Enhanced wettability of long narrow carbon nanotubes in a double-walled hetero-structure: unraveling the effects of a boron nitride nanotube as the exterior†
Received
8th September 2019
, Accepted 21st November 2019
First published on 23rd November 2019
Abstract
Studying the structure and dynamics of nano-confined water inside carbon nanotubes has consistently attracted the wide-spread interest of researchers. In the present work, molecular dynamics simulations indicated internal nonwetting behavior for the central region of the long and narrow single-wall carbon nanotube (5,5) (SWNT) and showed that continuous single-file water molecules are not formed through it. Unlike the SWNT, by adding boron nitride nanotubes (6,6) as an outer wall to the SWNT, a continuously long single-file water chain is formed through the double-walled carbon and boron nitride hetero-nanotube (DWHNT) and thorough internal wetting of the DWHNT is observed. The position and the number of water molecules, electrostatic potential heatmap of the nanotube's wall, free energy profile of nano-confined water, and number of hydrogen bonds between them confirmed the aforementioned results and complete internal wetting of the DWHNT. After using the boron nitride nanotube (6,6) as the outer wall, an homogeneous electrostatic potential distribution in the DWHNT and increase in the hydrophilic characteristics of the nano-channel wall are observed, bringing about gradual trapping of more water molecules through it. Finally, water molecules occupied the central region of the DWHNT and a thorough single-file water chain is formed inside the nano-channel. Water dipole orientation inside the DWHNT and their radial distribution function asserted the occurrence of the liquid–solid quasi-phase transition of single-file water molecules confined inside the long and narrow carbon nanotube (5,5) under ambient conditions.
1. Introduction
The extraordinarily fast and selective transport and confinement of water in the nanoscale have a wide range of applications such as in drug delivery, filtration, biosensors, and, nanofluidics.1–6 Investigations of diffusion and permeation of water molecules in artificial water channels as a promising subject is of great importance to researchers, which can provide new ideas in the design of synthetic channels with similar performance to biological water channels, including selectivity and salt rejection properties. In recent years, the application of carbon nanotubes (CNTs) for water transport and confinement in the molecular scale has attracted research interests.7–15 Due to their symmetric structure, unique electrical properties, and chemical, mechanical and thermal stability, CNTs are promising building blocks for transporter channels and desalination membranes. Water confined in CNTs behaves indeed differently compared to bulk water.16 Fast permeation of water molecules inside CNTs and their internal wetting follows from the hydrophobic wall of the CNTs, weak interaction between water and a frictionless surface at the CNT wall, and continuously ordered hydrogen bonds between water molecules.17–22 Hydrogen bond formation between water molecules and the CNT wall, which affects the water flow rate through the CNT, is not possible in the depletion region at the water–CNT interface.23 Furthermore, Strogatz et al. achieved remarkable long slip length for the CNT permeating water, confirming the frictionless surface at the CNT wall and high fluid velocities through it.18 Hummer et al. in 2001 observed internal wetting of narrow CNTs by molecular dynamics simulation for the first time. They reported that the one-dimensional single-file water chain immediately fills the CNT and the wetting behavior of the inner surface of the CNT changes between wetting and nonwetting states on a nanosecond timescale.17 Wang et al. as well as Hummer et al. demonstrated that the single-file water chain, leading to fast water and proton permeation, is only formed inside CNTs with a diameter from 6.76 to 8.11 Å. They also revealed that the wetting behavior of the CNT interior and properties of water nano-confined inside them are dramatically affected by the diameter of the CNTs.24–27 CNTs transport rapidly not only the water liquid, but also light gases and Skoulidas et al. confirmed the relevance of fluid diffusivities through CNTs and the pore size of the nanotubes. In this regard, they notified that a higher curvature of CNTs with a smaller diameter results in a smoother molecule–nanotube potential energy surface and eventually faster transport.28 In addition to the effect of the diameter on the wetting of CNTs, the dependency of water permeation through CNTs and their internal wetting on the length of the nanotubes is proved by Waghe et al. As a consequence, in long and narrow CNTs, only partial wetting occurs at the beginning and the end of the nanotubes and minor water molecules could permeate through it.29,30 Accordingly, Walter et al. by investigating the barrier superfast water permeation reported that the water transport enhancement rate is attributed to the slip length for long CNTs, whereas it is governed by entrance/exit losses for short CNTs.31
Moreover, confined water in CNTs could have multiple phase structures and phase transitions.32 Noon et al. investigated the probable formation of ordered ice sheets inside the CNTs in 2002. Under ambient conditions, they found highly ordered hydrogen-bond networks among confined water molecules inside CNTs with special diameters, giving rise to disordered to ordered structure transition.33–37 By tuning the CNT diameter under the ambient conditions, nano-confined water in the CNT shows a liquid–solid critical point at which liquid and solid coexist and also the interface between them vanishes. Due to this unusual critical point which is not observed for bulk water, a direct and continuous liquid–solid transition occurs in nano-confined water inside the CNTs.38 Ice formation and crystal shapes inside CNTs are reliant on the nanotube diameters and wetting behavior.39–41 The phase transition could be described by the two-dimensional square-lattice model proposed by Ising. Although based on the Ising model, ordered phase and phase transition cannot occur in the one-dimensional single-file water chain,42 in 2007, Mukherjee et al. predicted solid-like ordering structure for single-file water molecules inside CNT (6,6) under ambient conditions.43 Even in 2015, Kumar et al. carried out molecular dynamics simulation on confined water inside long CNTs (6,6) using different water models to prove the solid-like ordering structure of the single-file water chain. Inside long CNTs (6,6), the single-file water chain exhibits a solid-like ordering structure arising due to the stronger hydrogen bond between the next and the previous neighbor water molecule in the chain.44,45 In accordance with the previous studies, Ma et al. observed a liquid–solid quasi-phase transition of the single-file water chain that is confined inside very narrow CNTs with a diameter range from 5.4 to 9 Å by decreasing the temperature.46 They assigned temperature-induced changes of the water dipole orientation that are obtained from molecular dynamics simulations to the quasi-phase transition occurring in the single-file water chain inside the CNT (6,5).46 The CNT (5,5) with short length has the smallest nano-channel that water molecules can permeate through as a highly ordered structure, single-file water chain.24,34 Infrequent water permeation inside the CNT (5,5) often arises from translational entropy originating from the translational movement of water molecules.47 Single-file water chain formation inside the CNT (5,5) and absolute internal wetting are length-dependent: the longer the CNT, the higher the surface friction. Considering this high energy barrier, wetting could not occur in the central region of the long CNT (5,5).30,48–50 In the present work, a thorough internal wetting of a long CNT (5,5) is observed by using a short-length boron nitride nanotube (6,6) as an exterior wall. Furthermore, the implementation of the aforesaid outer wall led to the liquid–solid quasi-phase transition of the single-file water chain occurrence in the long CNT (5,5). Introducing a computational framework for the wetting of narrow and long nanotubes using targeted structural improvements and investigating the role of such modifications in the wetting procedure of the internal surface of carbon nanotubes are considered as the novelty of this research study.
2. Computational method
2.1. Simulation details
Simulations were carried out using molecular dynamics software NAMD51 using a timestep of 1 fs. The CHARMM force field has the ability to take the harmonic potential expression into consideration for stretching and angle terms, along with specific expressions for dihedral and cross-terms. Moreover, it is capable of considering non-bonded terms as a function of the Lennard-Jones and Coulomb electrostatic potentials. Based on the terms describing the CHARMM force field, all the interatomic and intermolecular interaction parameters of the components inside the system, including the water model used, have also been reported in the previous studies. Hence, the CHARMM36 force field parameters52,53 were utilized for the CNT, water, ions, and, graphene. Lennard-Jones parameters for nitrogen and boron atoms and their partial charges are taken from Won et al.54 (oN–N′ = 0.3365 nm, εN–N = 0.6060 kJ mol−1, oB–B′ = 0.3453 nm, εB–B = 0.3971 kJ mol−1). The TIP3P water model55 and rigid bonds between oxygen and hydrogen atoms in water molecules are applied. The simulation of nano-channels is carried out in the NVT ensemble at T = 300 K, controlled by a Langevin thermostat and using a damping coefficient of 5 ps−1. Periodic boundary conditions are employed in all directions. Electrostatic interactions are calculated using the particle mesh Ewald (PME) method over a cubic grid with a ∼1 Å spacing,56 and a cutoff (10–12 Å) is implemented for van der Waals (VdW) interactions. The electrostatic potential map of nano-channels is computed by VMD software and further information regarding the calculation method is elucidated in the ESI.†
2.2. System configuration
In order to investigate the formation and properties of a single-file water chain inside long and narrow CNTs, a CNT with indices of (5,5) armchair with a length of about 6 nm is chosen. Based on the aim of our work, a double-walled hetero-nanotube is made by employing the CNT (5,5) as the inner wall. A boron nitride nanotube with indices of (6,6) with a length of about 2 nm is also considered as the outer wall of the double-walled hetero-nanotube. The aforementioned boron nitride nanotube is coaxially placed at the central region of the CNT (5,5). The diameter of the CNT (5,5) and the boron nitride nanotube (6,6) is 6.78 and 8.14 Å, respectively. Two water boxes of different sizes in the Z direction with ions in them are constructed by Packmol.57 A water box with dimensions of (40 × 40 × 40) Å3, containing 2102 water molecules, is added to the top of the CNT along the Z-axis and another box with dimensions of (40 × 40 × 20) Å3 containing 1052 water molecules is appended to the bottom of the CNT. Sodium and chloride ions are added into the mentioned water boxes with a concentration of 1 M. To prevent water from permeating out of the nano-channel, CNT (5,5) and double-walled carbon–boron nitride hetero-nanotubes are separated by two graphene sheets with dimensions of (40 × 40) Å2. Fig. 1 illustrates the side view of the initial configuration of the CNT (5,5) and double-walled carbon–boron nitride hetero-nanotube. The snapshots are rendered by VMD.58 The simulation of the CNT (5,5) and double-walled carbon–boron nitride hetero-nanotube is performed for about 30 ns. For the sake of calculating the lifetime of the hydrogen bonds between water molecules confined inside the nano-channels, an additional simulation of the systems is carried out for 1 ns starting at frame 30 ns. During these 1 ns runs, coordinates are recorded with a frequency of 20 fs.
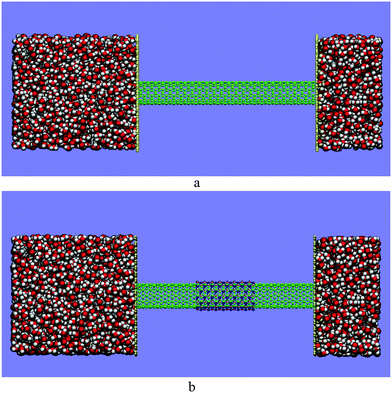 |
| Fig. 1 The side view for the initial configuration of (a) the CNT (5,5) and (b) double-walled carbon–boron nitride hetero-nanotube embedded between two water boxes along the Z axis. | |
In the subsequent sections, the CNT (5,5) nano-channel and double-walled carbon–boron nitride hetero-nanotube are denoted by SWNT and DWHNT, respectively.
3. Results and discussion
In view of realizing the internal wetting behavior of the hetero-nanotube and the formation of the single-file water chain inside it, the number of water molecules and hydrogen bonds between them, positions of water molecules along the Z-axis versus the time inside the SWNT and DWHNT and free energy profile of water molecules permeating through the nano-channels are studied in Section 3.1. In Section 3.2, the radial distribution function of confined water, the dipole moment orientation of water molecules, the lifetime of the hydrogen bond between water molecules inside the hetero-nanotube and the electrostatic potential heatmap of nano-channels were all evidence of liquid–solid quasi-phase transition and solid-like ordering of the single-file water chain inside the DWHNT.
3.1. Internal wetting behavior of the hetero-nanotube
3.1.1. The number of water molecules and the hydrogen bonds between them inside the hetero-nanotube.
In order to ensure the equilibrium of water, the root mean square deviation (RMSD) for water molecules was evaluated. More descriptions are provided in the ESI† and the RMSD diagrams for both systems are depicted in Fig. S1 (ESI†). For the SWNT and the DWHNT nano-channels, the number of water molecules as a function of time is depicted in Fig. 2. It is noticed that water molecules do not permeate through SWNTs due to steric constraints and hydrophobic properties of the SWNT wall. In contrast, during 30 ns, only a small number of water molecules of about 5 which entered the SWNT was immediately expelled. The number of water molecules confined inside the DWHNT was boosted up to 22 by the evolution of time. The long created single-file water chain inside the DWHNT is not always continuous and connected during the whole simulation time, so, the number of confined water molecules sometimes reached a value lower than 22. It is noticed that the thorough internal wetting of the long CNT (5,5) along with the formation of the long and stable single-file water chain inside it is followed by applying a boron nitride nanotube (6,6) as the outer wall.
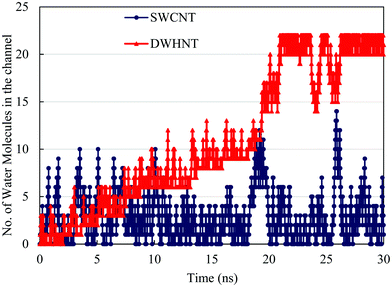 |
| Fig. 2 The number of confined water molecules inside the SWNT and the DWHNT. | |
Fig. 3 illustrates the snapshots of the SWNT and DWHNT channels along with the water chain formation state. According to Fig. 3, one can perceive that it is not possible to form a water chain in the SWNT system under the mentioned conditions, while in the DWHNT nano-channel a one-dimensional chain of confined water molecules can be apparently detected.
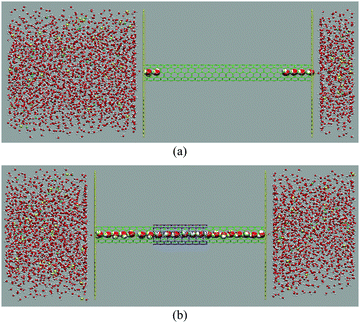 |
| Fig. 3 Side view snapshot of (a) the SWNT while the confined water molecule chain is not created and (b) the DWHNT with the continuous single-file water chain inside it. | |
In order to assure the formation of the single-file water chain inside the DWHNT, the average number of hydrogen bonds between confined water molecules is calculated which is displayed versus time in Fig. 4. A hydrogen bond can be defined when the donor–acceptor distance is considered to be less than 3.5 Å, and the angle between them is less than 30°.59 Obviously, the average number of hydrogen bonds between water molecules confined inside the SWNT, was about 2. While it fluctuates around a given value, the average number of hydrogen bonds inside the DWHNT increased with the increment of time. At 20–30 nanoseconds, when the single-file water chain was perfectly formed inside the DWHNT, the average number of hydrogen bonds between confined water molecules was about 8. Despite the results of the number of confined water molecules inside the DWHNT, indicating the thorough formation of the one-dimensional single-file water network, the average number of hydrogen bonds which was about 8 suggests that a long and thorough single-file water chain was occasionally formed. Thus the orientation of 22 confined water molecules inside the DWHNT was not simultaneously proper to create hydrogen bonds between all of them in the chain.
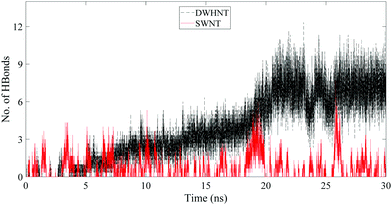 |
| Fig. 4 The average number of hydrogen bonds between confined water molecules inside the SWNT and the DWHNT versus time. | |
3.1.2. Trajectories of water molecules inside the hetero nano-channel.
For the sake of investigating the permeation of the water molecules and the level of wettability of the internal walls in more detail, the Z positions of the water molecules inside SWNT and DWHNT nano-channels in terms of simulation time are depicted in Fig. 5 and 6, respectively.
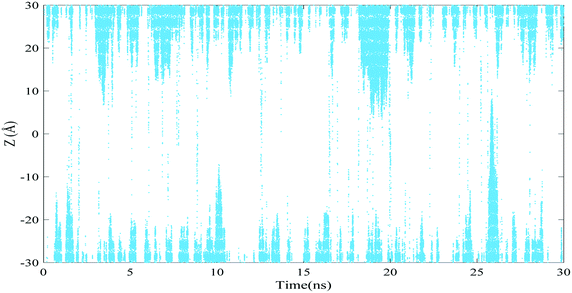 |
| Fig. 5 The Z coordination of water molecules over the simulation time for the SWNT nano-channel. | |
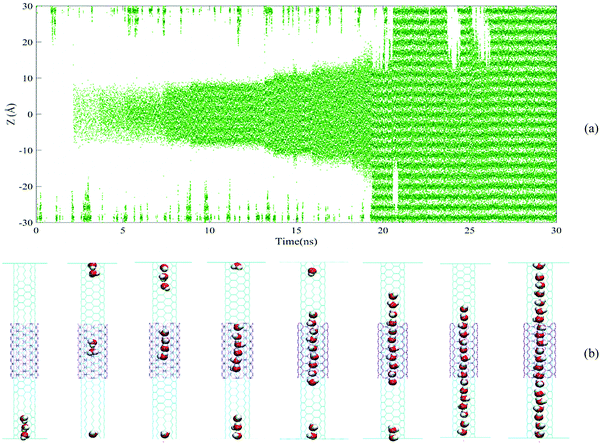 |
| Fig. 6 (a) The time evolution for Z coordination of water molecules inside a DWHNT hetero-channel, (b) snapshots of water molecules inside a DWHNT hetero-channel. | |
The time evolution for the position of water molecules along the Z-axis in Fig. 5 demonstrates that in the case of the SWNT nano-channel, the single-file of water molecules has not been completely formed along the full length of the (5,5) CNT. Furthermore, in most instances, the inner walls of the nanotube, especially in the central regions, are dehydrated. As illustrated in Fig. 5, in the SWNT nano-channel, a large number of water molecules entered the (5,5) carbon nanotubes from the upper and lower domains, but only a small number of the molecules could extend the entire length of the nanotube and permeate through it.
In Fig. 6(a), the position of water molecules along the Z-axis is shown in terms of the simulation time for the DWHNT hetero-nanotube. This diagram demonstrates that a small number of water molecules that could permeate through the center of the (5,5) carbon nanotube were trapped by the boron nitride nanotubes in the center of the nano-channel. At the beginning of the simulation, almost no water molecule permeates, and the central regions of Fig. 6(a) are thoroughly empty. But after 2.5 ns, only a few water molecules permeated through the central domain of the hetero-nanotube and were trapped by the short boron nitride nanotube in the Z range of −10 Å to 10 Å. After 17.5 ns, the number of trapped water molecules in this range increased, followed by the fact that the central regions of the hetero-nanotube in the Z range of −15 Å to 15 Å are completely hydrated. At the end of the simulation, the single-file of water molecules was completely formed throughout the hetero-nanotubes in the Z range of −30 Å to 30 Å. As a consequence of the short length of the boron nitride nanotube in the DWHNT nano-channel, the single-file of water molecules was formed gradually after 21 ns. In Fig. 6(b), the full wetting mechanism of the internal wall of the DWHNT hetero-nanotube is illustrated using the nano-channel snapshots. By providing the favorable conditions for the formation of the single-file of water molecules inside the (5,5) carbon nanotube and the wettability of its internal wall, the boron-nitride nanotube has increased the hydrophilic features of hetero-nanotubes.
3.1.3. Water molecules in the hetero-nanochannel: free energy assessment.
In order to illustrate the effects of nano-channel walls on permeability and formation of the single-file of water molecules, the free energy profile in the SWNT and DWHNT nano-channels was plotted as shown in Fig. 7. The free energy profile of water molecules can be calculated as a function of their longitudinal position inside the channel using the distribution function. The Boltzmann relation between the free energy and the distribution density of the water molecules permeating through the nano-channel was used to calculate the free energy barrier for water molecules inside the channel:60,61 | A(z) = −kBT ln n(z) + kBT ln C | (1) |
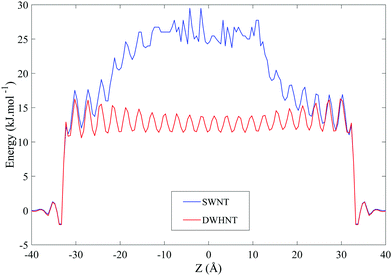 |
| Fig. 7 The free energy of water molecules in terms of their position inside the SWNT and DWHNT nano-channels. | |
In eqn (1), n(z) represents the number density of the water molecules and k, A and T are Boltzmann constant, free energy, and temperature respectively. In eqn (1), “C” is a constant parameter to set the free energy profile of water molecules to zero at the bulk solvent.60,61 Water molecules encountered an energy barrier of about 17.5 kJ mol−1 at the entrance of the SWNT nano-channel, and straightforwardly entered from the top and bottom domains of the nano-channel, but this energy barrier present throughout the SWNT nano-channel, especially in the Z range of −15 Å to 15 Å increased to 25 kJ mol−1, preventing the continuous and permanent permeation of water molecules.
The energy barrier in the DWHNT hetero-nanotube has reduced to 14 kJ mol−1. This energy barrier is not the same throughout the entire length of the nanotube, so that in the central domain of nanotube in which the boron nitride nanotube is located – in the Z range of −15 Å to 15 Å – the energy barrier reaches its minimum level. With the double-walled hetero-nanotube utilizing the boron nitride nanotube, water molecules encountered a lower energy barrier and this results in the possibility of forming the complete single-file water molecules.
3.2. Liquid–solid quasi-phase transition inside the hetero-nanotube
3.2.1. Axial distribution function of water inside the hetero nanotube.
Mukherjee in 2007 and Kumar in 2015 studied the structure and dynamics of a single-file of water molecules inside (6,6) carbon nanotubes with different lengths. They identified a solid-like ordering for the single-file of water molecules enclosed within the nanotube at ambient temperature and conditions by investigating the axial distribution function of water molecules.43,44
In Fig. 8, it is inferred from the axially projected distribution function of the oxygen atoms inside the hetero-nanotube that the single-file of water molecules is formed in the quasi-solid order at room temperature. In the axial distribution function for oxygen of water molecules inside the hetero-nanotubes, distinct peaks appeared with a periodicity of 2.6 Å, which are perfectly consistent with the results obtained by Chakraborty et al. for a single-file of water molecules inside the carbon nanotube (6,6).43–45
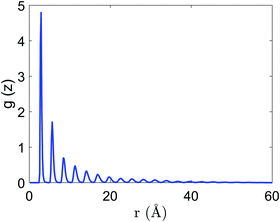 |
| Fig. 8 The axial distribution function of confined water molecules in the DWHNT system. | |
3.2.2. Dipole moment orientation of water and electrostatic heatmap of the hetero nanotube.
In 2017, Ma et al. investigated the phase transition and the formation of regular quasi-ice structures in highly narrow carbon nanotubes at low temperatures. They studied the orientational order of the single-file dipoles inside the carbon nanotube using molecular dynamics simulation and numerically indicated that the dipole orientation of a single-file of water molecules encompasses a wide range of angles at room temperature which is mostly distributed at two angles of 150° and 30°. However, this wide range decreases by reducing the temperature as well, and eventually, the most probable dipole orientation in low temperatures was obtained at about 38°. They identified that the alterations of the dipole orientation which can be ascribed to the reduction of temperature, can be considered as an indicator for the quasi-phase transition of the single-file of water inside the carbon nanotube.46
In Fig. 9, the orientation of the dipole moment of water molecules within the SWNT and DWHNT nano-channels with respect to the Z-axis is shown in three time intervals. As depicted in Fig. 9, a wide range of the dipole orientation of water inside the DWHNT is observed in the time interval from 0 to 10 ns (beginning of simulation). Nevertheless, two distinct peaks in the probability distribution of dipole orientation were indicated at 38° and 145°, which is highly consistent with the dipole orientation of the single-file of water within the very narrow carbon nanotubes.62
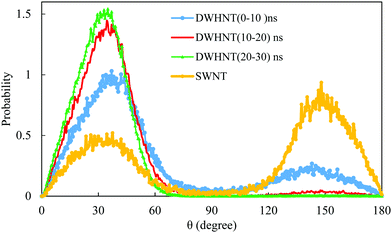 |
| Fig. 9 The distribution of dipole orientation of permeating water molecules inside: the SWNT during the time interval 0 to 30 ns and the DWHNT during 0 to 10 ns, 10 to 20 ns, and 20 to 30 ns. | |
In the time interval from 10 to 20 ns, the distribution of the dipole orientation of the water molecules in different directions was reduced. Rather, the probability distribution in the direction of 38° raised during this time interval, and finally, in the interval from 20 to 30 ns (end of simulation), the dipole orientation of water molecules at 38° takes the maximum amount of probability. The results of water dipole distribution are in complete agreement with the ones for water molecules inside the narrow carbon nanotubes presented by Ma et al. in 2017.46
In Fig. 9, the dipole moment distribution of the water molecules inside the SWNT nano-channel is shown in the time interval from 0 to 30 ns. As illustrated in Fig. 9, water molecules are aligned in a wide range of dipole angles; however, the highest distribution was observed in both directions at 38° and 145°. The mentioned changes in the patterns of dipole orientation of water inside the hetero-nanotube in three different time intervals and the distinction between the dipole orientational pattern for water within the SWNT can be ascribed to a phase transition from liquid to solid-like in the single-file of one-dimensional water molecules, that is observed due to the presence of the boron nitride nanotube around the CNT.
The water permeability for the SWNT and DWHNT was obtained at about (1.2709 ± 0.1382) × 10−14 and 0 cm3 s−1, respectively. The particular method of calculating water permeability is clarified in the supplementary information. Attaining the water permeability to the zero level after complete wettability of the internal surface of the DWHNT can confirm the liquid to solid-like phase transition of the single-file of water molecules within the heterogeneous nanotubes.
The electrostatic heatmap for the SWNT and DWHNT nano-channels is depicted in Fig. 10. The electrostatic contour plot shows that the potential is very high in the center of the SWNT nano-channel. Consequently, the central domain of the nano-channel is not electrostatically favorable for the presence of water molecules. The water molecules inside the SWNT are aligned in the most probable dipole orientations (38° and 145°) due to the difference in the electrostatic potential between the entrance regions of the SWNT nano-channel and water reservoirs at the top and bottom.63 Nevertheless, the most probable dipole orientation of the water molecules inside the DWHNT channel is only about 38° relative to the Z-axis. According to the electrostatic contour plot in Fig. 10(b), the monotonic form of the electrostatic potential across the whole length of the DWHNT and reduction in the electrostatic potential difference between the central and entrance domains of the nano-channel facilitated the entry of water molecules into it. Thus, the dipole moment of the water molecules inside the hetero nanotubes was orientated in the same direction, which results in a highly regular solid-like structure. Moreover, it is inferred from Fig. 10 that the electrostatic distribution of the DWHNT vicinity is distinctly effective on the wetting of the inner wall and the formation of the single-file of water molecules inside the nano-channel. Due to the different patterns of charge distribution on the BNNT compared to the CNT and distinct properties of van der Waals interaction of water molecules with the BNNT, it seems that the interaction between the water molecules and the outer layer differs remarkably from the interaction of the water molecules with the CNT. Subsequently, the heterogeneous nano-channel system with an exterior layer was designed to improve the wettability properties of the CNT channel. The electrostatic heatmap of the nano-channel confirms the formation of a single-file of water molecules inside the DWHNT, which clearly signifies that the boron nitride nanotube, due to the presence of nitrogen and boron atoms, made the hetero channel highly hydrophilic.
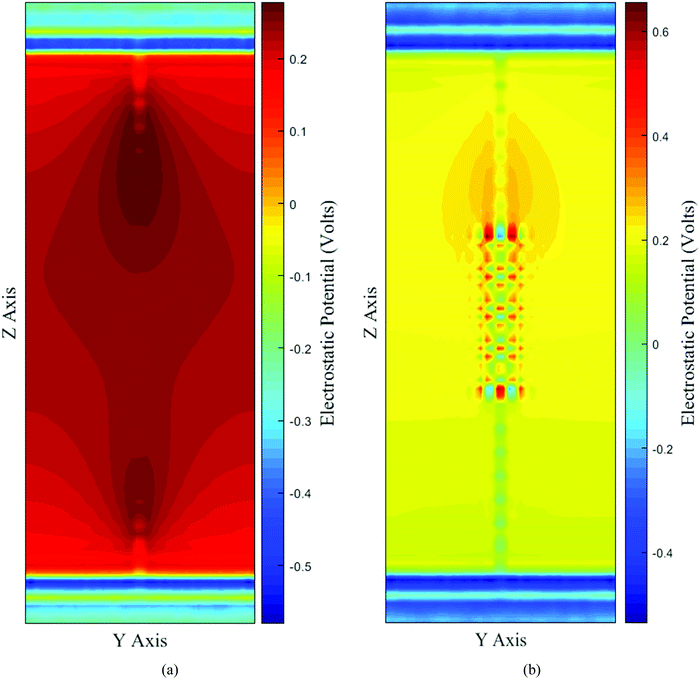 |
| Fig. 10 Electrostatic heatmap for: (a) the SWNT nano-channel in the ZX plane, and (b) the DWHNT nano-channel in the ZY plane. | |
3.2.3. Stability of hydrogen bonds between water molecules inside the nano-channels.
In order to investigate the formation mechanism of the single-file of solid-like ordered water inside the DWHNT nanotube more precisely, the stability of the hydrogen bonds between water molecules in the single-file along with their lifetime was evaluated.
In this regard, the hydrogen bond autocorrelation function (HBACF) was determined to analyze the stability of hydrogen bonds using eqn (2).59 The HBACF diagram for hydrogen bonds between water molecules inside the SWNT and DWHNT nano-channels is depicted in Fig. 11.
| 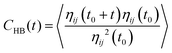 | (2) |
In
eqn (2), if the hydrogen bond between
i and
j occurs at time
t, the variable
ηij(
t) – bond order operator – takes the value zero, otherwise it will receive 1 as the value. The particular point of reference to consider in a hydrogen bond is that the donor–acceptor distance is considered to be less than 3.5 Å, and the angle between them is less than 30°.
59 Afterwards, a fitting procedure was carried out on the HBACF outputs to obtain the required parameters for the long time decay of the autocorrelation functions according to
eqn (3):
| 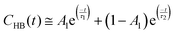 | (3) |
Finally, the lifetime of the hydrogen bond was acquired using
eqn (4):
64 | τHB(t) ≅ A1τ1 + (1 − A1)τ2 | (4) |
τHB for the single-file of water molecules inside the SWNT and DWHNT nano-channels was indicated to be 355.405 and 780.2 fs, respectively. In
Fig. 11, the hydrogen bond correlation decays exponentially with the simulation time. The correlation of the hydrogen bonds between water molecules inside the DWHNT nano-channel has decayed more quickly than in the SWNT. Accordingly, the retention time of hydrogen bonds between the water molecules in the DWHNT is less than the one in the SWNT which implies that the water molecules inside the DWHNT nano-channel show a faster rearrangement than the ones in the SWNT.
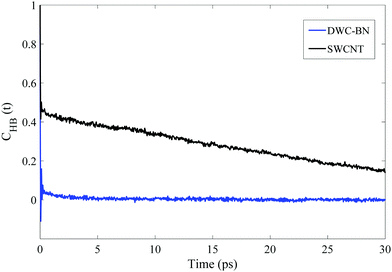 |
| Fig. 11 Hydrogen bond autocorrelation function for water molecules inside the SWNT and DWHNT. | |
Due to the reduced lifetime of hydrogen bonds between water molecules inside the DWHNT, it is deduced that the hydrogen bonding between water molecules is not the only determinant factor in forming the single-file structure for the water molecules. The strong interaction between water and the boron nitride nanotube, and the favorable electrostatic potential inside the nano-channel played an essential role in the formation of the single-file of water molecules. Presence of the boron nitride nanotube in the central domain of the hetero nano-channel has greatly enhanced the hydrophilicity of the nano-channel. Correspondingly, the tendency of water molecules to interact with or orientate towards the nano-channel wall will be amplified compared to their tendency to form a stronger hydrogen bond with adjacent water molecules. Therefore, the lifetime of hydrogen bonding between water molecules inside the DWHNT was reduced remarkably.
4. Summary and concluding remarks
As a consequence of the significant electrostatic energy in the central domains of the long (5,5) carbon nanotubes, the single-file water chain within the SWNT is not formed, and the proper wettability of the inner surface of the nano-channel does not occur. However, in the DWHNT hetero-nanochannel, a long single-file of water molecules inside the (5,5) carbon nanotube was formed and propagated throughout the whole length of the nano-channel. As a paradigmatic modification of the SWNT nano-channel, the addition of boron nitride nanotubes as the outer wall of the SWNT has increased the number of water molecules within the nano-channel and subsequently raised the number of hydrogen bonds between them.
The free energy profile of the water molecules in the SWNT shows that water molecules encounter a large energy barrier of up to 25 kJ mol−1 for permeating through a long (5,5) carbon nanotube. Nonetheless, the energy barrier in the DWHNT nano-channel has been reduced up to 14 kJ mol−1 resulting from the presence of boron nitride nanotubes as an outer wall. The electrostatic heatmap for DWHNT nano-channels suggests that the boron nitride nanotube has led to the formation of a single-file of water molecules inside the channel by increasing the hydrophilicity of the nano-channel and making the electrostatic distribution uniform. The distinct peaks appeared with a specific periodicity in the radial distribution function of oxygen atoms within the hetero nano-channel, zeroing the amount of water permeability through the DWHNT, and the sharp peaks of dipole orientation distribution at 38° for water molecules inside the DWHNT can be considered as the pieces of evidence for the liquid–solid quasi-phase transition and the formation of a solid-like ordered structure in a single-file of water molecule in one dimension. Owing to the short lifetime of hydrogen bonds between water molecules inside the DWHNT, the prominent factors for the formation of the solid-like structure of the single-file of water molecules inside the nano-channel are desirable electrostatic circumstances around the nano-channel and the stronger interactions of water molecules with the inner surface of the nano-channel due to the presence of the boron nitride nanotube. Making an assessment on the behavior of water inside an extremely narrow DWHNT nanotube with a diameter of about 6.78 Å can substantially assist in understanding the behavior of water molecules in complex inorganic systems.
Conflicts of interest
There are no conflicts to declare.
References
- Y. Cheng, X. Jiao, L. Zhao, Y. Liu, F. Wang, Y. Wen and X. Zhang, Wetting Transition in Nanochannels for Biomimetic Free-Blocking on-Demand Drug Transport, J. Mater. Chem. B, 2018, 6(39), 6269–6277 RSC.
- X. Chen, H. Zhang, R. H. Tunuguntla and A. Noy, Silicon Nanoribbon PH Sensors Protected by a Barrier Membrane with Carbon Nanotube Porins, Nano Lett., 2018, 19(2), 629–634 CrossRef PubMed.
- S. M. Fatemi and M. Foroutan, Review on Carbon Nanotubes and Carbon Nanotube Bundles for Gas/Ion Separation and Water Purification Studied by Molecular Dynamics Simulation, Int. J. Environ. Sci. Technol., 2016, 13(2), 457–470 CrossRef CAS.
- I. Ihsanullah, Carbon Nanotube Membranes for Water Purification: Developments, Challenges, and Prospects for the Future, Sep. Purif. Technol., 2019, 209, 307–337 CrossRef.
- A. Noy, H. G. Park, F. Fornasiero, J. K. Holt, C. P. Grigoropoulos and O. Bakajin, Nanofluidics in Carbon Nanotubes, Nano Today, 2007, 2(6), 22–29 CrossRef.
- V. F. Naeini, M. Foroutan, M. Maddah, Y. Rémond and M. Baniassadi, Determinative Factors in Inhibition of Aquaporin by Different Pharmaceuticals: Atomic Scale Overview by Molecular Dynamics Simulation, Biochim. Biophys. Acta, Gen. Subj., 2018, 1862(12), 2815–2823 CrossRef PubMed.
- B. H. S. Mendonça, D. N. de Freitas, M. H. Köhler, R. J. C. Batista, M. C. Barbosa and A. B. de Oliveira, Diffusion Behaviour of Water Confined in Deformed Carbon Nanotubes, Phys. A, 2019, 517, 491–498 CrossRef.
- K. Falk, F. Sedlmeier, L. Joly, R. R. Netz and L. Bocquet, Molecular Origin of Fast Water Transport in Carbon Nanotube Membranes: Superlubricity versus Curvature Dependent Friction, Nano Lett., 2010, 10(10), 4067–4073 CrossRef CAS PubMed.
- V. V. Chaban, V. V. Prezhdo and O. V. Prezhdo, Confinement by Carbon Nanotubes Drastically Alters the Boiling and Critical Behavior of Water Droplets, ACS Nano, 2012, 6(3), 2766–2773 CrossRef CAS PubMed.
- G. Cao, Y. Qiao, Q. Zhou and X. Chen, Infiltration Behaviour of Water in a Carbon Nanotube under External Pressure, Philos. Mag. Lett., 2008, 88(5), 371–378 CrossRef CAS.
- F. Ebrahimi, F. Ramazani and M. Sahimi, Nanojunction Effects on Water Flow in Carbon Nanotubes, Sci. Rep., 2018, 8(1), 7752 CrossRef PubMed.
- F. Lv, C. Fang and J. Su, Enhanced Water Transport through a Carbon Nanotube Controlled by the Lateral Pressure, Nanotechnology, 2019, 30(24), 245707 CrossRef CAS PubMed.
- S. Chen, Y. Cheng, G. Zhang and Y.-W. Zhang, Spontaneous Directional Motion of Water Molecules in Single-Walled Carbon Nanotubes with a Stiffness Gradient, Nanoscale Adv., 2019, 1(3), 1175–1180 RSC.
- J. Tao, X. Song, T. Zhao, S. Zhao and H. Liu, Confinement Effect on Water Transport in CNT Membranes, Chem. Eng. Sci., 2018, 192, 1252–1259 CrossRef CAS.
- K. Goh and Y. Chen, Controlling Water Transport in Carbon Nanotubes, Nano Today, 2017, 14, 13–15 CrossRef CAS.
- X. Qin, Q. Yuan, Y. Zhao, S. Xie and Z. Liu, Measurement of the Rate of Water Translocation through Carbon Nanotubes, Nano Lett., 2011, 11(5), 2173–2177 CrossRef CAS PubMed.
- G. Hummer, J. C. Rasaiah and J. P. Noworyta, Water Conduction through the Hydrophobic Channel of a Carbon Nanotube, Nature, 2001, 414(6860), 188–190 CrossRef CAS PubMed.
- M. Majumder, N. Chopra, R. Andrews and B. J. Hinds, Nanoscale Hydrodynamics: Enhanced Flow in Carbon Nanotubes, Nature, 2005, 438(7064), 44 CrossRef CAS PubMed.
- D. A. Britz and A. N. Khlobystov, Noncovalent Interactions of Molecules with Single Walled Carbon Nanotubes, Chem. Soc. Rev., 2006, 35(7), 637–659 RSC.
- M. Melillo, F. Zhu, M. A. Snyder and J. Mittal, Water Transport through Nanotubes with Varying Interaction Strength between Tube Wall and Water, J. Phys. Chem. Lett., 2011, 2(23), 2978–2983 CrossRef CAS PubMed.
- A. Striolo, The Mechanism of Water Diffusion in Narrow Carbon Nanotubes, Nano Lett., 2006, 6(4), 633–639 CrossRef CAS PubMed.
- H. Kyakuno, K. Matsuda, Y. Nakai, R. Ichimura, T. Saito, Y. Miyata, K. Hata and Y. Maniwa, Rotational Dynamics and Dynamical Transition of Water inside Hydrophobic Pores of Carbon Nanotubes, Sci. Rep., 2017, 7(1), 14834 CrossRef PubMed.
- S. Joseph and N. R. Aluru, Why Are Carbon Nanotubes Fast Transporters of Water?, Nano Lett., 2008, 8(2), 452–458 CrossRef CAS PubMed.
- J. Wang, Y. Zhu, J. Zhou and X.-H. Lu, Diameter and Helicity Effects on Static Properties of Water Molecules Confined in Carbon Nanotubes, Phys. Chem. Chem. Phys., 2004, 6(4), 829–835 RSC.
- M. Rana and A. Chandra, Filled and Empty States of Carbon Nanotubes in Water: Dependence on Nanotube Diameter, Wall Thickness and Dispersion Interactions, J. Chem. Sci., 2007, 119(5), 367–376 CrossRef CAS.
- J. Köfinger, G. Hummer and C. Dellago, Macroscopically Ordered Water in Nanopores, Proc. Natl. Acad. Sci. U. S. A., 2008, 105(36), 13218–13222 CrossRef PubMed.
- J. Köfinger, G. Hummer and C. Dellago, Single-File Water in Nanopores, Phys. Chem. Chem. Phys., 2011, 13(34), 15403–15417 RSC.
- A. I. Skoulidas, D. M. Ackerman, J. K. Johnson and D. S. Sholl, Rapid Transport of Gases in Carbon Nanotubes, Phys. Rev. Lett., 2002, 89(18), 185901 CrossRef PubMed.
- A. Waghe, J. C. Rasaiah and G. Hummer, Filling and Emptying Kinetics of Carbon Nanotubes in Water, J. Chem. Phys., 2002, 117(23), 10789–10795 CrossRef CAS.
- J. Su and H. Guo, Effect of Nanotube-Length on the Transport Properties of Single-File Water Molecules: Transition from Bidirectional to Unidirectional, J. Chem. Phys., 2011, 134(24), 244513 CrossRef PubMed.
- J. H. Walther, K. Ritos, E. R. Cruz-Chu, C. M. Megaridis and P. Koumoutsakos, Barriers to Superfast Water Transport in Carbon Nanotube Membranes, Nano Lett., 2013, 13(5), 1910–1914 CrossRef CAS PubMed.
- E. M. Kotsalis, J. H. Walther and P. Koumoutsakos, Multiphase Water Flow inside Carbon Nanotubes, Int. J. Multiphase Flow, 2004, 30(7–8), 995–1010 CrossRef CAS.
- W. H. Noon, K. D. Ausman, R. E. Smalley and J. Ma, Helical Ice-Sheets inside Carbon Nanotubes in the Physiological Condition, Chem. Phys. Lett., 2002, 355(5–6), 445–448 CrossRef CAS.
- R. J. Mashl, S. Joseph, N. R. Aluru and E. Jakobsson, Anomalously Immobilized Water: A New Water Phase Induced by Confinement in Nanotubes, Nano Lett., 2003, 3(5), 589–592 CrossRef CAS.
- Y. Maniwa, H. Kataura, M. Abe, S. Suzuki, Y. Achiba, H. Kira and K. Matsuda, Phase Transition in Confined Water inside Carbon Nanotubes, J. Phys. Soc. Jpn., 2002, 71(12), 2863–2866 CrossRef CAS.
- Y. Maniwa, H. Kataura, M. Abe, A. Udaka, S. Suzuki, Y. Achiba, H. Kira, K. Matsuda, H. Kadowaki and Y. Okabe, Ordered Water inside Carbon Nanotubes: Formation of Pentagonal to Octagonal Ice-Nanotubes, Chem. Phys. Lett., 2005, 401(4–6), 534–538 CrossRef CAS.
- K. Ritos, M. K. Borg, N. J. Mottram and J. M. Reese, Electric Fields Can Control the Transport of Water in Carbon Nanotubes, Philos. Trans. R. Soc., A, 2016, 374(2060), 20150025 CrossRef PubMed.
- K. Koga, G. T. Gao, H. Tanaka and X. C. Zeng, Formation of Ordered Ice Nanotubes inside Carbon Nanotubes, Nature, 2001, 412(6849), 802 CrossRef CAS PubMed.
- D. Takaiwa, I. Hatano, K. Koga and H. Tanaka, Phase Diagram of Water in Carbon Nanotubes, Proc. Natl. Acad. Sci. U. S. A., 2008, 105(1), 39–43 CrossRef CAS PubMed.
- O. Byl, J.-C. Liu, Y. Wang, W.-L. Yim, J. K. Johnson and J. T. Yates, Unusual Hydrogen Bonding in Water-Filled Carbon Nanotubes, J. Am. Chem. Soc., 2006, 128(37), 12090–12097 CrossRef CAS PubMed.
- S. Dalla Bernardina, E. Paineau, J.-B. Brubach, P. Judeinstein, S. Rouzière, P. Launois and P. Roy, Water in Carbon Nanotubes: The Peculiar Hydrogen Bond Network Revealed by Infrared Spectroscopy, J. Am. Chem. Soc., 2016, 138(33), 10437–10443 CrossRef CAS PubMed.
- E. Ising, Beitrag Zur Theorie Des Ferromagnetismus, Z. Phys. A: Hadrons Nucl., 1925, 31(1), 253–258 CrossRef CAS.
- B. Mukherjee, P. K. Maiti, C. Dasgupta and A. K. Sood, Strong Correlations and Fickian Water Diffusion in Narrow Carbon Nanotubes, J. Chem. Phys., 2007, 126(12), 124704 CrossRef PubMed.
- H. Kumar, C. Dasgupta and P. K. Maiti, Structure, Dynamics and Thermodynamics of Single-File Water under Confinement: Effects of Polarizability of Water Molecules, RSC Adv., 2015, 5(3), 1893–1901 RSC.
- S. Chakraborty, H. Kumar, C. Dasgupta and P. K. Maiti, Confined Water: Structure, Dynamics, and Thermodynamics, Acc. Chem. Res., 2017, 50(9), 2139–2146 CrossRef CAS PubMed.
- X. Ma, S. Cambré, W. Wenseleers, S. K. Doorn and H. Htoon, Quasiphase Transition in a Single File of Water Molecules Encapsulated in (6,5) Carbon Nanotubes Observed by Temperature-Dependent Photoluminescence Spectroscopy, Phys. Rev. Lett., 2017, 118(2), 27402 CrossRef PubMed.
- A. K. Giri, F. Teixeira and M. N. D. S. Cordeiro, Structure and Kinetics of Water in Highly Confined Conditions: A Molecular Dynamics Simulation Study, J. Mol. Liq., 2018, 268, 625–636 CrossRef CAS.
- X. Zhang, W. Zhou, F. Xu, M. Wei and Y. Wang, Resistance of Water Transport in Carbon Nanotube Membranes, Nanoscale, 2018, 10(27), 13242–13249 RSC.
- M. E. Suk and N. R. Aluru, Modeling Water Flow through Carbon Nanotube Membranes with Entrance/Exit Effects, Nanoscale Microscale Thermophys. Eng., 2017, 21(4), 247–262 CrossRef CAS.
- W. D. Nicholls, M. K. Borg, D. A. Lockerby and J. M. Reese, Water Transport through (7, 7) Carbon Nanotubes of Different Lengths Using Molecular Dynamics, Microfluid. Nanofluid., 2012, 12(1–4), 257–264 CrossRef CAS.
- J. C. Phillips, R. Braun, W. Wang, J. Gumbart, E. Tajkhorshid, E. Villa, C. Chipot, R. D. Skeel, L. Kale and K. Schulten, Scalable Molecular Dynamics with NAMD, J. Comput. Chem., 2005, 26(16), 1781–1802 CrossRef CAS PubMed.
- R. B. Best, X. Zhu, J. Shim, P. E. M. Lopes, J. Mittal, M. Feig and A. D. MacKerell Jr, Optimization of the Additive CHARMM All-Atom Protein Force Field Targeting Improved Sampling of the Backbone ϕ, ψ and Side-Chain X1 and X2 Dihedral Angles, J. Chem. Theory Comput., 2012, 8(9), 3257–3273 CrossRef CAS PubMed.
- J. Huang, S. Rauscher, G. Nawrocki, T. Ran, M. Feig, B. L. de Groot, H. Grubmüller and A. D. MacKerell Jr, CHARMM36m: An Improved Force Field for Folded and Intrinsically Disordered Proteins, Nat. Methods, 2017, 14(1), 71 CrossRef CAS PubMed.
- C. Y. Won and N. R. Aluru, Structure and Dynamics of Water Confined in a Boron Nitride Nanotube, J. Phys. Chem. C, 2008, 112(6), 1812–1818 CrossRef CAS.
- W. L. Jorgensen, J. Chandrasekhar, J. D. Madura, R. W. Impey and M. L. Klein, Comparison of Simple Potential Functions for Simulating Liquid Water, J. Chem. Phys., 1983, 79(2), 926–935 CrossRef CAS.
- T. Darden, D. York and L. Pedersen, Particle Mesh Ewald: An N.Log (N) Method for Ewald Sums in Large Systems, J. Chem. Phys., 1993, 98(12), 10089–10092 CrossRef CAS.
- L. Martínez, R. Andrade, E. G. Birgin and J. M. Martínez, PACKMOL: A Package for Building Initial Configurations for Molecular Dynamics Simulations, J. Comput. Chem., 2009, 30(13), 2157–2164 CrossRef PubMed.
- W. Humphrey, A. Dalke and K. Schulten, VMD: Visual Molecular Dynamics, J. Mol. Graphics, 1996, 14(1), 33–38 CrossRef CAS PubMed.
- A. Luzar and D. Chandler, Hydrogen-Bond Kinetics in Liquid Water, Nature, 1996, 379(6560), 55 CrossRef CAS.
- J. L. Trick, S. Chelvaniththilan, G. Klesse, P. Aryal, E. J. Wallace, S. J. Tucker and M. S. P. Sansom, Functional Annotation of Ion Channel Structures by Molecular Simulation, Structure, 2016, 24(12), 2207–2216 CrossRef CAS PubMed.
- M. Foroutan, V. F. Naeini and M. Ebrahimi, Carbon Nanotubes Encapsulating Fullerene as Water Nano-Channels with Distinctive Selectivity: Molecular Dynamics Simulation, Appl. Surf. Sci., 2019, 489, 198–209 CrossRef CAS.
- J. Su and K. Yang, On the Origin of Water Flow through Carbon Nanotubes, ChemPhysChem, 2015, 16(16), 3488–3492 CrossRef CAS PubMed.
- L. Ruiz, Y. Wu and S. Keten, Tailoring the Water Structure and Transport in Nanotubes with Tunable Interiors, Nanoscale, 2015, 7(1), 121–132 RSC.
- S. Murail, T. Vasiliu, A. Neamtu, M. Barboiu, F. Sterpone and M. Baaden, Water Permeation across Artificial I-Quartet Membrane Channels: From Structure to Disorder, Faraday Discuss., 2018, 209, 125–148 RSC.
Footnotes |
† Electronic supplementary information (ESI) available. See DOI: 10.1039/c9cp04977k |
‡ V. Fadaei Naeini and M. Ebrahimi contributed equally to this work. |
|
This journal is © the Owner Societies 2020 |
Click here to see how this site uses Cookies. View our privacy policy here.