Integration of aerobic granulation and UV/H2O2 processes in a continuous flow system for the degradation of sulfolane in contaminated water†
Received
26th November 2019
, Accepted 3rd March 2020
First published on 6th May 2020
Abstract
Sulfolane contamination has increasingly become a major environmental concern around the world with emerging reports of groundwater, drinking water wells and soil contamination. In this study, a novel approach of integrating aerobic granulation technology with UV/H2O2 process in a continuous flow-through operation sequence was investigated to remediate sulfolane contaminated waters. The new hybrid technology was able to eliminate more than 99.99% of sulfolane in less than 6.3 h of combined retention time. The degradation kinetics of sulfolane were evaluated in batch and continuous flow operation which showed zero and first order for aerobic granulation and UV/H2O2 processes, respectively. In addition, the flow-through system was able to generate and maintain a healthy aerobic granular system characterized by a stable MLVSS/MLSS ratio as well as elemental and bacterial community compositions within the granules. H2O2 concentration was a crucial element for sulfolane degradation in UV/H2O2 process and various key factors were also identified that govern residual H2O2 concentration in UV/H2O2 effluents.
Water impact
Conventional treatments have proved inefficient in removing emerging contaminants from our water resources. This study demonstrated a novel technology to degrade emerging contaminants, such as sulfolane, by integrating aerobic granulation with UV/H2O2 and provides in-depth knowledge for future field applications. Offering a smaller footprint, this new technology is more economical and efficient in eliminating a wide range of water contaminants.
|
1. Introduction
With industrial advancements, various anthropogenic chemicals are being engineered everyday throughout the world. Many of these chemicals are intended for specific industrial purposes and some of them are designed to be persistent. However, problems arise when these new chemicals that are not regulated for environmental impact are released into our water bodies. One such industrial chemical, sulfolane (C4H8O2S), which is commonly used in oil and natural gas refineries was reported to cause contamination of groundwaters in many places including Alberta,1 Brisbane,2 and hundreds of drinking water wells in interior Alaska.3 Sulfolane is an organo-sulfur compound having high miscibility in water combined with low affinity for aquifer materials.4 These properties make sulfolane a good candidate for offsite migration and a persistent groundwater contaminant, once released. Toxicological data on sulfolane is limited to a few studies on exposure to rats and guinea pigs5 while no chronic effects have been reported on humans yet.6
Over the years, various biological and advanced oxidation process (AOP) treatment studies have been conducted in response to the remediation of sulfolane impacted soil and groundwaters. Some of the early work on the biodegradation of sulfolane was conducted by Greene et al. who reported that biodegradation of sulfolane was enhanced when mixed bacterial cultures were utilized including indigenous bacteria from contaminated sites in the presence of abundant nutrients.7–9 Similarly, an intensive study conducted on subarctic aquifers in Alaska by Kasanke and Leigh, also found aerobic biodegradation to prevail over anaerobic.3 Lately aerobic granulation has emerged as a promising technology compared to conventional biological processes.10 Consequently, in our previous study coaggregation of indigenous microbes from sulfolane contaminated site with aerobic granules generated stable robust granules that were able to degrade sulfolane effectively.11 This technology is ideal for application because it benefits from having a consortium of millions of microorganisms harboring within a single granule with the potential to treat the most recalcitrant contaminants present in wastewaters.12 On the other hand, one of the first in-depth AOP studies on treatment of sulfolane in aqueous medium was conducted by Yu et al., who reported the combination of UVC/H2O2, UVC/O3/H2O2 and UVC/O3 to have significant effect on sulfolane degradation.13 Similarly, UVC/O3/H2O2, calcium peroxide/ozone and calcium oxide/ozone have also shown to effectively degrade and mineralize 100 mg L−1 of sulfolane to carbon dioxide, water and sulfate in around 4 h.14,15 Izadifard et al., evaluated persulfate as another water-soluble oxidant under UVC irradiation and noticed that bubbling ozone in the presence of persulfate significantly reduced reaction time for sulfolane degradation.16 It is understandable that the above mentioned AOPs are effective in the degradation of sulfolane, however, higher contaminant concentrations seem to prolong the reaction time for complete elimination which could eventually end up as an expensive treatment.
Both biological treatment and AOPs are effective options for degrading sulfolane in contaminated waters. However, sequential treatment of these two technologies may prove to be more cost effective. Biological treatments require longer retention times and may not meet the very low discharge limits for some recalcitrant micropollutants whereas AOPs can be expensive when contaminant concentrations are high.17 It is reasonable to argue that an integration of these two technologies can be a way forward for a more cost-effective option. Only few studies have highlighted the significance of integrating a biological process with downstream AOP in which some degree of disinfection can also be achieved along with contaminant removal.18,19 Of the various AOPs available, UV/H2O2 holds an attractive edge over other processes. For example, treatment of wastewaters by ozone-based AOPs have the potential of forming hazardous by-products as well as bromate in the treated water which is considered to be a human carcinogen.20,21 Likewise, Fenton-based AOPs require the disposal of iron sludge and produces high concentration of anions in the treated water.22 In comparison to Fenton-based AOP, Giannakis et al., reported UV/H2O2 as a more efficient option in combination with biological treatment to achieve the highest elimination of micropollutants.23 Similarly, in our previous study an integration of activated sludge with UV/H2O2 achieved significant sulfolane degradation and disinfection simultaneously.19 However, it is perceived that aerobic granulation might prevail over activated sludge technology in the future and an investigation of integrating aerobic granulation with AOP is needed.
This study reports for the first time a sequential integration of aerobic granulation with UV/H2O2 in a continuous flow system to study the synergistic effect of these technologies in removing sulfolane from contaminated waters. To the best of our knowledge this is a novel approach for sulfolane treatment and has never been investigated before. Aerobic granulation has already been shown to be more effective than conventional activated sludge and UV/H2O2 benefits outweigh other AOPs. This study evaluated the performance of the integrated technology and characterized the aerobic granules during continuous operation mode including the granule community. It is reported that residual H2O2 from AOPs has the potential to cause negative effects to the ecosystem of the receiving waters.24 Furthermore, it can react with chlorine, if chlorine is used as disinfectant in downstream process, compromising disinfection before distribution and increasing costs.25 Therefore, management of H2O2 residual was also investigated in order to establish a more efficient and effective integrated technology for potential field application of sulfolane groundwater remediations.
2. Materials and methods
2.1 Chemicals
Sulfolane with 99% purity, hydrogen peroxide (H2O2, A.R. grade, ≥30%), ammonium chloride (NH4Cl, 99.99%), potassium phosphate dibasic (K2HPO4, ≥98%), potassium phosphate monobasic (KH2PO4, 99.99%), sodium acetate anhydrous (>99%), dichloromethane (DCM), and titanium(IV) oxysulfate solution (1.9–2.1%) for H2O2 determination were purchased from Sigma Aldrich, Oakville, Canada. Deionized water was used for all analytical procedures.
2.2 Experimental setup
2.2.1 Aerobic granules and UV/H2O2 system.
Aerobic granules used in this study were cultivated as described in our previous work.11 A schematic of the continuous operation of integrated aerobic granulation and UV/H2O2 processes is presented in Fig. 1. The treatment of wastewater by aerobic granulation was conducted in a 12 liter column reactor with 11 liter working volume. Fine air bubbles at 1–3 mm in size were produced via polyethylene air diffusers (Model: AS4, pore size: 140 μm, pentair Aquatic Eco-Systems, Inc., British Columbia, Canada). The diffusers were installed at the bottom of the granule reactor and air was supplied continuously through two Fluval® Q2 air pumps (Hagen Inc., Montréal, Canada) with a combined flow rate of 480 L h−1. The granule column reactor was fitted with a mesh (size 60) at the top to contain the granules within the reactor during continuous operation. Synthetic wastewater was prepared using tap water with a biochemical oxygen demand (BOD) representing that of typical municipal wastewater treatment plants in the range of 190–350 mg L−1.26 The water quality parameters of the synthetic wastewater are presented in Table 1. The composition of the wastewater was as follows (concentrations, mg L−1): sodium acetate 450, sulfolane 20, NH4Cl 28, K2HPO4 4.11, KH2PO4 3.21, and microelement solution of 1 mL L−1 giving a chemical oxygen demand (COD) concentration in the range of 431 ± 2 mg L−1. The microelement solution composition was as described previously.19
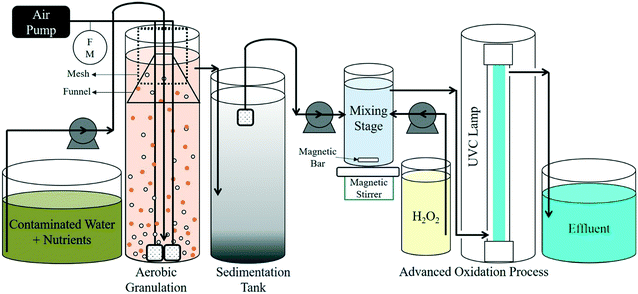 |
| Fig. 1 Schematic of a continuous flow integrated aerobic granulation and UV/H2O2 setup. | |
Table 1 Water quality parameters for the synthetic wastewater
Parameter |
Units |
Value |
pH |
— |
7.7 ± 0.3 |
COD |
mg L−1 |
431 ± 2 |
Total alkalinity |
mg L−1 (CaCO3) |
118 ± 21 |
Bicarbonate |
mg L−1 (CaCO3) |
0.033 ± 0.01 |
Calcium |
mg L−1 |
40 ± 10 |
Total dissolved solids |
mg L−1 |
194 ± 23 |
Conductivity |
μS cm−1 |
339 ± 42 |
Hardness |
mg L−1 (CaCO3) |
168 ± 32 |
Sulphate |
mg L−1 |
51 ± 8 |
The UV/H2O2 process was carried out using an annular vertical flow-through photoreactor with a low pressure UVC mercury lamp installed in the center. The 254 nm UVC lamp was placed inside a quartz sleeve with an outer diameter of 23.8 mm. The contaminated water flowed from the bottom of the photoreactor to the top (36 cm), filling the space between the quartz sleeve and the outer glass layer of the reactor (diameter of 44.5 mm) with a volume of approximately 300 mL. The radiation intensity by the UVC lamp entering the reaction vessel was determined to be 1.17 × 1018 ± 0.07 photons per s using ferrioxalate actinometry.27
2.2.2 Continuous flow experiments.
Wastewater was pumped using a peristaltic pump (Model: BT100S, Head: YZ15, Golander Pump, USA) to the bottom of aerobic granulation reactor at flow rates of 30 ± 1 and 15 ± 0.5 mL min−1 giving an organic loading rate (OLR) of 1.69 ± 0.6 and 0.85 ± 0.02 kg COD m−3 d−1, respectively. The biotreated wastewater was discharged from the top port of the bioreactor into a sedimentation tank at below mid-level. The sedimentation tank allowed the settling of any biomass that crossed over from the bioreactor. The post-bio treated water was driven into a mixing tank where 100 mg L−1 of H2O2 at 0.3 ± 0.02 mL min−1 was continuously pumped and mixed with the aid of a magnetic stirrer. A diffuser was installed at the water intake point in the sedimentation tank to allow filtration. Water in the mixing tank moved from the top port to the bottom of the UV reactor and travelled upwards to be discharged from the top port. The reactor was operated in continuous flow mode for five days and samples were collected at specific intervals. On the fifth day, the continuous flow was discontinued, and the aeration was stopped for reactor cleanup in an anaerobic state. After cleanup, the reactor was aerated for the next two days in batch mode to induce famine period. Subsequently, this process was repeated weekly.
2.2.3 Batch experiments.
Batch experiments were conducted separately to compare the degradation kinetics with the continuous flow system. For aerobic granulation, 10 liters of synthetic wastewater was prepared as described in section 2.2.1 and introduced into the granular reactor as shown in Fig. 1. Samples were withdrawn at specific time intervals, filtered using 0.45 μm filters and analyzed for sulfolane. Batch experiments for UV/H2O2 were conducted using aerobic granule treated water. Approximately 2 liters of biotreated water was collected in a beaker and pumped at 30 mL min−1 into the annular vertical flow-through photoreactor as shown in Fig. 1. A diffuser was installed at the water intake point to allow filtration. The outlet from the reactor was redirected back into the beaker and the water in the beaker was continuously stirrer using a magnetic stirrer to have a homogenous solution. Initially, the biotreated water used for UV/H2O2 batch experiment was spiked with 0.5 mg L−1 of sulfolane to mimic the same conditions that were observed during the continuous flow. Samples were collected from the beaker at specific time intervals, filtered using 0.45 μm filters and analyzed for sulfolane.
2.3 H2O2 residual test
High concentrations of residual H2O2 can have negative impacts on the environment. Therefore, evaluation of residual H2O2 concentrations, post UV/H2O2 were conducted using the above-mentioned flow-through photoreactor. A series of experiments were conducted with varying flow rates, sulfolane and H2O2 concentrations, and water matrix as shown in Table 2. Samples were collected at specified time intervals, filtered using 0.45 μm filters before sulfolane and H2O2 analysis. For biotreated water, a diffuser was installed at the influent to allow filtration of the water before entering the photoreactor.
Table 2 Experimental design to evaluate the impact of various parameters on residual H2O2 concentration
Sample I.D. |
Water matrix |
Flow rate (mL min−1) |
Sulfolane (mg L−1) |
H2O2 (mg L−1) |
D.I. = deionized water spiked with sulfolane. B.W. = biotreated synthetic wastewater.
|
F1 |
D.I. |
120 |
0.5 |
100 |
F2 |
D.I. |
60 |
0.5 |
100 |
F3 |
D.I. |
30 |
0.5 |
100 |
F4 |
D.I. |
10 |
0.5 |
100 |
S1 |
D.I. |
30 |
5 |
100 |
S2 |
D.I. |
30 |
2 |
100 |
S3 |
D.I. |
30 |
0.5 |
100 |
H1 |
D.I. |
10 |
0.5 |
100 |
H2 |
D.I. |
10 |
0.5 |
30 |
H3 |
D.I. |
10 |
0.5 |
10 |
W1 |
B.W. |
30 |
0.5 |
100 |
W2 |
B.W. |
10 |
0.5 |
100 |
2.4 Analytical methods
Turbidity measurements were conducted using 2100Q HACH Turbidimeter and UV Transmittance at 254 nm (UVT254) was measured using DR/4000 UV-vis spectrophotometer, HACH. H2O2 concentration in water was determined according to DIN 38409 H15.28 The concentrations of mixed liquor suspended solids (MLSS) and mixed liquor volatile suspended solids (MLVSS) were measured using Standard Methods.29 Sulfolane concentration was analyzed using gas chromatography flame ionization detector (GC-FID) and mass spectrometry (GC-MS) as described previously.19 Surface images, elemental characterization were obtained using scanning electron microscopy (SEM) whereas granule microbial community were analyzed through 16S rRNA gene extraction using DNeasy® PowerSoil® kit as described previously.30
3. Results and discussion
3.1 Evaluation of continuous flow integrated technology
A sequential integration of aerobic granulation and UV/H2O2 processes was investigated to treat sulfolane contaminated water in a continuous flow setup. Pre-grown aerobic granules were harvested from a sequencing batch reactor and were inoculated in a continuous flow bioreactor. The system was initially operated over two weeks to reach steady state before experimental studies. At 30 mL min−1, corresponding to a residence time of ∼6.3 h, the integrated technology had significant improvement over the three weeks of continuous operation. As shown in Fig. 2, sulfolane degradation after aerobic granulation treatment had increased by more than one log over the span of three weeks leading to an improvement in the overall sulfolane degradation (>99%). However, the sulfolane residual in the effluent was still above the guideline value of 90 μg L−1.31 When the flow rate was decreased to 15 mL min−1, sulfolane (20 mg L−1) degradation by aerobic granules was observed to be greater than 4.0
log in the bioreactor as sulfolane effluent concentration were below detection limit of 10 μg L−1. This was due to the slow flow rate that allowed granules enough time to degrade the contaminant. However, longer retention time require larger space and potentially increase operating costs associated with biological treatment. It is understandable to have a faster optimum flow rate where majority of the contaminant can be removed by the biological process, thereby, leaving AOP as a polishing step and disinfecting purposes. AOP as post treatment to biological processes almost certainly guarantees a complete mineralization of the organics in the wastewater.32 It should be noted in a continuous flow reactor, limited availability of substrate (low sulfolane concentration and other carbon source) can result in outgrowth of loose flocculent bacteria and ultimate collapse of granule.33 Therefore, efficient functioning of aerobic granulation system is dependent on higher substrate concentration. The reverse is true for AOP, where higher concentrations can make the entire system inefficient with incomplete removal due to oxidant limitations.17
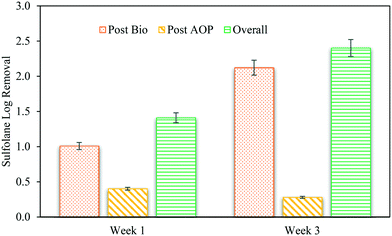 |
| Fig. 2 Evaluation of integrating aerobic granulation and UV/H2O2 in a continuous flow mode (30 mL min−1) for sulfolane degradation. | |
In a previous study, a batch mode integration of activated sludge with UV/H2O2 was able to achieve 2.8
log degradation of sulfolane (∼28 mg L−1 sulfolane concentration) effectively in less than 24 h.19 Although the initial concentration used in that study was much higher, nevertheless, ∼28 mg L−1 of sulfolane was degraded by sequential integration of 20 h activated sludge and 30 min UV/H2O2. In comparison, in this study an integration of aerobic granulation (6 h) and UV/H2O2 (10 min) in a continuous flow-through operation was able to achieve ∼2.5
log degradation (20 mg L−1 sulfolane concentration). This illustrates the potential and efficacy of aerobic granulation over activated sludge technology as a pretreatment for sulfolane degradation. It should be noted that Khan et al., used sulfolane as the sole carbon source for the activated sludge treatment19 whereas, a combination of acetate and sulfolane was provided as the substrate for aerobic granulation. Here the activated sludge biomass comprised of indigenous microbes from the sulfolane contaminated site whereas the aerobic granules were cultivated by coaggregating two different bacterial communities including the indigenous microbes.11 This has been reported by Greene et al., as well where the inoculation of mixed cultures provided a better sulfolane degradation compared to single isolates.8
The continuous flow operation of aerobic granulation was effective in removing more than 97% sulfolane and was consistent throughout the duration of the experiment as shown in Fig. 3a. Previous continuous flow aerobic granular reactor studies have also reported more than 90% COD removal efficiencies.34,35 The efficacy of the aerobic granular reactor is largely due to the dense granular biomass making it more efficient than the activated sludge process. A sedimentation tank was kept inline after the bioreactor and before the photoreactor. The purpose of the tank was to allow settling of any new biomass that might escape out of the mesh at the top of the bioreactor, thereby, avoiding the presence of biomass in the photoreactor. Fig. 3c shows the turbidity level overtime near the outlet of the bioreactor and the top of the sedimentation tank. It was observed that after 24 h, there was significant change in the effluent turbidity level of the bioreactor from new biomass growth. However, as seen from Fig. 3c, the turbidity level of the sedimentation tank at the top was consistently below 20 NTU throughout the duration of the experiment. There was accumulation of biomass at the bottom of the sedimentation tank as shown in Fig. S1† indicating the significance of the tank. Subsequently, in Fig. 3b the continuous flow-through UV/H2O2 process was also consistently effective in removing ∼50% sulfolane with 10 min of reaction time. Both Fig. 3a and b show data after the reactors reaching steady state.
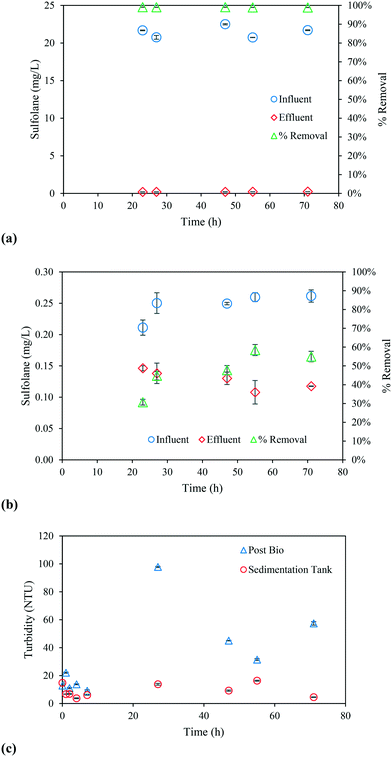 |
| Fig. 3 Degradation of sulfolane in a continuous flow reactor by (a) aerobic granulation, (b) UV/H2O2 and (c) changes in turbidity overtime in sedimentation tank and post-bio (before outlet of bioreactor). | |
The degradation kinetics of sulfolane was also compared between batch and continuous mode as shown in Table 3. It was observed that aerobic granulation strictly follows zero order kinetics irrespective of reactor mode. However, the rate constant was approximately 79% faster in continuous mode. This shows that application of aerobic granulation for the degradation of sulfolane is more efficient when applied in continuous flow reactor. Previous studies have also shown aerobic granulation to follow zero order kinetics for contaminant degradation.11,36 However, it is understood that the zero-order kinetics for biodegradation holds true for high concentration of contaminants. As the concentration depletes to a lower range, the zero-order kinetics no longer becomes valid due to substrate limitation for biomass (when sulfolane is the only carbon source) and the kinetics switch to first order. This characteristic relates to Michaelis–Menten kinetics which states that at low substrate concentrations, reaction rates are linearly proportional to substrate concentration, thus following first order kinetics. However, at high substrate concentration the rates are independent of substrate concentration, thereby, a zero order kinetics prevails.37 UV/H2O2 process followed first order kinetics for sulfolane degradation as shown in Table 3. Different from the granular reactor, the continuous mode yielded similar degradation kinetics as batch conditions in UV/H2O2 reactor which clearly demonstrates that the kinetics obtained from batch study can be used to predict the performance of continuous flow-through reactor for sulfolane treatment. In a previous study, Khan et al., had also reported a first order kinetics for sulfolane degradation by UV/H2O2 in batch study for activated sludge treated wastewater.19
Table 3 Comparison of rate order and rate constant for batch and continuous mode
Operation mode |
Technology |
Rate order |
Rate constant |
Note that the rate constants are calculated from data presented in Fig. S2–S5 in ESI.†
|
Batch |
Aerobic granulation |
Zero order |
0.0125 ± 0.002 (mg L−1 min−1) |
UV/H2O2 |
First order |
0.0529 ± 0.001 (1/min) |
Continuous |
Aerobic granulation |
Zero order |
0.0592 ± 0.002 (mg L−1 min−1) |
UV/H2O2 |
First order |
0.0566 ± 0.009 (1/min) |
3.2 Management of hydrogen peroxide in a continuous flow integrated treatment system
The presence of H2O2 in treated effluent waters can be harmful to the ecosystem of the receiving waters. The hydroxyl radicals produced from H2O2 can damage cells of microorganisms in rivers, lakes or ponds by oxidation of cellular proteins and DNA.63,64 Previous studies have shown around 50 mg L−1 of H2O2 concentrations can affect zooplanktons and phytoplankton in lakes.65 Therefore, it is a necessity to consider the discharge of residual H2O2 post UV/H2O2 treatment of contaminated waters. A series of experiments were conducted to evaluate the fate of residual H2O2 based on flow rates, water matrix, initial sulfolane and H2O2 concentrations and the results are shown in Table 4. All experiments were conducted with initial sulfolane and H2O2 concentrations of 0.5 and 100 mg L−1, respectively, unless otherwise stated. During the integrated technology experiments, more than 96% of the initial sulfolane concentration had been degraded by the aerobic granulation process leaving behind about 0.5 mg L−1 of sulfolane for the UV/H2O2 process at 30 mL min−1. Therefore, to replicate the same scenario, 0.5 mg L−1 of sulfolane concentration was selected to study the management of H2O2 in the UV/H2O2 process as part of the integrated technology.
Table 4 Sulfolane degradation and H2O2 residual under different experimental conditions
Variables |
Sample I.D. |
Sulfolane degradation (%) |
H2O2 residual (mg L−1) |
B.W. = biotreated synthetic wastewater. All experiments were conducted using deionized water, except sample W1 (30 ± 1 mL min−1) and W2 (10 ± 0.5 mL min−1).
|
Flow rate (mL min−1) |
120 ± 1 |
F1 |
86 ± 1 |
74.7 ± 2.0 |
60 ± 1 |
F2 |
96 ± 1 |
55.4 ± 1.2 |
30 ± 1 |
F3 |
100 ± 0 |
30.6 ± 1.8 |
10 ± 0.5 |
F4 |
100 ± 0 |
12.2 ± 0.9 |
Sulfolane (mg L−1) |
5 ± 0.3 |
S1 |
96 ± 1 |
38.5 ± 1.1 |
2 ± 0.1 |
S2 |
100 ± 0 |
35.5 ± 0.8 |
0.5 ± 0.01 |
S3 |
100 ± 0 |
30.6 ± 0.9 |
H2O2 (mg L−1) |
100 ± 0.6 |
H1 |
100 ± 0 |
12.8 ± 0.4 |
30 ± 0.5 |
H2 |
100 ± 0 |
5.0 ± 0.5 |
10 ± 0.3 |
H3 |
100 ± 0 |
2.0 ± 0.2 |
Water matrix |
B.W. |
W1 |
57 ± 8 |
9.7 ± 0.9 |
B.W. |
W2 |
65 ± 8 |
3.5 ± 0.7 |
It was observed that faster flow rates had higher H2O2 residual in the effluents. This was due to less retention time in the UV reactor for H2O2 cleavage by photons. Consequently, the degradation of sulfolane was also incomplete at flow rates of 60 and 120 mL min−1. At 30 mL min−1, 100% sulfolane was removed leaving behind approximately 30 mg L−1 of residual H2O2. The least amount of residual H2O2 in effluents was found at 10 mL min−1. Varying the concentrations of H2O2 at 10 mL min−1 showed that 100% sulfolane could be eliminated even with 10 mg L−1 of H2O2 leaving a residual of 2% in a clean water matrix. Interestingly, increasing sulfolane concentrations gave unexpected results. The decomposition of H2O2 in water is determined by the light intensity/wavelength, the presence of reductants and catalysts such as ferric iron. In our previous findings, Yu et al., showed that sulfolane in aqueous solution did not react with hydroperoxide but its degradation byproduct or itself might compete with H2O2 in absorbing photons, thus reducing the decomposition of H2O2.13
The impact of water matrix was quite significant when experiments were conducted with biotreated water as shown in Table 4 for samples W1 and W2. More than 90 and 96% of H2O2 had been consumed at 30 and 10 mL min−1, respectively. Although the residual concentration was much lower compared to the experiments with deionized water, nevertheless, the degradation of sulfolane in the effluent was not efficient. More than 35% sulfolane was detected in the effluent at 10 mL min−1 and even higher at 30 mL min−1. This is because majority of H2O2 was being consumed via oxidation reaction with microbial intracellular constituents such as DNA, proteins and lipids in the biotreated water.66 Even though 10 mL min−1 (sample W2) showed more than 64% sulfolane degradation with less than 4% H2O2 residual, it does not conclude that this flow rate is ideal in managing H2O2 concentration in a UV/H2O2 process effluents. Degradation of sulfolane in the contaminated waters is also a priority along with managing minimal H2O2 discharge concentrations. It is understood that H2O2 continues to get consumed in biotreated water even without exposure to UV/H2O2 process. As shown in Table S1,† there was immediate reduction of H2O2 upon injection into biotreated water as observed concentrations were lower than theoretical concentrations. The biotreated water had an indirect correlation with H2O2 observed concentrations. The observed values were much closer to the theoretical values as the percentage of biotreated water reduced. Furthermore, the applied H2O2 dose had been completely consumed by the biotreated water after 24 h of injection indicating that H2O2 continuous to deplete with time in a biotreated water. It should be noted in Table S1† that the sample with clean water had the same concentration of H2O2 even after 24 h. Therefore, it is the quality of the biotreated water that defines the residual H2O2 concentration. A lower flow rate might not be ideal for sulfolane treatment by UV/H2O2 process as most of the peroxide will be consumed for disinfection much faster than for contaminant degradation. A flow rate of 30 mL min−1 can be used, however, conditions should be suitable for the applied H2O2 dosage depending on the quality of the biotreated water. By optimizing the applied dose of H2O2, a dual function of degrading sulfolane and performing disinfection can be achieved.
3.3 Characterization of aerobic granules during continuous flow operation
A healthy granular system can be predicted from the amount of biomass contained within the granule. MLVSS analysis in Fig. 4a show the concentration of organic proportion of aerobic granules increased by ∼60% over the course of 30 d. Furthermore, Fig. 4b shows the physical appearance of granule quantity which increased significantly in the continuous flow reactor. This indicates that the design and operation of the continuous flow reactor in this study enhanced the growth of granules. A similar concentration of MLVSS was observed by Zou et al., in a continuous flow reactor with a side stream sedimentation tank and kept stable for more than 80 d.38 The ratio of MLVSS/MLSS in aerobic granules was observed to increase by 30% in the continuous flow reactor and was stable at around 0.7 from day 20 to 30 (Fig. S6†) which further illustrates a healthy granular sludge system. Chen et al.,39 operated a continuous flow membrane bioreactor and reported a similar ratio that kept constant in the range of 0.7–0.75 from day 30 to 120.
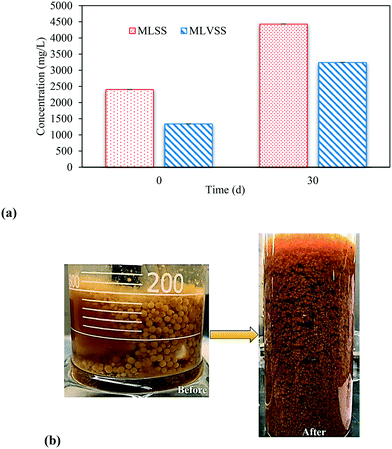 |
| Fig. 4 Transformative changes in aerobic granules during continuous flow operation, a) MLVSS concentration, and b) quantity of granules (before = day 0, after = day 30). | |
The increase in MLSS concentration (Fig. 4a) can be an indication of inorganic element accumulation within the granule. Fig. 5a shows elemental composition map on the granule surface with Fig. 5b–e showing energy dispersive X-ray spectroscopy (EDX) analysis of the notable elements such as calcium (Ca2+), phosphorus (P), potassium (K+) and iron (Fe). Other elements were also observed such as sodium (Na2+), carbon (C), oxygen (O) with low peaks of chloride (Cl−) and sulphur (S) as well. Gold (Au) and osmium (Os) peaks also appeared as they were used as coating reagents during SEM-EDX analysis. Ca2+, Fe3+, and K+ both had two peaks which indicates a higher proportion of precipitates on the granule. These divalent and multivalent elements are predominantly linked with extracellular polymetric substance (EPS) secretion in bacteria that governs the structural stability of granules. Ca2+ is reported to form the structural backbone of aerobic granules and can bind with other proteins to allow adherence of bacteria to surfaces.40 Similarly, Fe3+ is also linked to EPS binding as well as in bio-coagulation allowing other organic and inorganic molecules to aggregate giving granules a compact structure and increased settleability.41 It is noted that although Ca2+ was not added in the synthetic wastewater, but approximately 40 mg L−1 of Ca2+ was present in the local tap water used to prepare the synthetic feed.
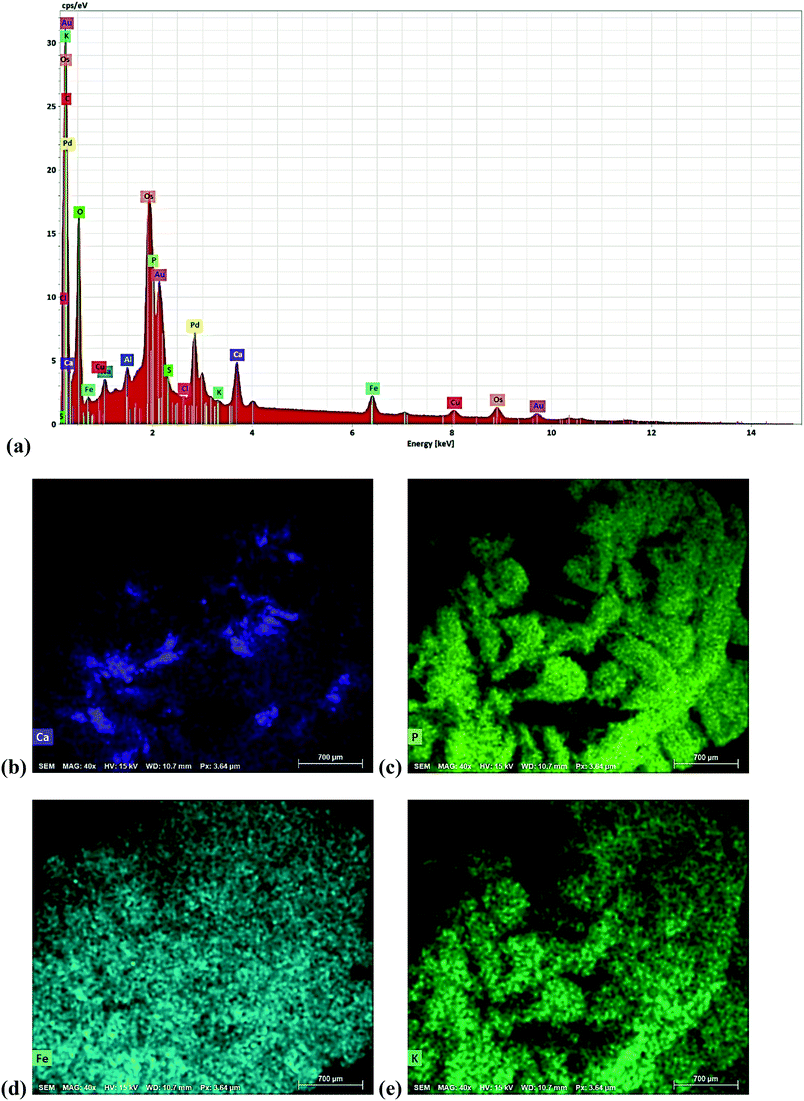 |
| Fig. 5 EDX analysis of aerobic granule for a) elemental composition map, b) calcium, c) phosphorus, d) iron and e) potassium precipitates. | |
In addition, precipitates of P were also detected on granular surface as shown in Fig. 5c. P can form salt precipitates with Ca2+ and can be biologically removed from the aqueous media by aerobic granules as previously reported.42 Therefore, the presence of P precipitates on the surface of granules is an indication of phosphorous removal from wastewater. Previous research has shown that majority of Ca2+, P and Fe3+ precipitates occur in the core of the granules compared to the surface.43,44 Therefore, although EDX analysis were not conducted for the inside of the core but it is hypothesized that there would be a higher proportion of these multivalent ion precipitates. EPS also plays a key role in the accumulation and transferring of these elements from the aqueous phase into phosphate accumulating organisms (PAOs).45 Consequently, it is suspected that PAOs must have been inhabited within the granules that aided in the removal of P through EPS formation.
In a real-life full-scale wastewater treatment plant, it is suggested that granular reactor on an optimized continuous flow can be intermittently operated under anaerobic phase at low flow rate, thus, allowing selective pressure. This step can potentially ensure removal of P through anaerobic/aerobic phase while any surplus contaminant bypass during this period can be eliminated in the subsequent AOP stage due to increased retention time by low flow rate. An anaerobic feeding condition has also been linked to the formation of stable aerobic granules during a continuous flow reactor operation.46,47
The morphology of aerobic granules used in this study was analyzed through SEM and the images are shown in Fig. 6a. It is evident that filamentous bacteria were dominant on the surface of the granules. A similar formation of filamentous aerobic granules was reported by feeding sucrose at an OLR of 6 kg COD m−3 d−1.48 However, the appearance of filamentous bacteria was linked to the disintegration of granules due to mass transfer limitations. Furthermore, irregular shaped granules with filamentous outgrowth was also observed and labelled as the reason for poor settleability of granules, disintegration and subsequent washout of biomass.49,50 On the contrary, the filamentous granules observed in this study appeared more rigid and compact with a symmetrical distribution of filamentous bacteria on the surface and no indication of disintegration. It is understood that these granules did not suffer from mass transfer limitation because of a lower OLR which might have kept the growth of flocculant filamentous bacteria in control. Additionally, these filamentous bacteria appear to provide more stability for the granules by providing protection from toxic contaminants.51 Our previous study also reported the long-term stability of theses granules to be consistent over prolonged operation.11
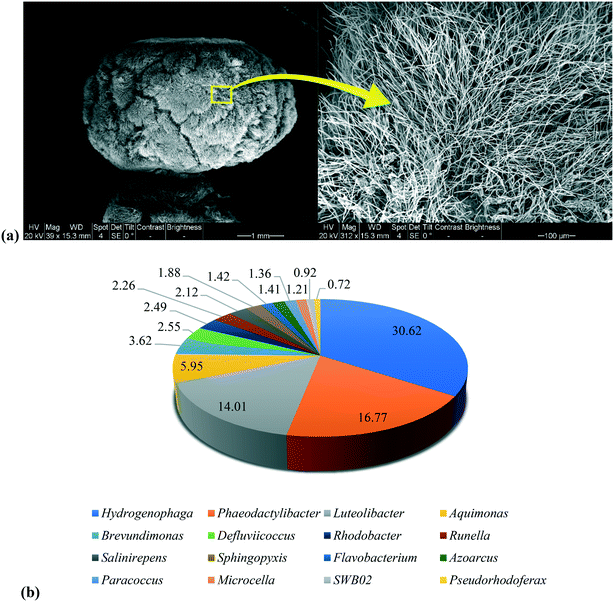 |
| Fig. 6 Microbial analysis on the a) morphology of granules through SEM and b) granule microbial community composition (top 16) at genus level through 16S rRNA gene sequencing analysis. | |
A vast variety of diverse microbial communities' harbor within the aerobic granules. DNA analysis showed Hydrogenophaga to be the dominant bacterial genus as shown in Fig. 6b. This genus and Pseudorhodoferax belong to Burkholderiaceae family which are known aromatic hydrocarbon pollutant degraders.52–54 More importantly, another classification of this genus is Pseudomonas55 which is considered as the main degrader of aromatic sulfonic acids such as sulfolane.56 Additionally, Paracoccus species have also been reported to degrade sulfolane.57Phaeodactylibacter, Paracoccus and Azoarcus are considered as denitrifiers with the ability to use both oxygen and nitrogen as electron acceptors, thus allowing them to survive in aerobic and anaerobic conditions effectively.58–60Paracoccus and Rhodobacter both belonging to Rhodobacteraceae family have also been detected with Burkholderiaceae in ecological wastewater treatment plants targeting pharmaceuticals removal.61 Therefore, it is understood that these genera can coexist together within the aerobic granules with the ability to degrade microcontaminants as observed in this study. Flavobacterium and Sphingopyxis genus were also identified in the 16S rRNA gene sequencing analysis of the mature aerobic granules. These microorganisms are important part of the granular community that aid in the production of EPS for granule stability and phosphorus accumulation.62 As indicated earlier, there was evidence of PAOs being inhabited within the aerobic granules in this study. Aquimonas and Luteolibacter genus were present in higher abundance as well.
4. Conclusion
In this paper, an integration of aerobic granulation and UV/H2O2 treatment has been shown as an effective approach to treat sulfolane in water. Aerobic granule process along with UV/H2O2 as a post biological treatment process can achieve more than 99.99% of sulfolane degradation within 6 h and 10 min. The adaption of aerobic granule in wastewater treatment can potentially boost the performance of biological treatment, while the addition of UV/H2O2 as a post-biological treatment can save space requirement in biological treatment to achieve the stringent discharge water guideline. The kinetics study revealed aerobic granulation process to follow zero order while UV/H2O2 process was strictly first order. Furthermore, it was found that the kinetics obtained from batch studies of the UV/H2O2 system can be used to predict sulfolane degradation kinetics in a continuous flow-through operation. H2O2 is one of the key operational parameters and one of the major costs for UV/H2O2. The H2O2 management study in this paper showed that the presence of hydrogen peroxide is necessary for fast degradation and high percentage degradation of sulfolane but the excess of H2O2 pose a potential threat to the discharge water receiving body. The selection of H2O2 concentration ultimately depends on the contaminant concentration, water matrix and the retention time. The continuous flow-through system also allowed a healthy granular system to flourish which was evidenced by the identification of multivalent elemental ions, such as Ca2+, Fe3+ and P, and diverse bacterial communities that aided in stable granular structure.
Conflicts of interest
There are no conflicts to declare.
Acknowledgements
The authors acknowledge the financial support provided by Bonavista Energy Corporation and the National Sciences and Engineering Research Council (NSERC) of Canada (Grant No. 10012306).
References
- P. M. Fedorak and D. L. Coy, Biodegradation of sulfolane in soil and groundwater samples from a sour gas plant site, Environ. Technol., 1996, 17, 1093–1102 CrossRef CAS.
- C. G. Kim, W. P. Clarke and D. Lockington, Anaerobic biodegradation of sulfolane in soil environment, Environ. Eng. Res., 1999, 4, 185–193 Search PubMed.
- C. P. Kasanke and M. B. Leigh, Factors limiting sulfolane biodegradation in contaminated subarctic aquifer substrate, PLoS One, 2017, 12, 1–17 CrossRef PubMed.
- S. M. Luther, M. J. Dudas and P. M. Fedorak, Sorption of sulfolane and diisopropanolamine by soils, clays and aquifer materials, J. Contam. Hydrol., 1998, 32, 159–176 CrossRef CAS.
-
O. Stewart and L. Minnear, Sulfolane Technical Assistance and Evaluation Report, 2010 Search PubMed.
- C. M. Thompson, D. W. Gaylor, J. A. Tachovsky, C. Perry, M. C. Carakostas and L. C. Haws, Development of a chronic noncancer oral reference dose and drinking water screening level for sulfolane using benchmark dose modeling, J. Appl. Toxicol., 2013, 33, 1395–1406 CrossRef CAS PubMed.
- E. A. Greene, L. M. Gieg, D. L. Coy and P. M. Fedorak, Sulfolane biodegradation potential in aquifer sediments at sour natural gas plant sites, Water Res., 1998, 32, 3680–3688 CrossRef CAS.
- E. A. Greene, P. H. Beatty and P. M. Fedorak, Sulfolane degradation by mixed cultures, and a bacterial isolate identified as a Variovorax sp., Arch. Microbiol., 2000, 174, 111–119 CrossRef CAS PubMed.
- E. A. Greene and P. M. Fedorak, Nutrient stimulation of sulfolane biodegradation in a contaminated soil from a sour natural gas plant and in a pristine soil, Environ. Technol., 2001, 22, 619–629 CrossRef CAS PubMed.
- M. Pronk, M. K. de Kreuk, B. de Bruin, P. Kamminga, R. Kleerebezem and M. C. M. van Loosdrecht, Full scale performance of the aerobic granular sludge process for sewage treatment, Water Res., 2015, 84, 207–217 CrossRef CAS PubMed.
- M. F. Khan, L. Yu, J. H. Tay and G. Achari, Coaggregation of bacterial communities in aerobic granulation and its application on the biodegradation of sulfolane, J. Hazard. Mater., 2019, 377, 206–214 CrossRef CAS PubMed.
- A. M. Maszenan, Y. Liu and W. J. Ng, Bioremediation of wastewaters with recalcitrant organic compounds and metals by aerobic granules, Biotechnol. Adv., 2011, 29, 111–123 CrossRef CAS PubMed.
- L. Yu, M. Mehrabani-Zeinabad, G. Achari and C. H. Langford, Application of UV based advanced oxidation to treat sulfolane in an aqueous medium, Chemosphere, 2016, 160, 155–161 CrossRef CAS PubMed.
- M. Izadifard, G. Achari and C. H. Langford, Mineralization of sulfolane in aqueous solutions by Ozone/CaO2 and Ozone/CaO with potential for field application, Chemosphere, 2018, 197, 535–540 CrossRef CAS PubMed.
- M. Mehrabani-Zeinabad, L. Yu, G. Achari and C. H. Langford, Mineralisation of sulfolane by UV/O3/H2O2 in a tubular reactor, J. Environ. Eng. Sci., 2016, 11, 44–51 CrossRef.
- M. Izadifard, G. Achari and C. H. Langford, Degradation of sulfolane using activated persulfate with UV and UV-Ozone, Water Res., 2017, 125, 325–331 CrossRef CAS PubMed.
- I. Muñoz, J. Rieradevall, F. Torrades, J. Peral and X. Domènech, Environmental assessment of different solar driven advanced oxidation processes, Sol. Energy, 2005, 79, 369–375 CrossRef.
- S. Giannakis, M. Voumard, D. Grandjean, A. Magnet, L. F. De Alencastro and C. Pulgarin, Micropollutant degradation, bacterial inactivation and regrowth risk in wastewater effluents: Influence of the secondary (pre)treatment on the efficiency of Advanced Oxidation Processes, Water Res., 2016, 102, 505–515 CrossRef CAS PubMed.
- M. F. Khan, L. Yu, G. Achari and J. H. Tay, Degradation of sulfolane in aqueous media by integrating activated sludge and advanced oxidation process, Chemosphere, 2019, 222, 1–8 CrossRef CAS PubMed.
- S. G. Zimmermann, M. Wittenwiler, J. Hollender, M. Krauss, C. Ort, H. Siegrist and U. von Gunten, Kinetic assessment and modeling of an ozonation step for full-scale municipal wastewater treatment: Micropollutant oxidation, by-product formation and disinfection, Water Res., 2011, 45, 605–617 CrossRef CAS PubMed.
- U. von Gunten, Ozonation of drinking water: Part II. Disinfection and by-product formation in presence of bromide, iodide or chlorine, Water Res., 2003, 37, 1469–1487 CrossRef CAS PubMed.
- B. H. Moon, Y. B. Park and K. H. Park, Fenton oxidation of Orange II by pre-reduction using nanoscale zero-valent iron, Desalination, 2011, 268, 249–252 CrossRef CAS.
- S. Giannakis, F. A. Gamarra Vives, D. Grandjean, A. Magnet, L. F. De Alencastro and C. Pulgarin, Effect of advanced oxidation processes on the micropollutants and the effluent organic matter contained in municipal wastewater previously treated by three different secondary methods, Water Res., 2015, 84, 295–306 CrossRef CAS PubMed.
-
L. J. Schmidt, M. P. Gaikowski and W. H. Gingerich, Environmental Assessment for the Use of Hydrogen Peroxide in Aquaculture for Treating External Fungal and Bacterial Diseases of Cultured Fish and Fish Eggs, Wisconsin, 2006 Search PubMed.
- A. Zamyadi, E. Sawade, L. Ho, G. Newcombe and R. Hofmann, Impact of UV–H2O2 Advanced Oxidation and Aging Processes on GAC Capacity for the Removal of Cyanobacterial Taste and Odor Compounds, Environ. Health Insights, 2015, 9, 1–10 Search PubMed.
-
Metcalf & Eddy Inc., Wastewater Engineering: Treatment and Reuse, McGraw-Hill, New York, 5th edn, 2013 Search PubMed.
-
J. G. Calvert and J. N. Pitts, Photochemistry, John Wiley and Sons, Inc., New York, 1966 Search PubMed.
- D. B. Miklos, R. Hartl, P. Michel, K. G. Linden and U. Hübner, UV/H2O2 process stability and pilot-scale validation for trace organic chemical removal from wastewater treatment plant effluents, Water Res., 2018, 136, 169–179 CrossRef CAS PubMed.
-
APHA, Standard Methods for the Examination of Water and Wastewater, American Public Health Association, American Water Works Association, Water Environment Federation, Washington, D.C., 2012 Search PubMed.
- S. S. Tiwari, O. T. Iorhemen and J. H. Tay, Aerobic granular sludge and naphthenic acids treatment by varying initial concentrations and supplemental carbon concentrations, J. Hazard. Mater., 2019, 362, 348–357 CrossRef CAS PubMed.
-
CCME, Canadian Environmental Quality Guidelines for Sulfolane: Water and Soil, 2006 Search PubMed.
- C. F. Bustillo-Lecompte, M. Mehrvar and E. Quiñones-Bolaños, Combined anaerobic-aerobic and UV/H2O2 processes for the treatment of synthetic slaughterhouse wastewater, J. Environ. Sci. Health, Part A: Toxic/Hazard. Subst. Environ. Eng., 2013, 48, 1122–1135 CrossRef CAS PubMed.
- T. R. Kent, C. B. Bott and Z. W. Wang, State of the art of aerobic granulation in continuous flow bioreactors, Biotechnol. Adv., 2018, 36, 1139–1166 CrossRef CAS PubMed.
- C. Wan, S. Sun, D. J. Lee, X. Liu, L. Wang, X. Yang and X. Pan, Partial nitrification using aerobic granules in continuous-flow reactor: Rapid startup, Bioresour. Technol., 2013, 142, 517–522 CrossRef CAS PubMed.
- D. Li, Y. Lv, M. Cao, H. Zeng and J. Zhang, Optimized hydraulic retention time for phosphorus and COD removal from synthetic domestic sewage with granules in a continuous-flow reactor, Bioresour. Technol., 2016, 216, 1083–1087 CrossRef CAS PubMed.
- S. S. Adav, D. J. Lee and N. Q. Ren, Biodegradation of pyridine using aerobic granules in the presence of phenol, Water Res., 2007, 41, 2903–2910 CrossRef CAS PubMed.
- G. C. Okpokwasili and C. O. Nweke, Microbial growth and substrate utilization kinetics, Afr. J. Biotechnol., 2005, 5, 305–317 Search PubMed.
- J. Zou, Y. Tao, J. Li, S. Wu and Y. Ni, Cultivating aerobic granular sludge in a developed continuous-flow reactor with two-zone sedimentation tank treating real and low-strength wastewater, Bioresour. Technol., 2018, 247, 776–783 CrossRef CAS PubMed.
- C. Chen, L. Bin, B. Tang, S. Huang, F. Fu, Q. Chen, L. Wu and C. Wu, Cultivating granular sludge directly in a continuous-flow membrane bioreactor with internal circulation, Chem. Eng. J., 2017, 309, 108–117 CrossRef CAS.
- S. Salmiati, F. A. Dahalan, M. Z. Mohamed Najib, M. R. Salim and Z. Ujang, Characteristics of developed granules containing phototrophic aerobic bacteria for minimizing carbon dioxide emission, Int. Biodeterior. Biodegrad., 2015, 102, 15–23 CrossRef.
- B. Kończak, J. Karcz and K. Miksch, Influence of Calcium, Magnesium, and Iron Ions on Aerobic Granulation, Appl. Biochem. Biotechnol., 2014, 174, 2910–2918 CrossRef PubMed.
- S. Johansson, M. Ruscalleda and J. Colprim, Phosphorus recovery through biologically induced precipitation by partial nitritation-anammox granular biomass, Chem. Eng. J., 2017, 327, 881–888 CrossRef CAS.
- M. Angela, B. Béatrice and S. Mathieu, Biologically induced phosphorus precipitation in aerobic granular sludge process, Water Res., 2011, 45, 3776–3786 CrossRef CAS PubMed.
- Y. C. Juang, S. S. Adav, D. J. Lee and J. H. Tay, Stable aerobic granules for continuous-flow reactors: Precipitating calcium and iron salts in granular interiors, Bioresour. Technol., 2010, 101, 8051–8057 CrossRef CAS PubMed.
- R. Wang, Y. Peng, Z. Cheng and N. Ren, Understanding the role of extracellular polymeric substances in an enhanced biological phosphorus removal granular sludge system, Bioresour. Technol., 2014, 169, 307–312 CrossRef CAS PubMed.
- L. Jahn, K. Svardal and J. Krampe, Comparison of aerobic granulation in SBR and continuous-flow plants, J. Environ. Manage., 2019, 231, 953–961 CrossRef CAS PubMed.
- M. K. de Kreuk and M. C. M. van Loosdrecht, Selection of Slow Growing Organisms as a Means for Aerobic Granular Sludge Stability, Water Sci. Technol., 2004, 49, 9–17 CrossRef CAS PubMed.
- Y.-M. Zheng, H.-Q. Yu, S.-J. Liu and X.-Z. Liu, Formation and instability of aerobic granules under high organic loading conditions, Chemosphere, 2006, 63, 1791–1800 CrossRef CAS PubMed.
- Y. Liu and Q. S. Liu, Causes and control of filamentous growth in aerobic granular sludge sequencing batch reactors, Biotechnol. Adv., 2006, 24, 115–127 CrossRef CAS PubMed.
- S. F. Corsino, R. Campo, G. Di Bella, M. Torregrossa and G. Viviani, Study of aerobic granular sludge stability in a continuous-flow membrane bioreactor, Bioresour. Technol., 2016, 200, 1055–1059 CrossRef CAS PubMed.
- S. T. L. Tay, B. Y. P. Moy, A. M. Maszenan and J. H. Tay, Comparing activated sludge and aerobic granules as microbial inocula for phenol biodegradation, Appl. Microbiol. Biotechnol., 2005, 67, 708–713 CrossRef CAS PubMed.
- L. Lin, H. Wang and P. Xu, Immobilized TiO2-reduced graphene oxide nanocomposites on optical fibers as high performance photocatalysts for degradation of pharmaceuticals, Chem. Eng. J., 2017, 310, 389–398 CrossRef CAS.
- E. A. Greene and P. M. Fedorak, A differential medium for the isolation and enumeration of sulfolane-degrading bacteria, J. Microbiol. Methods, 1998, 33, 255–262 CrossRef CAS.
- J. Min, B. Wang and X. Hu, Effect of inoculation of Burkholderia sp. strain SJ98 on bacterial community dynamics and para-nitrophenol, 3-methyl-4-nitrophenol, and 2-chloro-4-nitrophenol degradation in soil, Sci. Rep., 2017, 7, 1–9 CrossRef PubMed.
- A. Willems, J. Busse, M. Goor, B. Pot, E. Falsen, E. Jantzen, B. Hoste, M. Gillis, K. Kersters, G. Auling and J. De Ley, Hydrogenophaga, a New Genus of Hydrogen-Oxidizing Bacteria That Includes Hydrogenophaga flava comb. nov. (Formerly Pseudomonas flava), Hydrogenophaga palleronii (Formerly Pseudomonas palleronii), Hydrogenophaga pseudoflava (Formerly Pseudomonas pseudoflav), Int. J. Syst. Bacteriol., 1989, 39, 319–333 CrossRef CAS.
- N. A. Lee and D. P. Clark, A natural isolate of Pseudomonas maltophila which degrades aromatic sulfonic acids, FEMS Microbiol. Lett., 1993, 107, 151–155 CrossRef CAS PubMed.
- W. J. Lee, J. K. Lee, J. Chung, Y. J. Cho and D. H. Park, Effects of electrochemical
reduction reactions on the biodegradation of recalcitrant organic compounds (ROCs) and bacterial community diversity, J. Microbiol. Biotechnol., 2010, 20, 1230–1239 CrossRef CAS PubMed.
- B. Baumann, M. Snozzi, A. J. B. Zehnder and J. A. N. R. van der Meer, Dynamics of Denitrification Activity of Paracoccus denitrificans in Continuous Culture during Aerobic-Anaerobic Changes, J. Bacteriol., 1996, 178, 4367–4374 CrossRef CAS PubMed.
- Q. Ma, Y. Y. Qu, X. W. Zhang, W. L. Shen, Z. Y. Liu, J. W. Wang, Z. J. Zhang and J. T. Zhou, Identification of the microbial community composition and structure of coal-mine wastewater treatment plants, Microbiol. Res., 2015, 175, 1–5 CrossRef CAS PubMed.
- Z. Liu, A. Zhou, J. Zhang, S. Wang, Y. Luan, W. Liu, A. Wang and X. Yue, Hydrogen Recovery from Waste Activated Sludge: Role of Free Nitrous Acid in a Prefermentation-Microbial Electrolysis Cells System, ACS Sustainable Chem. Eng., 2018, 6, 3870–3878 CrossRef CAS.
- I. N. Balcom, H. Driscoll, J. Vincent and M. Leduc, Metagenomic analysis of an ecological wastewater treatment plant's microbial communities and their potential to metabolize pharmaceuticals, F1000Research, 2016, 5(1881), 1–21 Search PubMed.
- P. Świątczak and A. Cydzik-Kwiatkowska, Performance and microbial characteristics of biomass in a full-scale aerobic granular sludge wastewater treatment plant, Environ. Sci. Pollut. Res., 2018, 25, 1655–1669 CrossRef PubMed.
- K. Apel and H. Hirt, Reactive oxygen species: Metabolism, Oxidative Stress, and Signal Transduction, Annu. Rev. Plant Biol., 2004, 55, 373–399 CrossRef CAS PubMed.
- A. Latifi, M. Ruiz and C. C. Zhang, Oxidative stress in cyanobacteria, FEMS Microbiol. Rev., 2009, 33, 258–278 CrossRef CAS PubMed.
- A. Burson, H. C. P. Matthijs, W. de Bruijne, R. Talens, R. Hoogenboom, A. Gerssen, P. M. Visser, M. Stomp, K. Steur, Y. van Scheppingen and J. Huisman, Termination of a toxic Alexandrium bloom with hydrogen peroxide, Harmful Algae, 2014, 31, 125–135 CrossRef CAS PubMed.
- M. Ksibi, Chemical oxidation with hydrogen peroxide for domestic wastewater treatment, Chem. Eng. J., 2006, 119, 161–165 CrossRef CAS.
Footnote |
† Electronic supplementary information (ESI) available. See DOI:10.1039/c9ew01048c |
|
This journal is © The Royal Society of Chemistry 2020 |