylsterols in vegetable oils, J. Chromatogr. A, 2006, 1108, 183–187 CrossRef CAS PubMed.
- J. N. Valitova, A. G. Sulkarnayeva and F. V. Minibayeva, Plant sterols: Diversity, biosynthesis, and physiological functions, Biochemistry, 2016, 81, 819–834 CAS.
- J. J. A. Ferguson, E. Stojanovski, L. Macdonald-Wicks and M. L. Garg, Fat type in phytosterol products influence their cholesterol-lowering potential: A systematic review and meta-analysis of RCTs, Prog. Lipid Res., 2016, 64, 16–29 CrossRef CAS.
- J. Plat, S. Baumgartner, T. Vanmierlo, D. Lütjohann, K. L. Calkins, D. G. Burrin, G. Guthrie, C. Thijs, A. A. Te Velde, A. C. E. Vreugdenhil, R. Sverdlov, J. Garssen, K. Wouters, E. A. Trautwein, T. G. Wolfs, C. van Gorp, M. T. Mulder, N. P. Riksen, A. K. Groen and R. P. Mensink, Plant-based sterols and stanols in health & disease: “Consequences of human development in a plant-based environment?”, Prog. Lipid Res., 2019, 74, 87–102 CrossRef CAS PubMed.
- T. Vanmierlo, J. F. J. Bogie, J. Mailleux, J. Vanmol, D. Lütjohann, M. Mulder and J. J. A. Hendriks, Plant sterols: Friend or foe in CNS disorders?, Prog. Lipid Res., 2015, 58, 26–39 CrossRef CAS PubMed.
- K. M. Carvalho, T. S. de Melo, K. M. de Melo, A. L. Quinderé, F. T. de Oliveira, A. F. Viana, P. I. Nunes, J. D. Quetz, D. A. Viana and S. A. Da, Amyrins from Protium heptaphyllum Reduce High-Fat Diet-Induced Obesity in Mice via Modulation of Enzymatic, Hormonal And Inflammatory Responses, Planta Med., 2016, 83, 285–291 CrossRef PubMed.
- V. Sudhahar, S. A. Kumar, Y. Mythili and P. Varalakshmi, Remedial effect of lupeol and its ester derivative on hypercholesterolemia-induced oxidative and inflammatory stresses, Nutr. Res., 2007, 27, 778–787 CrossRef CAS.
- F. Santos, V. Rao, K. Carvalho, T. Morais, A. D. Silva and M. Chaves, The Alpha, Beta-Amyrin from Protium Heptaphyllum Exerts Antiobese-Related Effects in Mice Fed on High Fat Diet, Planta Med., 2013, 79, 14 Search PubMed.
- K. N. Sangeetha, K. Shilpa, P. J. Kumari and B. S. Lakshmi, Reversal of dexamethasone induced insulin resistance in 3T3L1 adipocytes by 3β-taraxerol of Mangifera indica, Phytomedicine, 2013, 20, 213–220 CrossRef CAS.
- K. N. Sangeetha, S. Sujatha, V. S. Muthusamy, S. Anand, N. Nithya, D. Velmurugan, A. Balakrishnan and B. S. Lakshmi, 3beta-taraxerol of Mangifera indica, a PI3K dependent dual activator of glucose transport and glycogen synthesis in 3T3-L1 adipocytes, Biochim. Biophys. Acta, Gen. Subj., 2010, 1800, 359–366 CrossRef CAS PubMed.
- V. Sudhahar, S. A. Kumar, P. Varalakshmi and V. Sujatha, Protective effect of lupeol and lupeol linoleate in hypercholesterolemia associated renal damage, Mol. Cell. Biochem., 2008, 317, 11–20 CrossRef CAS PubMed.
- World Health Organization (WHO), Deaths due to noncommunicable diseases: age-standardized death rate (per 100
000 population) – both sexes, http://gamapserver.who.int/mapLibrary/Files/Maps/Global_NCD_deaths_2015.png, accessed data: 25 April 2017.
- Food and Agriculture Organization of the United Nations (FAO), Food Balance Sheets, http://www.fao.org/faostat/en/#data/FBS, accessed date: 12 December 2017.
-
F. Shahidi, Baileys Industrial Oil and Fat Products, Volume 3, Edible Oil and Fat Products: Specialty Oils and Oil Products, John Wiley & Sons, Inc., New Jersey, part 3, 2005 Search PubMed.
- N. Maurya and B. K. Velmurugan, Therapeutic applications of cannabinoids, Chem.-Biol. Interact., 2018, 293, 77–88 CrossRef CAS PubMed.
- K. Sharma and R. Zafar, Occurrence of taraxerol and taraxasterol in medicinal plants, Pharmacogn. Rev., 2015, 9, 19–23 CrossRef CAS PubMed.
-
L. H. N. VZquez, J. Palazon and A. Navarro-OcaA, The Pentacyclic Triterpenes amyrins: A Review of Sources and Biological Activities. Phytochemicals-A Global Perspective of Their Role in Nutrition and Health, InTech., 2012 Search PubMed.
- M. Saleem, Lupeol, a novel anti-inflammatory and anti-cancer dietary triterpene, Cancer Lett., 2009, 285, 109–115 CrossRef CAS.
- S. K. Karan, K. K. Rout, S. K. Mishra and D. K. Pal, Development and Validation of HPTLC Method for Quantification of the Antidiabetic Compound alpha-Amyrin Acetate in Streblus asper Lour, JPC-J. Planar Chromatogr., 2012, 25, 554–558 CrossRef CAS.
- J. H. Lee, K. T. Lee, J. H. Yang, N. I. Baek and D. K. Kim, Acetylcholinesterase inhibitors from the twigs ofvaccinium oldhamimiquel, Arch. Pharmacal Res., 2004, 27, 53–56 CrossRef CAS.
- C. Meyresilva, C. M. Petry, T. E. Berté, R. G. Becker, F. Zanatta, F. Dellemonache, V. Cechinel-Filho and S. F. Andrade, Phytochemical analyses and gastroprotective effects of Eugenia umbelliflora (Myrtaceae) on experimental gastric ulcers, Nat. Prod. Commun., 2009, 4, 911–916 CAS.
- Q. V. Vuong, V. T. Nguyen, D. T. Thanh, D. J. Bhuyan, C. D. Goldsmith, E. Sadeqzadeh, C. J. Scarlett and M. C. Bowyer, Optimization of ultrasound-assisted extraction conditions for euphol from the medicinal plant, Euphorbia tirucalli, using response surface methodology, Ind. Crops Prod., 2015, 63, 197–202 CrossRef CAS.
- J. Han, Y. Yang and M. Feng, Analysis of phytosterol contents in Chinese plant food and primary estimation of its intake of people, J. Hyg. Res., 2007, 36, 301–305 CAS.
- L. Normén, S. Bryngelsson, M. Johnsson, P. Evheden, L. Ellegård, H. Brants, H. Andersson and P. Dutta, The Phytosterol Content of Some Cereal Foods Commonly Consumed in Sweden and in the Netherlands, J. Food Compos. Anal., 2002, 15, 693–704 CrossRef.
- N. B. S. Rao, Nonglyceride Components of Edible Oils and Fats. Chemistry and Distribution, Food Nutr. Bull., 2001, 22, 81–86 CrossRef.
- S. Azadmard-Damirchi, M. Nemati, J. Hesari, M. Ansarin and B. Fathi-Achachlouei, Rapid Separating and Enrichment of 4,4′-Dimethylsterols of Vegetable Oils by Solid-Phase Extraction, J. Am. Oil Chem. Soc., 2010, 87, 1155–1159 CrossRef CAS.
- S. Mo, L. Dong, W. J. Hurst and R. B. V. Breemen, Quantitative Analysis of Phytosterols in Edible Oils Using APCI Liquid Chromatography–Tandem Mass Spectrometry, Lipids, 2013, 48, 949–956 CrossRef CAS PubMed.
- E. J. Corey, S. P. T. Matsuda, C. H. Baker, A. Y. Ting and H. Cheng, Molecular Cloning of a Schizosaccharomyces pombe cDNA Encoding Lanosterol Synthase and Investigation of Conserved Tryptophan Residues, Biochem. Biophys. Res. Commun., 1996, 219, 327–331 CrossRef CAS.
- J. B. Herrera, B. Bartel, W. K. Wilson and S. P. Matsuda, Cloning and characterization of the Arabidopsis thaliana lupeol synthase gene, Phytochemistry, 1998, 49, 1905–1911 CrossRef CAS.
- R. J. Cenedella, Digitonide precipitable sterols: A reevaluation with special attention to lanosterol, Lipids, 1982, 17, 443–447 CrossRef CAS.
- E. M. Gaydou, J. Viano and J. L. Bourreil, Canavalia ensiformis neutral lipids, a rich source of lupeol, J. Am. Oil Chem. Soc., 1992, 69, 495–497 CrossRef CAS.
- K. E. Peers, The non-glyceride saponifiables of shea butter, J. Sci. Food Agric., 2010, 28, 1000–1009 CrossRef.
- T. Akihisa, Triacylglycerol and Triterpene Ester Composition of Shea Nuts from Seven African Countries, J. Oleo Sci., 2001, 14, 135–138 Search PubMed.
- R. S. Dodd, F. Fromard, Z. A. Rafii and F. Blasco, Biodiversity among West African Rhizophora: Foliar wax chemistry, Biochem. Syst. Ecol., 1995, 23, 859–868 CrossRef CAS.
- M. Martelanc, I. Vovk and B. Simonovska, Separation and identification of some common isomeric plant triterpenoids by thin-layer chromatography and high-performance liquid chromatography, J. Chromatogr. A, 2009, 1216, 6662–6670 CrossRef CAS PubMed.
- L. Hernández-Vázquez, S. Mangas, J. Palazón and A. Navarro-Ocaña, Valuable medicinal plants and resins: Commercial phytochemicals with bioactive properties, Ind. Crops Prod., 2010, 31, 476–480 CrossRef.
- Y. P. Yoon, H. J. Lee, D. Lee, S. K. Lee, J. Hong and C. J. Lee, Effects of Lupenone, Lupeol, and Taraxerol Derived from Adenophora triphylla on the Gene Expression and Production of Airway MUC5AC Mucin, Tuberc, Respir. Dis., 2015, 78, 210–217 Search PubMed.
- M. Huang, L. Zhang, F. Zhou, X. Ma, Z. Li, T. Zhong and Y. Zhang, A New Ursane Triterpenoid Possessing Cytotoxicity from the Fruits of Vitex trifolia var. simplicifolia, Chem. Nat. Compd., 2016, 52, 660–663 CrossRef CAS.
- M. Silva, D. Santos, G. M. Pinna, T. J. R. Câmara and A. Oliveira, Chemical composition and ultrastructure of the foliar cuticular wax of two Brazilian cultivars of castor bean (Ricinus communis L.), Ind. Crops Prod., 2016, 95, 558–563 CrossRef.
- J. A. Ramos-Hernández, M. Calderon-Santoyo, A. Navarro-Ocaña, J. C. Barros-Castillo and J. Ragazzo-Sanchez, Use of emerging technologies in the extraction of lupeol, α-amyrin and β-amyrin from sea grape (Coccoloba uvifera L.), J. Food Sci. Technol. Mys., 2018, 57, 2377–2383 CrossRef.
- J.-M. Lavoie and T. Stevanovic, Variation of Chemical Composition of the Lipophilic Extracts from Yellow Birch (Betula alleghaniensis) Foliage, J. Agric. Food Chem., 2005, 53, 4747–4756 CrossRef CAS.
- J. De la Cruz-Cañizares, M. Doménech-Carbó, J. V. Gimeno-Adelantado, R. Mateo and F. Reig, Study of Burseraceae, resins used in binding media and varnishes from artworks by gas chromatography-mass spectrometry and pyrolysis-gas chromatography-mass spectrometry, J. Chromatogr. A, 2005, 1093, 177–194 CrossRef.
- S. Bauer, E. Schulte and H.-P. Thier, Composition of the surface waxes from bell pepper and eggplant, Eur. Food Res. Technol., 2005, 220, 5–10 CrossRef CAS.
- G. Aragao, M. Pinheiro, B. P. Nogueira, T. Lemos and G. Viana, Analgesic and Anti-Inflammatory Activities of the Isomeric Mixture of Alpha- and Beta-Amyrin from Protium heptaphyllum (Aubl.) March, J. Herb. Pharmacother., 2007, 7, 31–47 CrossRef CAS.
- A. Rüdiger and V. Veiga, Chemodiversity of Ursane-and Oleanane-Type Triterpenes in Amazonian Burseraceae Oleoresins, Chem. Biodivers., 2013, 10, 1142–1153 CrossRef.
- M. Gawrońska-Grzywacz and T. Krzaczek, Identification and determination of triterpenoids in Hieracium pilosella L, J. Sep. Sci., 2007, 30, 746–750 CrossRef.
- A. Romero-Estrada, A. Maldonado-Magaña, J. González-Christen, S. M. Bahena, M. L. Garduno-Ramirez, V. Rodriguez and L. Alvarez, Anti-inflammatory and antioxidative effects of six pentacyclic triterpenes isolated from the Mexican copal resin of Bursera copallifera, BMC Complementary Altern. Med., 2016, 16, 422 CrossRef.
- G. Yang, B. R. Wiseman, D. J. Isenhour and K. E. Espelie, Chemical and ultra-structural analysis of corn cuticular lipids and their effect on feeding by fall armyworm larvae, J. Chem. Ecol., 1993, 19, 2055–2074 CrossRef CAS.
- X. Xu, J. Dong, X. Mu and L. Sun, Supercritical CO2 extraction of oil, carotenoids, squalene and sterols from lotus (Nelumbo nucifera Gaertn) bee pollen, Food Bioprod. Process., 2011, 89, 47–52 CrossRef CAS.
- A. Szakiel, C. Pączkowski and S. Huttunen, Triterpenoid Content of Berries and Leaves of Bilberry Vaccinium myrtillus from Finland and Poland, J. Agric. Food Chem., 2012, 60, 11839–11849 CrossRef CAS.
- S. Dewanjee, T. Dua and R. Sahu, Potential anti-inflammatory effect of Leea macrophylla Roxb. leaves: A wild edible plant, Food Chem. Toxicol., 2013, 59, 514–520 CrossRef CAS.
- Q. Peng, G. Li, Y. Ma, J. Huang, X. Wei and J. Wang, Chemical constituents of Euphorbia kansui, Biochem. Syst. Ecol., 2012, 43, 64–66 CrossRef CAS.
- A. Munhoz, B. R. Minozzo, L. Cruz, T. L. Oliveira, W. M. Machado, A. V. Pereira, D. Fernandes, F. Manente, J. C. Vellosa, A. Nepel, A. Barison and F. Beltrame, Chemical and Pharmacological Investigation of the Stem Bark of Synadenium grantii, Planta Med., 2014, 80, 458–464 CrossRef CAS.
- V. Anjaneyulu, K. H. Prasad, K. Ravi and J. D. Connolly, Triterpenoids from Mangifera indica, Phytochemistry, 1989, 28, 2359–2367 CrossRef.
- I. Ivanov, N. Petkova, Y. Tumbarski, I. Dincheva, I. Badjakov, P. Denev and A. Pavlov, GC-MS characterization of n-hexane soluble fraction from dandelion (Taraxacum officinale Weber ex F.H. Wigg.) aerial parts and its antioxidant and antimicrobial properties, Z. Naturforsch., C: J. Biosci., 2018, 73, 41–47 CAS.
- A. Szakiel, M. Grabarczyk, C. Pączkowski and A. Mieczkowski, Comparison of the profiles of non-glycosylated triterpenoids from leaves of plants of selected species of genus Dioscorea, Phytochem. Lett., 2017, 20, 350–355 CrossRef CAS.
- A. Ranalli, S. Contento, L. Lucera, G. Pavone, G. Di Giacomo, A. Loretta, C. Di Gregorio, A. Mucci and I. Kourtikakis, Characterization of Carrot Root Oil Arising from Supercritical Fluid Carbon Dioxide Extraction, J. Agric. Food Chem., 2004, 52, 4795–4801 CrossRef CAS.
-
A. Kamal-Eldin, Edible Oil & Fat Products: Minor Components of Fats and Oils, in Bailey's Industrial Oil and Fat Products, John Wiley & Sons, Inc., New Jersey, 2005 Search PubMed.
- D. Chrysafidis, P. Maggos, V. Kiosseoglou and D. Boskou, Composition of total and esterified 4α-monomethylsterols and triterpene alcohols in virgin olive oil, J. Sci. Food Agric., 1992, 58, 581–583 CrossRef.
- P. J. Barnes, Lipid Composition of Wheat Germ and Wheat Germ Oil, Eur. J. Lipid Sci. Technol., 1982, 84, 256–269 CAS.
- M. Farines, S. Cocallemen and J. Soulier, Triterpene alcohols, 4-methylsterols and 4-desmethylsterols of eggplant seed oil: A new phytosterol, Lipids, 1988, 23, 349–354 CrossRef CAS.
- J. Li, C.-T. Ho, H. E. Li, H. Tao and L. Tao, Separation of sterols and triterpene alcohols from unsaponifiable fractions of three plant seed oils, J. Food Lipids, 2007, 7, 11–20 CrossRef.
- T. S. C. Li, T. H. J. Beveridge and J. C. G. Drover, Phytosterol content of sea buckthorn (Hippophae rhamnoides L.) seed oil: Extraction and identification, Food Chem., 2007, 101, 1633–1639 CrossRef CAS.
- W. J. Zhou, H. L. Yang, J. Shao, J. Mei, K. K. Chang, R. Zhu and M. Q. Li, Anti-inflammatory cytokines in endometriosis, Cell. Mol. Life Sci., 2019, 76, 1–22 CrossRef.
- M. B. Gupta, T. N. Bhalla, G. P. Gupta, C. R. Mitra and K. P. Bhargava, Anti-inflammatory activity of natural products (I) triterpenoids, Eur. J. Pharmacol., 1969, 6, 67–70 CrossRef CAS.
- M. Fernandez, B. De-Las-Heras, M. Garcia, M. Saenz and A. Villar, New insights into the mechanism of action of the anti-inflammatory triterpene lupeol, J. Pharm. Pharmacol., 2010, 53, 1533–1539 CrossRef.
- A. Fernández, A. Álvarez, M. D. GarcíA and M. T. Sáenz, Anti-inflammatory effect of Pimenta racemosa var. ozua and isolation of the triterpene lupeol, Farmaco, 2001, 56, 335–338 CrossRef.
- A. Fernandez-Arche, M. T. Saenz, M. Arroyo, R. De La Puerta and M. D. Garcia, Topical anti-inflammatory effect of tirucallol, a triterpene isolated from Euphorbia lactea latex, Phytomedicine, 2010, 17, 146–148 CrossRef CAS.
- T. Akihisa, K. Yasukawa, H. Oinuma, Y. Kasahara, S. Yamanouchi, M. Takido, K. Kumaki and T. Tamura, Triterpene alcohols from the flowers of compositae and their anti-inflammatory effects, Phytochemistry, 1996, 43, 1255–1260 CrossRef CAS.
- T. Geetha and P. Varalakshmi, Anticomplement activity of triterpenes from Crataeva nurvala stem bark in adjuvant arthritis in rats, Gen. Pharmacol., 1999, 32, 495–497 CrossRef CAS.
- G. Kweifio-Okai, D. Bird, P. Eu, A. R. Carroll, R. Ambrose and B. Field, Effect of alpha-amyrin palmitate on adjuvant arthritis, Drugs Exp. Clin. Res., 1994, 20, 1–5 CAS.
- R. M. Latha, M. Lenin, M. Rasool and P. Varalakshmi, A novel derivative pentacyclic triterpene and omega 3 fatty acid,, Prostaglandins, Leukotrienes Essent. Fatty Acids, 2001, 64, 81–85 CrossRef CAS.
- T. Veena, T. Neelam, P. Tawatchai, B. Marshall, G. Marie, L. Harvinder and C. S. David, CD4 and CD8 T cells in susceptibility/protection to collagen-induced arthritis in HLA-DQ8-transgenic mice: implications for rheumatoid arthritis, J. Immunol., 2002, 168, 5867–5875 CrossRef.
- R. S. Liblau, S. M. Singer and H. O. Mcdevitt, Th1 and Th2 CD4+ T cells in the pathogenesis of organ-specific autoimmune diseases, Immunol. Today, 1995, 16, 34–38 CrossRef CAS.
- B. Sarang, K. Anpurna, K. Beenish, A. S. Fayaz, K. A. Suri, B. D. Gupta, N. K. Satti and G. N. Qazi, Suppression of T lymphocyte activity by lupeol isolated from Crataeva religiosa, Phytother. Res., 2010, 20, 279–287 Search PubMed.
- S. A. H. Pinto, L. M. S. Pinto, G. M. A. Cunha, M. H. Chaves, F. A. Santos and V. S. Rao, Anti-inflammatory effect of α, β-Amyrin, a pentacyclic triterpene from Protium heptaphyllum in rat model of acute periodontitis, Inflammopharmacology, 2008, 16, 48–52 CrossRef CAS.
- A. Thirupathi, P. C. Silveira, R. T. Nesi and R. A. Pinho, β-Amyrin, a pentacyclic triterpene, exhibits anti-fibrotic, anti-inflammatory, and anti-apoptotic effects on dimethyl nitrosamine-induced hepatic fibrosis in male rats, Hum. Exp. Toxicol., 2016, 36, 113–122 CrossRef.
- M. B. Gupta, T. N. Bhalla, K. K. Tangri and K. P. Bhargava, Biochemical study of the anti-inflammatory activity of α and β-amyrin acetate, Biochem. Pharmacol., 1971, 20, 401–405 CrossRef CAS PubMed.
- E. L. Nguemfo, T. Dimo, A. B. Dongmo, A. G. B. Azebaze, K. Alaoui, A. E. Asongalem, Y. Cherrah and P. Kamtchouing, Anti-oxidative and anti-inflammatory activities of some isolated constituents from the stem bark of Allanblackia monticola Staner L.C (Guttiferae), Inflammopharmacology, 2009, 17, 37–41 CrossRef CAS PubMed.
- J. F. Vasconcelos, M. M. Teixeira, J. M. Barbosa-Filho, A. S. Lúcio, J. R. Almeida, L. P. de Queiroz, R. Ribeiro-Dos-Santos and M. B. P. Soares, The triterpenoid lupeol attenuates allergic airway inflammation in a murine model, Int. Immunopharmacol., 2008, 8, 1216–1221 CrossRef CAS.
- C. E. Vitor, C. P. Figueiredo, D. B. Hara, A. F. Bento, T. L. Mazzuco and J. B. Calixto, Therapeutic action and underlying mechanisms of a combination of two pentacyclic triterpenes, alpha- and beta-amyrin, in a mouse model of colitis, Br. J. Pharmacol., 2010, 157, 1034–1044 CrossRef.
- R. Khanra, S. Dewanjee, T. K. Dua and N. Bhattacharjee, Taraxerol, a pentacyclic triterpene from Abroma augusta leaf, attenuates acute inflammation via inhibition of NF-κB signaling, Biomed. Pharmacother., 2017, 88, 918–923 CrossRef CAS.
- C. M. Melo, K. M. Carvalho, J. C. Neves, T. C. Morais, V. S. Rao, F. A. Santos, G. A. Brito and M. H. Chaves, alpha,beta-amyrin, a natural triterpenoid ameliorates L-arginine-induced acute pancreatitis in rats, World J. Gastroenterol., 2010, 16, 4272–4280 CrossRef CAS PubMed.
- T. Geetha and P. Maralakshmi, Effect of lupeol and lupeol linoleate on lysosomal enzymes and collagen in adjuvant-induced arthritis in rats, Mol. Cell. Biochem., 1999, 201, 83–87 CrossRef CAS PubMed.
- J. B. Nikiéma, R. Vanhaelen-Fastré, M. Vanhaelen, J. Fontaine, C. De Graef and M. Heenen, Effects of antiinflammatory triterpenes isolated from Leptadenia hastata latex on keratinocyte proliferation, Phytother. Res., 2001, 15, 131–134 CrossRef PubMed.
- O. N. Nkasi, A. D. Lotanna, O. H. Nnaemeka, I. E. Emeka, N. C. Sylvester and F. B. C. Okoye, beta-Amyrin and alpha-amyrin acetate isolated from the stem bark of Alstonia boonei display profound anti-inflammatory activity, Pharm. Biol., 2014, 52, 1478–1486 CrossRef PubMed.
- K. Yasukawa, T. Akihisa, Z. Y. Yoshida and M. Takido, Inhibitory effect of euphol, a triterpene alcohol from the roots of Euphorbia kansui, on tumour promotion by 12-O-tetradecanoylphorbol-13-acetate in two-stage carcinogenesis in mouse skin, J. Pharm. Pharmacol., 2010, 52, 119–124 CrossRef PubMed.
- C. M. Melo, T. C. Morais, A. R. Tomé, G. A. C. Brito, M. H. Chaves, V. S. Rao and F. A. Santos, Anti-inflammatory effect of α,β-amyrin, a triterpene from Protium heptaphyllum, on cerulein-induced acute pancreatitis in mice, Inflammation Res., 2011, 60, 673–681 CrossRef CAS PubMed.
- R. Medeiros, M. Otuki, M. C. Avellar and J. Calixto, Mechanisms underlying the inhibitory actions of the pentacyclic triterpene α-amyrin in the mouse skin inflammation induced by phorbol ester 12-O-tetradecanoylphorbol-13-acetate, Eur. J. Pharmacol., 2007, 559, 227–235 CrossRef CAS PubMed.
- M. F. Otuki, J. Ferreira, F. V. Lima, C. Meyresilva, A. Malheiros, L. A. Muller, G. S. Cani, A. R. Santos, R. A. Yunes and J. B. Calixto, Antinociceptive properties of mixture of alpha-amyrin and beta-amyrin triterpenes: evidence for participation of protein kinase C and protein kinase A pathways, J. Pharmacol. Exp. Ther., 2005, 313, 310–318 CrossRef CAS PubMed.
- N. K. Kasinathan, B. Subramaniya and N. Devaraj, NF-κB/twist mediated regulation of colonic inflammation by lupeol in abating dextran sodium sulfate induced colitis in mice, J. Funct. Foods, 2018, 41, 240–249 CrossRef CAS.
- Y. Zhu, X. Li, J. Chen, T. Chen, Z. Shi, M. Lei, Y. Zhang, P. Bai, Y. Li and X. Fei, The pentacyclic triterpene Lupeol switches M1 macrophages to M2 and ameliorates experimental inflammatory bowel disease, Int. Immunopharmacol., 2016, 30, 74–84 CrossRef CAS PubMed.
- H. Badshah, T. Ali, R. Shafiq-ur, A. Faiz-ul, F. Ullah, T. H. Kim and M. O. Kim, Protective Effect of Lupeol Against Lipopolysaccharide-Induced Neuroinflammation via the p38/c-Jun N-Terminal Kinase Pathway in the Adult Mouse Brain, J. Neuroimmune Pharm., 2016, 11, 48–60 CrossRef PubMed.
- F. A. Sheikh, P. Anjali, K. Kiranjeet and B. Sarang, Downregulation of pro-inflammatory cytokines by lupeol measured using cytometric bead array immunoassay, Phytother. Res., 2010, 24, 9–13 CrossRef PubMed.
- R. C. Dutra, R. F. Claudino, A. F. Bento, M. Rodrigo, E. C. Schmidt, Z. L. Bouzon, L. F. Pianowski and J. O. B. Calixto, Preventive and therapeutic euphol treatment attenuates experimental colitis in mice, PLoS One, 2011, 6, e27122 CrossRef CAS PubMed.
- R. Khanra, N. Bhattacharjee, T. K. Dua, A. Nandy, A. Saha, J. Kalita, P. Manna and S. Dewanjee, Taraxerol, a pentacyclic triterpenoid, from Abroma augusta leaf attenuates diabetic nephropathy in type 2 diabetic rats, Biomed. Pharmacother., 2017, 94, 726–741 CrossRef CAS PubMed.
- C. Ahumada, T. Sáenz, D. García, L. P. R. De, A. Fernandez and E. Martinez, The effects of a triterpene fraction isolated from Crataegus monogyna Jacq. on different acute inflammation models in rats and mice. Leucocyte migration and phospholipase A2 inhibition, J. Pharm. Pharmacol., 1997, 49, 329–331 CrossRef CAS PubMed.
- T. Akihisa, K. Yasukawa, M. Yamaura, M. Ukiya, Y. Kimura, N. Shimizu and K. Arai, Triterpene alcohol and sterol ferulates from rice bran and their anti-inflammatory effects, J. Agric. Food Chem., 2000, 48, 2313–2319 CrossRef CAS PubMed.
- G. F. Passos, R. Medeiros, R. Marcon, A. F. Nascimento, J. B. Calixto and L. F. Pianowski, The role of PKC/ERK1/2 signaling in the anti-inflammatory effect of tetracyclic triterpene euphol on TPA-induced skin inflammation in mice, Eur. J. Pharmacol., 2013, 698, 413–420 CrossRef CAS PubMed.
- M. Saleem, F. Afaq, V. M. Adhami and H. Mukhtar, Lupeol modulates NF-kappaB and PI3K/Akt pathways and inhibits skin cancer in CD-1 mice, Oncogene, 2004, 23, 5203–5214 CrossRef CAS PubMed.
- S. Sultana, A. Alam, N. Khan and S. Sharma, Inhibition of benzoyl peroxide and ultraviolet-B radiation induced oxidative stress and tumor promotion markers by cycloartenol in murine skin, Redox Rep., 2003, 8, 105–112 CrossRef CAS PubMed.
- M. Takasaki, T. Konoshima, H. Tokuda, K. Masuda, Y. Arai, K. Shiojima and H. Ageta, Anti-carcinogenic activity of Taraxacum plant. I, Biol. Pharm. Bull., 1999, 22, 606–610 CrossRef CAS PubMed.
- P. Ovadje, S. Ammar, J. A. Guerrero, J. T. Arnason and S. Pandey, Dandelion root extract affects colorectal cancer proliferation and survival through the activation of multiple death signalling pathways, Oncotarget, 2016, 7, 73080–73100 CrossRef PubMed.
- C. V. Rao, H. L. Newmark and B. S. Reddy, Chemopreventive effect of farnesol and lanosterol on colon carcinogenesis, Cancer Detect. Prev., 2002, 26, 419–425 CrossRef CAS PubMed.
- S. Prasad, N. Kalra and Y. Shukla, Hepatoprotective effects of lupeol and mango pulp extract of carcinogen induced alteration in Swiss albino mice, Mol. Nutr. Food Res., 2010, 51, 352–359 CrossRef PubMed.
- J. F. Hong, Y. F. Song, L. Zheng, Z. C. Zheng, H. J. Chen and S. S. Wang, Anticancer activity of taraxerol acetate in human glioblastoma cells and a mouse xenograft model via induction of autophagy and apoptotic cell death, cell cycle arrest and inhibition of cell migration, Mol. Med. Rep., 2016, 13, 4541–4548 CrossRef CAS PubMed.
- T. G. Hastings and M. J. Zigmond, Neurodegenerative Disease and Oxidative Stress: Insights from an Animal Model of Parkinsonism, Neurodegener. Dis., 1996, 37–46 CAS.
- C. G. Parsons, W. Danysz, A. Dekundy and I. Pulte, Memantine and Cholinesterase Inhibitors: Complementary Mechanisms in the Treatment of Alzheimer's Disease, Neurotoxic. Res., 2013, 24, 358–369 CrossRef CAS PubMed.
- T. E. Berté, A. P. Dalmagro, P. L. Zimath, A. E. Gonçalves, C. Meyre-Silva, C. Bürger, C. J. Weber, D. A. Dos Santos, V. Cechinel-Filho and M. M. de Souza, Taraxerol as a possible therapeutic agent on memory impairments and Alzheimer's disease: Effects against scopolamine and streptozotocin-induced cognitive dysfunctions, Steroids, 2018, 132, 5–11 CrossRef PubMed.
- S. Schwarz, A. Loesche, S. D. Lucas, S. Sommerwerk, I. Serbian, B. Siewert, E. Pianowski and R. Csuk, Converting maslinic acid into an effective inhibitor of acylcholinesterases, Eur. J. Med. Chem., 2015, 103, 438–445 CrossRef CAS PubMed.
- S. J. Park, Y. J. Ahn, S. R. Oh, Y. Lee, G. Kwon, H. Woo, H. E. Lee, D. S. Jang and J. H. Ryu, Amyrin attenuates scopolamine-induced cognitive impairment in mice, Biol. Pharm. Bull., 2014, 37, 1207–1213 CrossRef CAS PubMed.
- S. T. Ngo and M. S. Li, Top-leads from natural products for treatment of Alzheimer's disease: docking and molecular dynamics study, Mol. Simul., 2013, 39, 279–291 CrossRef CAS.
- D. Raju, E. T. Bin, M. M. N. Uddin, I. Ashekul and J. Md, Molecular docking of fisetin with AD associated AChE, ABAD and BACE1 proteins, Bioinformation, 2014, 10, 562–568 CrossRef PubMed.
- S. M. Hosen, M. Rubayed, R. Dash, M. Junaid, S. Mitra, M. Alam and R. Dey, Prospecting and Structural Insight into the Binding of Novel Plant-Derived Molecules of Leea indica as Inhibitors of BACE1, Curr. Pharm. Des., 2018, 24, 3972–3979 CrossRef PubMed.
- P. Koirala, H. S. Su, H. A. Jung and J. S. Choi, Comparative molecular docking studies of lupeol and lupenone isolated from Pueraria lobata that inhibits BACE1: Probable remedies for Alzheimer's disease, Asian Pac. J. Trop. Med., 2017, 10, 1117–1122 CrossRef PubMed.
- W. J. Hashmi, H. Ismail, F. Mehmood and B. Mirza, Neuroprotective, antidiabetic and antioxidant effect of Hedera nepalensis and lupeol against STZ+AlCl3 induced rats model, Daru, J. Pharm. Sci., 2018, 26, 179–190 CrossRef PubMed.
- L. Lim, V. Jackson-Lewis, L. C. Wong, G. H. Shui, A. X. H. Goh, S. Kesavapany, A. M. Jenner, M. Fivaz, S. Przedborski and M. R. Wenk, Lanosterol induces mitochondrial uncoupling and protects dopaminergic neurons from cell death in a model for Parkinson's disease, Cell Death Differ., 2012, 19, 416–427 CrossRef CAS PubMed.
- C. C. Wei, C. H. Chang and H. C. Liao, Anti-Parkinsonian effects of β-amyrin are regulated via LGG-1 involved autophagy pathway in Caenorhabditis elegans, Phytomedicine, 2017, 36, 118–125 CrossRef CAS PubMed.
- S. Giordano, V. Darley-Usmar and J. Zhang, Autophagy as an essential cellular antioxidant pathway in neurodegenerative disease, Redox Biol., 2014, 2, 82–90 CrossRef CAS PubMed.
- T. Sigmond, J. Barna, M. L. Tóth, K. Takács-Vellai, G. Pásti, A. L. Kovács and T. Vellai, Autophagy in Caenorhabditis elegans, Methods Enzymol., 2008, 451, 521–540 CAS.
- H. Braak and K. Del Tredici, Invited Article: Nervous system pathology in sporadic Parkinson disease, Neurology, 2008, 70, 1916–1925 CrossRef PubMed.
- X. Huang, H. Chen, W. C. Miller, R. B. Mailman, J. L. Woodard, P. C. Chen, X. Dong, R. W. Murrow, Y. Z. Wang and C. Poole, Lower low density lipid cholesterol levels are associated with Parkinson's Disease, Mov. Disord., 2007, 22, 377–381 CrossRef PubMed.
- F. A. Santos, Antihyperglycemic and hypolipidemic effects of α, β-amyrin, a triterpenoid mixture from Protium heptaphyllum in mice, Lipids Health Dis., 2012, 11, 98 CrossRef CAS PubMed.
- F. A. Santos, K. M. M. B. Carvalho, F. J. Batista-Lima, P. I. G. Nunes, A. F. S. C. Viana, A. A. de Carvalho Almeida da Silva, S. G. da Cruz Fonseca, M. H. Chaves, V. S. Rao, P. J. C. Magalhaes and T. S. de Brito, The triterpenoid alpha, beta-amyrin prevents the impaired aortic vascular reactivity in high-fat diet-induced obese mice, Naunyn Schmiedebergs Arch. Pharmacol., 2017, 6, 1–11 Search PubMed.
- B. Grygiel-Górniak, Peroxisome proliferator-activated receptors and their ligands: Nutritional and clinical implications-A review, Nutr. J., 2014, 13, 17–17 CrossRef PubMed.
- P. Prabhakar, K. H. Reeta, S. K. Maulik, A. K. Dinda and Y. K. Gupta, Alpha amyrin attenuates high fructose diet-induced metabolic syndrome in rats, Appl. Physiol., Nutr., Metab., 2017, 42, 23–32 CrossRef CAS PubMed.
- Ardiansyah, Y. Eri, S. Hitoshi, H. Keishi, H. Kazuyuki, O. Kousaku, G. Tomoko and K. Michio, Lupeol supplementation improves blood pressure and lipid metabolism parameters in stroke-prone spontaneously hypertensive rats, J. Agric. Chem. Soc. Jpn., 2012, 76, 183–185 CAS.
- V. Sudhahar, S. Ashokkumar and P. Varalakshmi, Effect of lupeol and lupeol linoleate on lipemic–hepatocellular aberrations in rats fed a high cholesterol diet, Mol. Nutr. Food Res., 2010, 50, 1212–1219 CrossRef PubMed.
- F. Daisuke, O. Fumiaki, H. Kohjiro, Y. Kiyotaka, O. Noriko and S. Akira, Triterpene alcohols and sterols from rice bran lower postprandial glucose-dependent insulinotropic polypeptide release and prevent diet-induced obesity in mice, J. Appl. Physiol., 2014, 117, 1337–1348 CrossRef PubMed.
- F. Okahara, J. Suzuki, K. Hashizume, N. Osaki and A. Shimotoyodome, Triterpene alcohols and sterols from rice bran reduce postprandial hyperglycemia in rodents and humans, Mol. Nutr. Food Res., 2016, 60, 1521–1531 CrossRef CAS PubMed.
- R. C. Dutra, K. A. S. Silva, A. F. Bento, R. Marcon, A. F. Paszcuk, F. C. Meotti, L. F. Pianowski and J. B. Calixto, Euphol, a tetracyclic triterpene produces antinociceptive effects in inflammatory and neuropathic pain: the involvement of cannabinoid system, Neuropharmacology, 2012, 63, 593–605 CrossRef CAS PubMed.
- R. C. Dutra, M. A. Bicca, G. C. Segat, K. A. Silva, E. M. Motta, L. F. Pianowski, R. Costa and J. B. Calixto, The antinociceptive effects of the tetracyclic triterpene euphol in inflammatory and neuropathic pain models: The potential role of PKCε, Neuroscience, 2015, 303, 126–137 CrossRef CAS PubMed.
- D. L. F. Oliveira, A. Vivian, B. F. J. Maria, R. L. Cezar, M. B. P. Soares and V. Cristiane Flora, Antinociceptive effect of lupeol: evidence for a role of cytokines inhibition, Phytother. Res., 2013, 27, 1557–1563 Search PubMed.
- S. S. Pereira, L. S. Lopes, R. B. Marques, K. A. Figueiredo, D. A. Costa, M. H. Chaves and F. R. C. Almeida, Antinociceptive effect of Zanthoxylum rhoifolium Lam. (Rutaceae) in models of acute pain in rodents, J. Ethnopharmacol., 2010, 129, 227–231 CrossRef CAS PubMed.
- S. Preetha, M. Kanniappan, E. Selvakumar, M. Nagaraj and P. Varalakshmi, Lupeol ameliorates aflatoxin B1 -induced peroxidative hepatic damage in rats, Comp. Biochem. Phys. C, 2006, 143, 333–339 CAS.
- T. Geetha and P. Varalakshmi, Anti-inflammatory activity of lupeol and lupeol linoleate in rats, J. Ethnopharmacol., 2001, 76, 77–80 CrossRef CAS PubMed.
- F. A. Oliveira, R. C. Lima-Junior, W. M. Cordeiro, G. M. Vieira-Junior, M. H. Chaves, F. R. Almeida, R. M. Silva, F. A. Santos and V. S. Rao, Pentacyclic triterpenoids, alpha,beta-amyrins, suppress the scratching behavior in a mouse model of pruritus, Pharmacol., Biochem. Behav., 2004, 78, 719–725 CrossRef CAS PubMed.
- F. A. Oliveira, C. L. Costa, M. H. Chaves, F. R. Almeida, I. J. Cavalcante, A. F. Lima, R. C. Lima Jr., R. M. Silva, A. R. Campos, F. A. Santos and V. S. Rao, Attenuation of capsaicin-induced acute and visceral nociceptive pain by alpha- and beta-amyrin, a triterpene mixture isolated from Protium heptaphyllum resin in mice, Life Sci., 2005, 77, 2942–2952 CrossRef CAS PubMed.
- J. Ferreira, A. E. O. Floriani, V. C. Filho, F. D. Monache, R. A. Yunes, J. B. Calixto and A. R. S. Santos, Antinociceptive properties of the methanolic extract and two triterpenes isolated from Epidendrum mosenii stems (Orchidaceae), Life Sci., 2000, 66, 791–802 CrossRef CAS PubMed.
- R. Asha, V. G. Devi and A. Abraham, Lupeol, a pentacyclic triterpenoid isolated from Vernonia cinerea attenuate selenite induced cataract formation in Sprague Dawley rat pups, Chem.-Biol. Interact., 2016, 245, 20–29 CrossRef CAS PubMed.
- Z. Ling, C. Xiang-Jun, Z. Jie, X. Yi-Bo, Y. Xu, H. Li-Dan, O. Hong, S. H. Patel, J. Xin and L. Danni, Lanosterol reverses protein aggregation in cataracts, Nature, 2015, 523, 607–611 CrossRef PubMed.
- R. S. Gupta, A. K. Bhatnager, Y. C. Joshi, M. C. Sharma, K. Veena and J. B. S. Kachhawa, Induction of antifertility with lupeol acetate in male albino rats, Pharmacology, 2005, 75, 57–62 CrossRef CAS PubMed.
- R. S. Gupta, A. K. Bhatnager, Y. C. Joshi, R. Sharma and A. Sharma, Suppression of Fertility in Male Albino Rats Following α-Amyrin Acetate Administration, Pharm. Biol., 2004, 42, 98–104 CrossRef CAS.
- P. N. Okusa, C. Stévigny, M. Névraumont, M. Gelbcke, A. P. Van, J. C. Braekman and P. Duez, Ferulaldehyde and lupeol as direct and indirect antimicrobial compounds from Cordia gilletii (Boraginaceae) root barks, Nat. Prod. Commun., 2014, 9, 619–622 CrossRef CAS PubMed.
- M. Sharifi-Rad, T. Roberts, K. R. Matthews, C. Bezerra, M. F. B. Morais-Braga, H. Coutinho, F. Sharopov, B. Salehi, Z. Yousaf, M. Sharifi-Rad, M. d. M. Contreras, E. Varoni, D. Verma, M. Iriti and J. Sharifi-Rad, Ethnobotany of the genus Taraxacum-Phytochemicals and antimicrobial activity, Phytother. Res., 2018, 32, 2131–2145 CrossRef CAS PubMed.
- G. F. Aragão, L. M. V. Carneiro, A. P. F. Junior, L. C. Vieira, P. N. Bandeira, T. L. G. Lemos and G. S. de B. Viana, A possible mechanism for anxiolytic and antidepressant effects of alpha- and beta-amyrin from Protium heptaphyllum (Aubl.) March, Pharmacol., Biochem. Behav., 2006, 85, 827–834 CrossRef PubMed.
- T. Akihisa, J. Ogihara, J. Kato, K. Yasukawa, M. Ukiya, S. Yamanouchi and K. Oishi, Inhibitory effects of triterpenoids and sterols on human immunodeficiency virus-1 reverse transcriptase, Lipids, 2001, 36, 507–512 CrossRef CAS PubMed.
- N. Mannowetz, M. R. Miller and P. V. Lishko, Regulation of the sperm calcium channel CatSper by endogenous steroids and plant triterpenoids, Proc. Natl. Acad. Sci. U. S. A., 2017, 114, 5743–5748 CrossRef CAS PubMed.
- D. M. Vincenzo, B. Maurizio and D. P. Luciano, The endocannabinoid system and its therapeutic exploitation, Nat. Rev. Drug Discovery, 2004, 3, 771–784 CrossRef.
- V. D. Marzo, Endocannabinoids: synthesis and degradation, Rev. Physiol., Biochem. Pharmacol., 2008, 160, 1–24 Search PubMed.
- V. Di Marzo and D. G. Deutsch, Biochemistry of the endogenous ligands of cannabinoid receptors, Neurobiol. Dis., 1998, 5, 386–404 CrossRef CAS.
- D. A. Karanian, S. L. Karim, J. A. T. Wood, J. S. Williams, L. Sonyuan, M. Alexandros and B. A. Bahr, Endocannabinoid enhancement protects against kainic acid-induced seizures and associated brain damage, J. Pharmacol. Exp. Ther., 2007, 322, 1059–1066 CrossRef CAS PubMed.
- J. R. Savinainen, S. M. Saario and J. T. Laitinen, The serine hydrolases MAGL, ABHD6 and ABHD12 as guardians of 2-arachidonoylglycerol signalling through cannabinoid receptors, Acta Physiol., 2012, 204, 267–276 CrossRef CAS PubMed.
- M. Edward and F. Paige, The case for medical marijuana in epilepsy, Epilepsia, 2014, 55, 783–786 CrossRef PubMed.
- P. Daniele, The molecular logic of endocannabinoid signalling, Nat. Rev. Neurosci., 2003, 4, 873–884 CrossRef PubMed.
- N. Vinogran, D. A. Karanian, S. K. Vadivel, J. R. Locklear, J. A. T. Wood, N. Mahmoud, P. M. P. Quizon, E. E. Graves, S. Vidyanand and M. Alexandros, Equipotent inhibition of fatty acid amide hydrolase and monoacylglycerol lipase - dual targets of the endocannabinoid system to protect against seizure pathology, Neurotherapeutics, 2012, 9, 801–813 CrossRef PubMed.
- R. G. Pertwee, A. C. Howlett, M. E. Abood, S. P. Alexander, M. V. Di, M. R. Elphick, P. J. Greasley, H. S. Hansen, G. Kunos and K. Mackie, International Union of Basic and Clinical Pharmacology. LXXIX. Cannabinoid receptors and their ligands: beyond CB1 and CB2, Pharmacol. Rev., 2010, 62, 588–631 CrossRef CAS PubMed.
- A. R. King, E. Y. Dotsey, A. Lodola, K. M. Jung, A. Ghomian, Y. Qiu, J. Fu, M. Mor and D. Piomelli, Discovery of Potent and Reversible Monoacylglycerol Lipase Inhibitors, Chem. Biol., 2009, 16, 1045–1052 CrossRef CAS PubMed.
- K. A. Silva, A. F. Paszcuk, G. F. Passos, E. S. Silva, A. F. Bento, F. C. Meotti and J. B. Calixto, Activation of cannabinoid receptors by the pentacyclic triterpene α,β-amyrin inhibits inflammatory and neuropathic persistent pain in mice, Pain, 2011, 152, 1872–1887 CrossRef PubMed.
- I. Matos, A. F. Bento, R. Marcon, R. F. Claudino and J. B. Calixto, Preventive and therapeutic oral administration of the pentacyclic triterpene α,β-amyrin ameliorates dextran sulfate sodium-induced colitis in mice: The relevance of cannabinoid system, Mol. Immunol., 2013, 54, 482–492 CrossRef CAS PubMed.
- A. Chicca, J. Marazzi and J. Gertsch, The antinociceptive triterpene β-amyrin inhibits 2-arachidonoylglycerol (2-AG) hydrolysis without directly targeting cannabinoid receptors, Br. J. Pharmacol., 2012, 167, 1596–1608 CrossRef CAS PubMed.
- M. R. Miller, N. Mannowetz, A. T. Iavarone, R. Safavi, E. O. Gracheva, J. F. Smith, R. Z. Hill, D. M. Bautista, Y. Kirichok and P. V. Lishko, Unconventional endocannabinoid signaling governs sperm activation via the sex hormone progesterone, Science, 2016, 352, 555–559 CrossRef CAS PubMed.
- Z. Merali, C. Cayer, P. Kent, R. Liu, V. Cal, C. S. Harris and J. T. Arnason, Sacred Maya incense, copal (Protium copal - Burseraceae), has antianxiety effects in animal models, J. Ethnopharmacol., 2018, 216, 63–70 CrossRef PubMed.
- D. A. Ciulla, A. G. Wagner, X. Liu, C. L. Cooper, M. T. Jorgensen, C. Wang, P. Goyal, N. K. Banavali, J. L. Pezzullo, J.-L. Giner and B. P. Callahan, Sterol A-ring plasticity in hedgehog protein cholesterolysis supports a primitive substrate selectivity mechanism, Chem. Commun., 2019, 55, 1829–1832 RSC.
- E. J. Barreiro, A. E. Kümmerle and C. A. M. Fraga, The methylation effect in medicinal chemistry, Chem. Rev., 2011, 111, 5215–5246 CrossRef CAS PubMed.
- M. Kanyonyo, S. J. Govaerts, E. Hermans, J. H. Poupaert and D. M. Lambert, 3-Alkyl-(5,5′-diphenyl) imidazolidinediones as new cannabinoid receptor ligands, Bioorg. Med. Chem. Lett., 1999, 9, 2233–2236 CrossRef CAS PubMed.
- B. Kirsten, S. Petra and K. Teymuras, Sterol structure determines the separation of phases and the curvature of the liquid-ordered phase in model membranes, Proc. Natl. Acad. Sci. U. S. A., 2005, 102, 3272–3277 CrossRef PubMed.
- K. Bloch, Sterol Structure and Membrane Function, CRC Crit. Rev. Biochem., 1981, 14, 47–92 CrossRef PubMed.
- B. de Kruyff, R. A. Demel and L. L. M. dan Deenen, The effect of cholesterol and epicholesterol incorporation on the permeability and on the phase transition of intact Acholeplasma laidlawii cell membranes and derived liposomes, Biochim. Biophys. Acta, 1972, 255, 331–347 CrossRef CAS.
- M. Nishio, Influence of Basicity and Steric Hindrance on the Reactivity of Amines toward Tetrachloroaurate(III) Ion, Inorg. Chem., 1966, 5, 1674–1677 CrossRef.
- K. H. Choi, H. J. Lee, A. Karpfen, C. J. Yoon, J. Park and Y. S. Choi, Hydrogen-bonding interaction of methyl-substituted pyridines with thioacetamide: steric hindrance of methyl group, Chem. Phys. Lett., 2001, 345, 338–344 CrossRef CAS.
- K. Brejc, T. K. Sixma, P. A. Kitts, S. R. Kain, R. Y. Tsien, M. Ormö and S. J. Remington, Structural basis for dual excitation and photoisomerization of the Aequorea victoria green fluorescent protein, Proc. Natl. Acad. Sci. U. S. A., 1997, 94, 2306–2311 CrossRef CAS PubMed.
- F. Gelmini, M. Ruscica, C. Macchi, V. Bianchi, F. R. Maffei, G. Beretta and P. Magni, Unsaponifiable Fraction of Unripe Fruits of Olea europaea: An Interesting Source of Anti-inflammatory Constituents, Planta Med., 2015, 82, 273–278 CrossRef PubMed.
- H. Constance, L. Melanie, S. Armin, E. Reinhard and C. M. Schempp, Treatment of actinic keratoses with birch bark extract: a pilot study, J. Dtsch. Dermatol. Ges., 2010, 4, 132–136 Search PubMed.
- Y. A. M. Yusof, Gingerol and Its Role in Chronic Diseases, Adv. Exp. Med. Biol., 2016, 929, 145–175 CrossRef PubMed.
- K. Ogihara, Y. Naya, Y. Okamoto and K. Hata, Differentiation-inducing and anti-proliferative activities of lupeol on canine melanoma cells, SpringerPlus, 2014, 3, 632 CrossRef PubMed.
- F. D. Porter, Malformation syndromes due to inborn errors of cholesterol synthesis, J. Clin. Invest., 2002, 110, 715–724 CrossRef CAS PubMed.
- D. Haas and R. I. Kelley, Inborn Errors of Cholesterol Biosynthesis, Annu. Rev. Genomics Hum. Genet., 2003, 2, 299–341 Search PubMed.
- X. Fang, S. D. Rychnovsky, J. D. Belani, H. H. Hobbs, J. C. Cohen and R. B. Rawson, Dual roles for cholesterol in mammalian cells, Proc. Natl. Acad. Sci. U. S. A., 2005, 102, 14551–14556 CrossRef PubMed.
- M. A. Ruiz-Rodríguez, A. Vedani, A. L. Flores-Mireles, M. H. Chairez-Ramirez, J. A. Gallegos-Infante and R. F. Gonzalez-Laredo, In silico prediction of the toxic potential of lupeol, Chem. Res. Toxicol., 2017, 30, 1562–1571 Search PubMed.
- Y. Yamauchi, S. Yokoyama and T. Y. Chang, ABCA1-dependent sterol release: sterol molecule specificity and potential membrane domain for HDL biogenesis, J. Lipid Res., 2015, 57, 77–88 CrossRef PubMed.
|
This journal is © The Royal Society of Chemistry 2020 |