Aqueous systems of a surface active ionic liquid having an aromatic anion: phase behavior, exfoliation of graphene flakes and its hydrogelation†
Received
10th August 2019
, Accepted 17th November 2019
First published on 18th November 2019
Abstract
Surface active ionic liquid (SAIL) induced hydrogelation, in the absence of additives, is important considering the properties of soft-hydrogels that can be utilized in different applications. The present study is concerned with the phase behavior and hydrogelation of a SAIL, 1-hexadecyl-3-methylimidazolium p-toluenesulfonate, [C16mim][PTS]. The obtained information about the phase behavior along with the surfactant like behavior of the SAIL was exploited for effective exfoliation of graphene-flakes from graphite in aqueous medium that remain stable for at least one month. Thus the obtained dispersion of graphene-flakes was subsequently hydrogelated exploiting the observations made from the phase behavior of the SAIL, via entanglement of long worm-like micelles of the SAIL formed at higher concentration. The obtained graphene-flake based hydrogels were found to be equally stable as compared to the blank hydrogel as well as against centrifugation. The low melting point of hydrogel facilitates the extraction of graphene-flakes from the hydrogel matrix by heating and diluting the gel and there is no sign of agglomeration in the extracted graphene-flakes even if the extraction is carried out after a period of three months. The present work is an exemplary study on exfoliation, hydrogelation and extraction of graphene-flakes from a hydrogel, when required, using a SAIL and is expected to provide a new platform for utilization of SAILs for efficient graphene exfoliation and subsequent preparation of functional materials.
1. Introduction
Ionic liquids (ILs), which are composed entirely of ions and have a melting point below 100 °C, have attracted great interest from the scientific community in the past two decades for diverse applications1–3 owing to their unique physicochemical properties. Depending upon the nature of the constituent ions, many ILs display amphiphilic character, which places them in the category of surfactants. Such amphiphilic ILs are generally called surface active ionic liquids (SAILs).4–8 To date, a variety of SAILs have been investigated, where many of them were found to exhibit even better surface active properties as compared to those of homologous conventional ionic surfactants.9–24 Considering the better surface activity of SAILs, studies on the preparation and charaterization of supramolecular hydrogels by SAILs, in the presence of additives or by mixing SAILs with other solvents, have been performed.25–29 The obtained supramolecular hydrogels were indeed weak in strength in line with that reported for conventional ionic surfactants30–33 for different applications34–37 as compared to those obtained by using polymers due to weaker intermolecular interactions. However, the thermoreversibility along with the greater flexibility and dynamics of these hydrogels is advantageous over polymer based gels.38 It is expected that the applicability of SAIL based additive free hydrogels could be extended to exfoliation and subsequent hydrogelation of two dimensional materials (TDMs) such as graphene, MnS2, BN, etc.39–41
Among these TDMs, graphene is an extraordinary material owing to its unique electrical and other physico-chemical properties.39,42–44 Efficient utilization of graphene for different applications requires thin graphene layers in a highly dispersed form. For extracting such thin layers from graphite, different methods have been reported in the literature.45–52 Among these methods, liquid phase exfoliation (LPE)50,51 is the most commonly used technique, which involves direct sonication of graphene in solution.50,51 However, most of the organic solvents used in LPE show poor dispersion stability along with high toxicity. On the other hand, the use of aqueous medium for exfoliation of graphene is eco-friendly, however, it is limited by poor dispersion stability owing to the hydrophobic nature of graphene. Therefore, water-soluble polymers,52 biomacromolecules,53 and surfactants54–57 which act as an interface between graphene and water have been exploited for exfoliation and stabilization of graphene in aqueous medium.
Given the above background, we herein report a new strategy wherein a SAIL, appended with an aromatic anion, is employed for exfoliation of graphene-flakes from graphite in aqueous medium and its subsequent hydrogelation by tuning the concentration of the SAIL. For this, we have employed a p-toluenesulphate (PTS) based SAIL, 1-hexadecyl-3-methylimidazolium p-toluenesulfonate, [C16mim][PTS], previously reported by our group.7 [PTS]− was chosen due to its hydrotropic nature,58 where hydrotropes exhibit weak amphiphilic behavior without showing any self-assembly and help in solubilizing organic moieties in aqueous medium.58 This along with the highly surface active nature of [C16mim]+ was thought to synergistically assist in exfoliation of graphene-flakes from graphite. A phase trasition from an aqueous solution of rod-like micelles of the SAIL at relatively lower concentrations to an interpenetrated network of elongated worm-like micelles at higher concentrations of the SAIL was employed to formulate graphene-flake based hydrogels from stable SAIL–graphene-flake dispersions. The thus prepared dispersions and hydrogels of graphene-flakes were characterized thoroughly using appropriate techniques. It is important to mention that neat ILs have also been employed for exfoliation of graphene by direct sonication,59–61 however, the high cost and viscosity of neat ILs are a limitation. To overcome this, some groups have investigated exfoliation of graphene by aqueous-polymer ionic liquid (PIL) systems, and successfully exfoliated graphene layers.62,63 However, to the best of our knowledge, there is no report on exfoliation and hydrogelation of graphene-flakes using a SAIL, which not only gives stable dispersions but also offers a platform to formulate SAIL–graphene-flake hydrogels, from which graphene can be extracted even after a period of 3 months without any significant agglomeration.
2. Experimental section
2.1. Materials
The SAIL, [C16mim][PTS], was used from the same batch that was reported earlier.7 [C16mim][PTS] was dried and characterized via1H NMR (Annexure S1, ESI†) for its structural integrity before use. For the sample preparation, deionized water with resistivity ≥18 MΩ cm at 298.15 K was used. Graphite was purchased from Sigma Aldrich (product no. 332461) and was used as received.
2.2. Methods
2.2.1. Phase behavior of the aqueous SAIL system.
For investigating the phase behavior of the SAIL in aqueous medium, samples were prepared by mixing the SAIL in the concentration range from 1 mmol L−1 to 150 mmol L−1 in 5 mL glass vials by gentle shaking. The phase behavior was investigated in the temperature range of 15–75 °C via the naked eye by inverting the vials.
2.2.2. Characterization of aqueous solution and hydrogels of the SAIL.
Differential scanning calorimetry (DSC) on the hydrogel was performed using a METTLER TOLEDO differential scanning calorimeter, DSC 3, instrument using an aluminium crucible in the temperature range of 25–70 °C at a scan rate of 2° min−1 under an N2 environment. SANS measurements were performed by using a SANS diffractometer at the Dhruva reactor, Babha Atomic Research Centre (BARC), Mumbai, India. The mean incident neutron beam wavelength was 5.2 Å with a wavelength resolution (Δλ/λ) of approximately 15%. A linear position-sensitive detector was used to detect scattered neutrons in an angular range of 0.5–15°. A 0.5 cm thick quartz sample holder was used at a temperature of 30 °C. The scattered neutrons were measured for wave-vector transfer Q (Q = 4π
sin(θ/2)/λ where θ is the scattering angle) in the range of 0.015–0.4 Å−1. The measured data were corrected for the background, empty cell contribution and transmission, and presented on an absolute scale using standard protocols. To minimize the incoherent scattering and to increase the contrast, the samples were prepared in D2O. Freeze Fracture-Transmission Electron Microscopy (FF-TEM) was performed using a Balzer BAF 400, Germany, at a temperature of −149 °C. For FF-TEM images, about 4 μL of the sample solution was placed on a 0.5 mm thick copper disk and covered with a second copper disk. The copper sandwich with the sample was frozen by plunging the sandwich into liquid propane which had been cooled with liquid nitrogen. Samples were examined with a ZEISS CEM 902. Rheological measurements of various gels were carried out using a rheometer (MCR-301). The temperature of the gel was maintained using a DC 50 water circulator.
2.2.3. Exfoliation, characterization and hydrogelation of graphene-flakes.
Aqueous solutions of [C16mim][PTS] at different concentrations (0.1 mmol L−1 to 10 mmol L−1), in the presence of 2.5 mg mL−1 of graphite were sonicated for 1 hour using a bath sonicator (Citizon CUB2.5, power 50 W, frequency 40 kHz). The thus formed dispersions were centrifuged at 1000 rpm for 60 minutes to remove unexfoliated graphite. The dark supernatant was recovered carefully. UV-visible absorption spectra of the thus obtained supernatant were measured using a UV-vis spectrophotometer (UV-1800 SHIMADZU) in the wavelength range of 300–800 nm using a quartz cuvette having a path length of 1 cm. Atomic force microscopy (AFM) was performed in tapping mode with a Nanoscope IIIa (Veeco). Samples were drop cast on a freshly prepared mica surface. Commercially available phosphorous doped silicon tips (Veeco, MPP-11100) were used as probes. All data were corrected with a 2nd order flattening procedure to clarify the surface patterns. Raman spectra of graphite powder and exfoliated graphene-flakes after drying the aqueous solution for 24 hours were recorded on a Renishaw Raman spectrophotometer in the range of 1000–300 cm−1 using an Ar-ion laser at a wavelength of 514 nm. X-ray diffraction patterns of graphite powder and exfoliated graphene-flakes were acquired on a SHIMADZU MAXIMA B70000 using a Cu-target in the 2θ range of 5–80°. Zeta-potential measurements were performed using a light scattering apparatus (Zeta-sizer, Nanoseries, Nano-ZS), Malvern Instruments, equipped with a built-in temperature controller having an accuracy of ±0.1 K at a scattering angle of 173° using a dip cell (ZEN-212). The exfoliated graphene-flakes were hydrogelated by increasing the concentration of the SAIL utilizing the information gained from the phase behavior studies. 1H NMR and 2D 1H–1H NOESY experiments were performed on a Brüker Ascend 500 spectrometer (AVANCE III HD console) with water suppression in a 10% D2O–90% H2O mixture using a 5 mm BBO (broad-band observation) double-channel probe equipped with z-gradients. Phase sensitive 1H homonuclear 2D NOESY NMR experiments were performed with experimental parameters: fid size 4096: F2 and 128: F1, number of scans: 32, mixing time 500 ms and the data obtained were processed using Topspin NMR software. For TGA measurements, exfoliated graphene-flakes were separated from the dispersion by centrifugation of the samples at 8000 rpm for 30 minutes followed by washing with methanol at least 5 times. Removal of the SAIL from the separated graphene-flakes has been confirmed by thermogravimetric analysis (TGA) (Fig. S1, ESI†) carried out using a HITACHI STA7200 thermal analysis system.
3. Results and discussion
3.1. Phase behavior of the SAIL
Fig. 1A shows the phase behavior as a function of temperature and concentration of the SAIL in aqueous medium, where different phases i.e. solution, viscous solution, loose gel and gel were observed depending upon the investigated conditions. In the temperature range of 15–35 °C, a clear solution was observed with an increase in the concentration of the SAIL up to 10 mmol L−1, which became viscous at a concentration of 20 mmol L−1 at 298.15 K. A further increase in the concentration of the SAIL up to 50 mmol L−1 resulted in the formation of a loose hydrogel, which transforms to a relatively stronger gel at and above 100 mmol L−1 of the SAIL. The thus formed hydrogels are transparent without any precipitation or crystallization. With a rise in temperature a changeover from the gel phase to a loose gel is observed above 35 and 45 °C in the case of 100 and 150 mmol L−1 of the SAIL, respectively. Above 55 °C, the loose gel transforms to a viscous solution. The presence of an endothermic peak at 58.8 °C (Fig. 1B) marks the phase transition of the hydrogel to a Newtonian liquid, while another endothermic peak at 59.3 °C suggests the breakdown of inter-micellar interactions. An increase in the zero shear viscosity (Fig. 1C) from 0.014 Pa s to 70 Pa s when going from the aqueous solution (5 mmol L−1 of the SAIL) to the hydrogel (150 mmol L−1 of the SAIL) is observed.64,65 The role of the counter ion in the phase behavior of the SAIL has been investigated by employing [C16mim][Cl] and [C16mim][Br]. No change in the phase behavior of [C16mim][Cl] and [C16mim][Br] has been observed with increasing concentration of the SAIL up to a concentration of 200 mmol L−1.
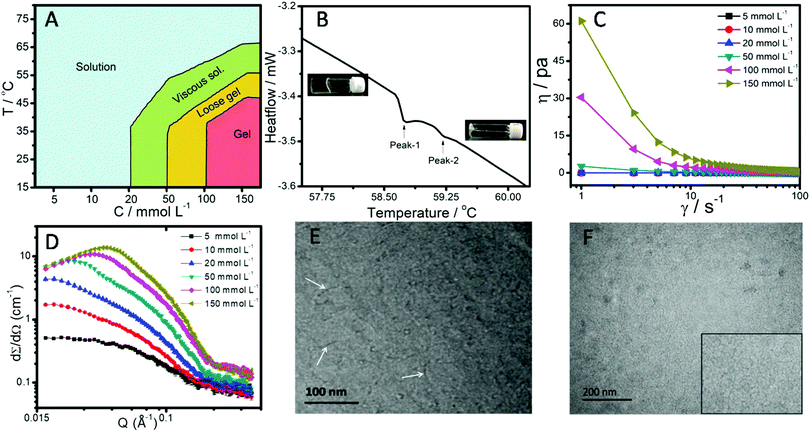 |
| Fig. 1 (A) Phase behavior of the SAIL in aqueous medium as a function of concentration and temperature; (B) DSC curve of the hydrogel obtained at a concentration of 150 mmol L−1 (gel phase) along with a photograph; (C) viscosity of an aqueous solution of the SAIL as a function of shear rate (γ); (D) representative SANS data pattern for different concentrations of the SAIL in aqueous medium; and FF TEM micro-graph of a (E) 20 mmol L−1, where smaller rod-like micelles are marked with arrows, and (F) 150 mmol L−1 concentration of the SAIL in aqueous medium (the inset of (F) is the enlarged view of the worm like micelles). | |
To gain insight into the microstructural origins of hydrogelation, SANS measurements (Fig. 1D) were perfomed. As can be seen from Fig. 1D, there is an increase in the scattering cross section with increasing concentration of the SAIL along with the appearance of a correlation peak that shifts toward higher Q value. The appearance of a correlation peak at and above 20 mmol L−1 of the SAIL indicates the presence of charge density and strong intermicellar interactions that lead to stability of the colloidal system. From the fitting of the measurement data to a suitable model, the dimensions and charge on micelles were obtained and are provided in Table S1 (ESI†). The obtained data about the size of micelles are in line with the formation of rod-like micelles at lower concentrations of the SAIL,7 which grow in length without much change in diameter at higher concentrations. Further, a negligible decrease in charge density after 50 mmol L−1 also supports the elongation of rod like micelles.66 To support the finding, FF-TEM (Fig. 1E and F) was performed, which confirms the presence of smaller rod-shaped micelles at relatively lower concentration, in line with that observed from TEM measurements.7 Long worm-like micelles, as judged from their varying curvature, are observed at the concentration corresponding to hydrogelation (Fig. 1F). The diameter of the elongated micelles is estimated to be ≈7 nm, while the length could not be estimated as the two ends of the elongated micelles are not clearly visible. Due to the bulky and relatively hydrophobic nature as compared to inorganic anions such as halides, [PTS]− has a stronger affinity towards the imidazolium head group, which effectively screens electrostatic repulsion between imidazolium head groups and promotes micellization and growth of micelles.7 It is natural to assume that the thus formed worm-like micelles entangle with each other to give rise to a three dimensional network entrapping water, resulting in hydrogelation of the aqueous solution. This behavior is similar to that reported for aqueous SAIL systems in the presence of additives such as sodium p-toluenesulfonate.64,67
3.2. SAIL assisted exfoliation of graphene-flakes and their characterization
Fig. 2A shows the photographs of aqueous dispersions of graphene-flakes obtained at different concentrations of the SAIL. The obtained aqueous dispersion of graphene-flakes in the presence of 1 mmol L−1 of the SAIL remains stable for 30 days (Fig. 2B), whereas the other dispersions remain stable for at least 24 hours (Fig. 2A). It is natural to assume that similar to surfactant mediated exfoliation of graphene in aqeuous solution,54,56 SAILs tend to incorporate into the graphene layers of graphite and facilitate the wetting of graphene layers, resulting in exfoliation of graphene-flakes. Similar to previous reports, the concentration of exfoliated graphene-flakes in aqueous solution was calculated by simple filteration and weighing of unexfoliated graphite-flakes.54 The maximum exfoliation of graphene-flakes i.e. 1 mg mL−1 is observed at a SAIL concentration of 1 mmol L−1 (Fig. 2C), the concentration at which the most stable dispersion, as compared to the other concentrations, is obtained. However, in the case of the SAIL having bromide and chloride as the counter anion, the maximum exfoliation of graphene-flakes i.e. 0.725 and 0.75 mg mL−1, respectively, is observed at a concentration of 4 mmol L−1 (Fig. S2, ESI†). This indicates the role of the [PTS]− anion in exfoliation of graphene-flakes to a relatively greater extent.
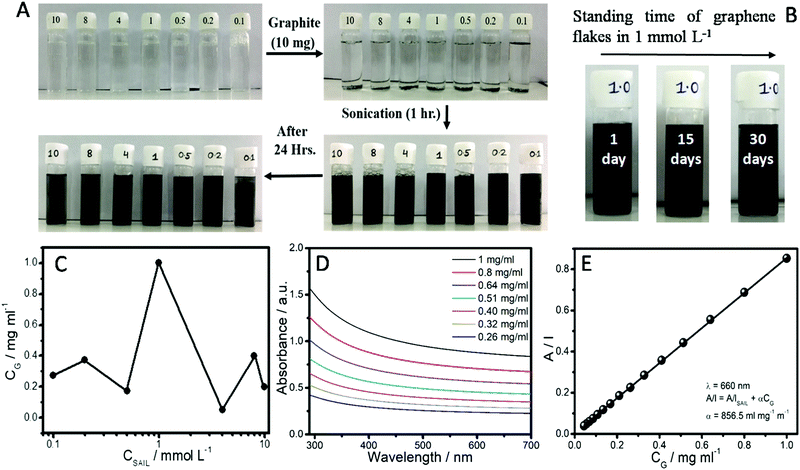 |
| Fig. 2 (A) Photographs showing SAIL mediated dispersion of graphene-flakes in aqueous solutions; (B) standing time of the dispersion of graphene-flakes in a 1 mmol L−1 concentration of the SAIL; (C) concentration of exfoliated graphene-flakes (CG) as a function of the concentration of the SAIL (CSAIL); (D) representative absorption spectra of the graphene-flake dispersion with varying SAIL concentration and (E) absorbance per unit length at 660 nm as a function of the graphene-flake concentration. | |
Further, depending upon the initial concentration of graphite and the sonication time, the extent of exfoliation obtained in this study i.e. 1 mg mL−1 is better than conventional surfactants and biopolymers.56,68 Henceforth, the system having graphene-flakes dispersed in a 1 mmol L−1 concentration of the SAIL will be discussed, if not mentioned otherwise. The absorption coefficient (α), an important parameter in characterizing the dispersions, is obtained from the UV-visible absorption spectra (Fig. 2D and E) of the supernatant obtained after centrifuging the dispersions. The solution with maximum exfoliated graphene-flakes i.e. 1 mg mL−1 was diluted several times to record the absorption spectra with varying concentration of graphene-flakes. The observed absorption spectra (Fig. 2D and Fig. S3, ESI†) are flat and featureless as expected for quasi two-dimensional materials including graphene.54,59 Employing the established method,54 the absorbance at 660 nm divided by the cell length (A/l) versus the concentration of dispersed graphene-flakes was used to calculate α by applying a straight fit (Fig. 2E). The obtained value of α is found to be 856 L g−1 m−1, which is lower than the reported α values for various solvents and surfactants.45 The obtained lower α value may be attributed to the presence of the SAIL in the dispersion medium.69
AFM measurements were performed to have statistical data about the size (length and breadth) and thickness of the exfoliated graphene-flakes (Fig. 3). Graphene-flakes varying in dimensions were observed from AFM measurements (Fig. 3A). The obtained height profile shows a mean height of ≈12 nm, however the obtained profile is positively skewed with a skewness of 0.59, the kurtosis value for which comes out to be −1.15.
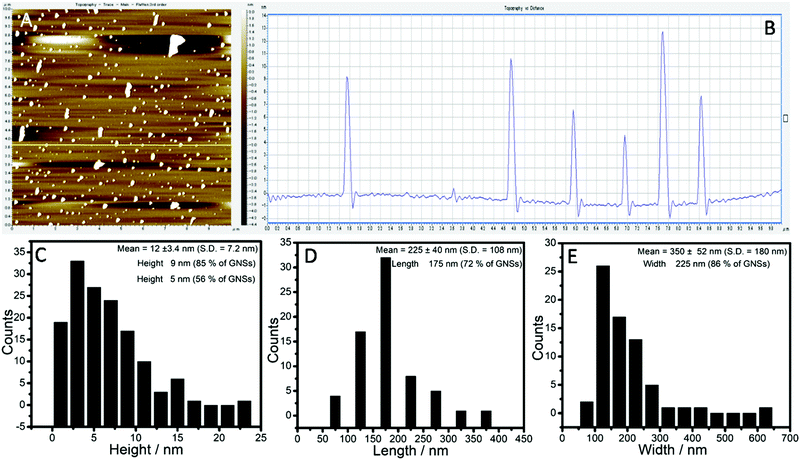 |
| Fig. 3 (A) AFM image and (B) surface topology of the obtained graphene-flakes by using the SAIL at a concentration of 1 mmol L−1; and (C)–(E) images showing statistical data obtained from analysis of the AFM images of the graphene-flakes: (C) height; (D) length; and (E) width. | |
This indicates that the distribution is not symmetrical around the mean and hence care must be taken in discussing the mean values. The distribution is centered around 3–5 nm, where ≈56% of flakes have a height <5 nm, whereas ≈85% graphene-flakes exhibit a thickness <9 nm. The obtained thickness of 3–5 nm corresponds to 7–12 layered graphene-flakes considering the interplanar spacing between two consecutive graphene planes as 0.4 nm.70 On similar lines, the distributions of both the length and width are not normal and are highly skewed with peaks centered at 175 and 135 nm, respectively. The amount of graphene-flakes with length ≤175 nm is ≈72% and flakes with width ≤225 nm is ≈86%.
The quality of the exfoliated graphene-flakes was ascertained via Raman spectroscopy measurements. The Raman spectrum of the dispersion (Fig. 4A) before sonication shows the presence of three bands i.e. the D-band at 1350 cm−1, G-band at 1582 cm−1 and 2D-band at 2723 cm−1 corresponding to graphite, whose intensity ratio changes for dispersions of graphene-flakes.54,56 A relatively larger D-band has been observed for the graphene-flakes as compared to that of the graphite powder. This indicates an increased fraction of defects in the graphene-flakes, which obviously appear after sonication and rotation during centrifugation.51,54,68 The intensity ratio, (ID/IG), of the D to the G band, a lower value of which is an indicator of high quality of the obtained graphene, comes out be 0.4 for the graphene-flakes.
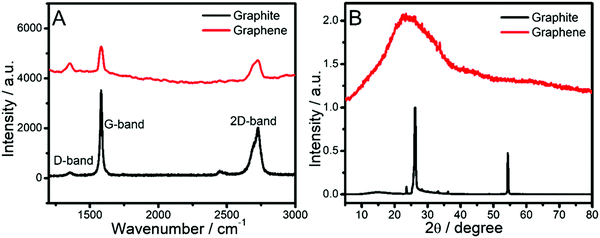 |
| Fig. 4 (A) Raman spectrum and (B) XRD pattern of graphite-flakes before and after exfoliation. | |
The calculated ID/IG value is closer to the reported range of 0.26–0.60 for graphene-flakes in colloidal systems of surfactants.51 A considerable difference in shape of the 2D band of the graphene-flakes as compared to graphite suggests that the graphene-flakes are comprised of about 5 layers of graphene,45 which is in good agreement with the AFM data. Further, the disappearance and broadening of X-ray diffraction peaks (Fig. 4B) around 54.35° (004) and 26.16° (002), respectively, for the graphene-flakes as compared to those present in the graphite powder support the SAIL assisted exfoliation of graphite-flakes in aqueous medium and are in line with literature reports.63
The role of the SAIL in exfoliation and providing stability to the obtained dispersions of graphene-flakes was monitored by ζ-potential and NMR spectroscopy measurements (Fig. 5). Positive ζ-potential values between 30 to 40 mV are observed for dispersions of graphene-flakes in the aqueous SAIL at varying concentrations, which is even higher than that observed for aqueous SAIL systems without graphene-flakes (Fig. 5A and B).
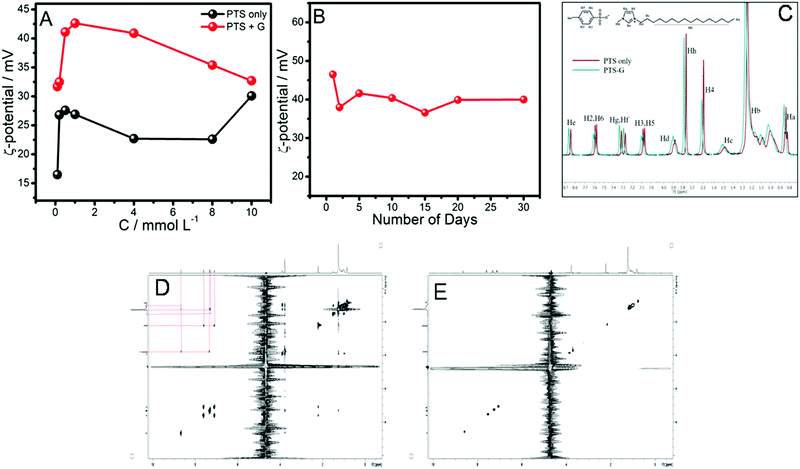 |
| Fig. 5 (A) Zeta-potential change as a function of SAIL concentration in aqueous solution without (PTS only) and with graphene-flakes (PTS + G); (B) zeta-potential of the dispersion of graphene-flakes in a 1 mmol L−1 concentration of the SAIL as a function of time; (C) 1H NMR spectra of an aqueous solution of a 1 mmol L−1 concentration of the SAIL without (PTS only) and with graphene-flakes (PTS-G); (D) 2D 1H–1H NOESY spectra of an aqueous solution of the SAIL in the presence of graphene-flakes; (E) 1H–1H 2D NOESY spectra of aqueous SAIL without graphene-flakes. | |
As such graphene has no zeta-potential, however, when coated with SAIL molecules, SAIL–graphene-flake conjugates acquire positive ζ-potential values, indicating the adsorption of the SAIL over the graphene-flakes, predominantly via hydrophobic interactions between the alkyl chain of the SAIL and surface of the graphene-flakes. The electrostatic repulsion between the SAIL ions adsorbed on the surface of the graphene-flakes provides stability to the exfoliated graphene-flakes against agglomeration. The role of such electrostatic repulsion in stabilizing aqueous dispersions of graphene-flakes is also reported in the literature.51 The observance of the highest ζ-potential at a SAIL concentration of 1 mmol L−1 is assigned to the greater extent of adsorption of the SAIL onto the graphene-flakes as compared to the other systems, which is in line with the maximum extent of exfoliation and dispersion stability in this case. A decrease in ζ-potential beyond a concentration of 1 mmol L−1 of the SAIL is suggestive of inter-micellar interactions, which reduces the stability of the dispersions. Further, the time-dependent ζ-potential measurements (Fig. 5B) establish the colloidal stability of the graphene-flake dispersion at least for 30 days. The utilized SAIL has been established to form micelles at a critical micelle concentation (cmc) of 0.23 mmol L−1,7 above which both monomers and micelles of the SAIL remain present in solution. The observed stability of the dispersion at a concentration (1 mmol L−1) higher than that of the cmc (0.23 mmol L−1) suggests the role of both monomers and micelles. It is obvious that with an increase in the concentration of the SAIL, the number of micelles, as per the law of mass action,71 increases, which could hinder the required interactions between the graphene-flakes and SAIL monomers. Further, an increase in the number of micelles would give rise to inter-micellar interactions, which along with a change in the hydration level of SAIL monomers could effect the efficiency of exfoliation. The dominating role of micelles in decreasing the extent of graphene-flake exfoliation is clear from the similarity in the ζ-potential values of aqueous solutions of the SAIL in the absence and presence of graphene-flakes at higher concentrations of the SAIL.
Further, to support our observation, 1H-NMR and 2D 1H–1H NOESY (Fig. 5C and E) experiments have been performed at a fixed concentration of the SAIL (1 mmol L−1) as the maximum exfoliation and dispersion stability were observed at this concentration of the SAIL. In general, the observed small downfield shift for different protons of the SAIL in the presence of graphene-flakes as compared to the aqueous SAIL infers their interaction with the graphene-flakes (Fig. 5C). This may be assigned to π–π interactions offered by the π-electron cloud of the graphene-flakes towards the protons of the SAIL. Further, the observed broadening of resonance peaks for the imidazolium ring protons of [C16mim]+ and aromatic ring protons of [PTS]− suggests their presence in a constrained environment, which indicates intercalation of SAIL ions between graphene-flakes. However, the interactions between the SAIL and graphene-flakes seem to be dominated by [C16mim]+ as compared to [PTS]− as suggested by positive ζ-potential values (Fig. 5A). The appearance of correlation peaks between cationic/anionic moieties of the SAIL in the presence of graphene-flakes (Fig. 5D), which don’t show up in the aqueous SAIL at the investigated concentration (Fig. 5E), clearly supports the adsorption of the SAIL on the graphene-flakes, which results in close proximity of ionic species, similar to that reported for surfactant systems.54,69
3.3. Hydrogelation of exfoliated graphene-flakes and rheological behavior of hydrogels
The information gained from the phase behavior of the SAIL in aqueous solution was employed to formulate SAIL–graphene-flake hydrogels. The aqueous solution of the SAIL with maximum exfoliated graphene-flakes (1 mmol L−1 of the SAIL; 1 mg mL−1 of graphene-flakes) was used for preparation of a supramolecular hydrogel by increasing the concentration of the SAIL up to 150 mmol L−1 (Fig. 6A).
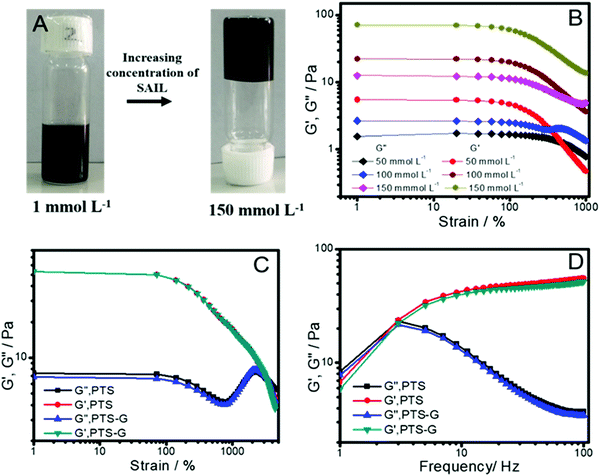 |
| Fig. 6 (A) Photograph showing formation of the SAIL–graphene-flake hybrid hydrogel; (B) variation of G′ (storage modulus) and G′′ (loss modulus) as a function of strain employing a frequency of 1 Hz; (C) strain-sweep measurements and (D) frequency-sweep measurements of the hydrogel without graphene-flakes (PTS) and with graphene-flakes (PTS-G). | |
The reason to choose this concentration of the SAIL lies in the fact that the hydrogel formed at the chosen concentration of the SAIL exhibits the largest value of the storage modulus (G′), an important parameter that displays the strength of a gel against mechanical stress, in strain-sweep measurements (Fig. 6B). The absence of any crossover between G′ and G′′ at higher concetration contrary to that observed at lower concentration (50 mmol L−1) indicates the dominance of G′ over G′′ across the entire deformation range and is assigned to the formation of viscoelastic gels. The prepared SAIL–graphene-flake hydrogel is found to exhibit an almost similar variation in G′ and G′′ under strain-sweep measurements (Fig. 6C). Further, the SAIL–graphene-flake hydrogel exhibits identical behavior to that of the SAIL hydrogel under frequency sweep measurements where an increase in G′ with a rise in frequency is observed (Fig. 6D).
Both these measurements establish that the incorporation of graphene-flakes into the hydrogel doesn’t affect the viscoelastic behavior and the tolerance of the hydrogel to external stress. This could only be possible when exfoliated SAIL decorated graphene-flakes remain entrapped in the voids of the hydrogel network formed by entanglement of worm like micelles. The SAIL–graphene-flake hydrogels exhibit a melting point of ≈40 °C, and the melted hydrosol was diluted with water to a final SAIL concentration of 1 mmol L−1, from which the graphene-flakes were obtained with no change in the ID/IG value in Raman spectra and characteristic peaks in X-ray diffraction (Fig. S4, ESI†) as compared to freshly exfoliated graphene-flakes (Fig. 4). In this manner, a new strategy for exfoliation of graphene-flakes from graphite in aqueous medium employing a low frequency bath sonicator at room temperature using a SAIL with long term stabilization (for at least 30 days) of the formed colloidal systems is achieved. The formulation of hydrogels of such SAIL–graphene-flake systems merely by changing the concentration of the SAIL provides an opportunity for extended stability of graphene-flakes against agglomeration and centrifugation by restricting the motion of graphene-flakes in the gel phase. The present work along with the recently reported studies on exfoliation of TDMs in aqueous medium using a polymer ionic liquid and subsequent hydrogelation62 would provide a new platform for extraction and storage of TDMs. The exfoliated graphene-flakes owing to the wide size distribution and thickness along with structural defects may not be suitable for electronic applications, however they may be utilized to prepare enzyme–graphene conjugates where an enhancement in the enzymatic activity is expected due to close association of the enzyme and substrate adsorbed on the graphene-flakes.
4. Conclusion
The phase behaviour of a surface active ionic liquid (SAIL) having an aromatic anion [PTS]− and imidazolium cation [C16mim]+ has been studied. A transition from rod-like micelles in aqueous solution to an interpenetrated network of elongated worm-like micelles is observed with increasing the concentration of the SAIL. The SAIL at lower concentrations is found to exhibit good efficiency towards exfoliation of graphene-flakes in aqueous medium with prolonged colloidal stability of at least one month against agglomeration. The exfoliated graphene-flakes are found to consist of 5–10 layers as suggested from AFM measurements. The intercalation of both [C16mim]+ and [PTS]− between the graphene-flakes is supposed to provide long-term colloidal stability. In this way, a new method for storage of exfoliated graphene-flakes in the form of a hydrogel, formed with the assistance of a SAIL, in the absence of any additive, is established. The stored graphene-flakes are found to remain stable without any agglomeration even against centrifugation and can be extracted from the hydrogel, when required.
Conflicts of interest
There are no conflicts to declare.
Acknowledgements
M. K. and G. S. are thankful to CSIR for SRF. DST is highly acknowledged for financial support with grant number EMR/2017/002656.
References
-
P. Wasserscheid and T. Welton, Ionic Liquids in Synthesis, Wiley, New York, 2003 Search PubMed.
- T. Welton, Chem. Rev., 1999, 99, 2071 CrossRef CAS PubMed.
- J. Dupont, R. F. De Souza and P. A. Z. Suarez, Chem. Rev., 2002, 102, 3667–3692 CrossRef CAS PubMed.
- J. Bowers, P. Butts, J. Martin, C. Vergara-Gutierrez and K. Heenan, Langmuir, 2004, 20, 2191–2198 CrossRef CAS PubMed.
- M. T. Garcia, I. Ribosa, L. Perez, A. Manresa and F. Comelles, Langmuir, 2013, 29, 2536–2545 CrossRef CAS PubMed.
- T. Singh and A. Kumar, J. Phys. Chem. B, 2007, 111, 7843–7851 CrossRef CAS PubMed.
- G. Singh, G. Singh and T. S. Kang, J. Phys. Chem. B, 2016, 120, 1092–1105 CrossRef CAS PubMed.
- R. Kamboj, P. Bharmoria, V. Chauhan, G. Singh, A. Kumar, S. Singh and T. S. Kang, Phys. Chem. Chem. Phys., 2014, 16, 26040–26050 RSC.
- G. Singh, M. Kaur, M. Drechsler and T. S. Kang, Chem. Commun., 2018, 54, 2432–2435 RSC.
- S. Mandal, J. Kuchlyan, S. Ghosh, C. Banerjee, N. Kundu, D. Banik and N. Sarkar, J. Phys. Chem. B, 2014, 118, 5913–5923 CrossRef CAS PubMed.
- B. Dong, N. Li, L. Zheng, L. Yu and T. Inoue, Langmuir, 2007, 23, 4178–4182 CrossRef CAS PubMed.
- H. Wang, J. Wang, S. Zhang and X. Xuan, J. Phys. Chem. B, 2008, 112, 16682–16689 CrossRef CAS PubMed.
- H. Wang, B. Tan, J. Wang, Z. Li and S. Zhang, Langmuir, 2014, 30, 3971–3978 CrossRef CAS PubMed.
- H. Wang, L. Zhang, J. Wang, Z. Lia and S. Zhang, Chem. Commun., 2013, 49, 5222–5224 RSC.
- N. Li, S. Zhang, L. Zheng, B. Dong, X. Li and L. Yu, Phys. Chem. Chem. Phys., 2008, 10, 4375–4377 RSC.
- Y. Bi, H. Wei, Q. Hu, W. Xu, Y. Gong and L. Yu, Langmuir, 2015, 31, 3789–3798 CrossRef CAS PubMed.
- P. Brown, C. Butts, R. Dyer, J. Eastoe, I. Grillo, F. Guittard, S. Rogers and R. Heenan, Langmuir, 2011, 27, 4563–4571 CrossRef CAS PubMed.
- P. Brown, C. P. Butts, J. Eastoe, D. Fermin, I. Grillo, H.-C. Lee, D. Parker, D. Plana and R. M. Richardson, Langmuir, 2012, 28, 2502–2509 CrossRef CAS PubMed.
- T. J. Trivedi, K. S. Rao, T. Singh, S. K. Mandal, N. Sutradhar, A. B. Panda and A. Kumar, ChemSusChem, 2011, 4, 604–608 CrossRef CAS PubMed.
- K. S. Rao, T. J. Trivedi and A. Kumar, J. Phys. Chem. B, 2012, 116, 14363–14374 CrossRef CAS PubMed.
- G. Singh, R. Kamboj, V. S. Mithu, V. Chauhan, T. Kaur, G. Kaur, S. Singh and T. S. Kang, J. Colloid Interface Sci., 2017, 496, 278–289 CrossRef CAS PubMed.
- R. Kamboj, P. Bharmoria, V. Chauhan, S. Singh, A. Kumar, V. S. Mithu and T. S. Kang, Langmuir, 2014, 30, 9920–9930 CrossRef CAS PubMed.
- S. Ghosh, C. Ghatak, C. Banerjee, S. Mandal, J. Kuchlyan and N. Sarkar, Langmuir, 2013, 29(32), 10066–10076 CrossRef CAS PubMed.
- C. Banerjee, S. Mandal, S. Ghosh, J. Kuchlyan, N. Kundu and N. Sarkar, J. Phys. Chem. B, 2013, 117, 3927–3934 CrossRef CAS PubMed.
- L. E. Buerkle, R. Galleguillos and S. J. Rowan, Soft Matter, 2011, 7, 6984–6990 RSC.
- N. Cheng, Q. Hu, Y. Bi, W. Xu, Y. Gong and Li. Yu, Langmuir, 2014, 30, 9076–9084 CrossRef CAS PubMed.
- F. D’Anna, P. Vitale, S. Marullo and R. Noto, Langmuir, 2012, 28, 10849–10859 CrossRef PubMed.
- M. Cai, Y. Liang, F. Zhou and W. Liua, J. Mater. Chem., 2011, 21, 13399–13405 RSC.
- Y. Lin, Y. Qiao, Y. Yan and J. Huang, Soft Matter, 2009, 5, 3047–3053 RSC.
- R. Oda, I. Huc and S. J. Candau, Angew. Chem., Int. Ed., 1998, 37, 2689–2691 CrossRef CAS PubMed.
- A. M. Brizard, M. C. A. Struartb and J. H. V. Esch, Faraday Discuss., 2009, 143, 345–357 RSC.
- L. E. Buerklea and S. J. Rowan, Chem. Soc. Rev., 2012, 41, 6089–6102 RSC.
- J.-H. Fuhrhop, S. Svenson, C. Boettcher, E. Rössler and H.-M. Veith, J. Am. Chem. Soc., 1990, 112, 4307–4312 CrossRef CAS.
- W. T. Truong, Y. Su, J. T. Meijer, P. Thordarson and F. Braet, Chem. – Asian J., 2011, 6, 30–42 CrossRef CAS PubMed.
- M. Rodrigues, A. C. Calpena, D. B. Amabilino, M. L. Garduño-Ramírez and L. Pérez-García, J. Mater. Chem. B, 2014, 2, 5419–5429 RSC.
- I. Yoshimura, Y. Miyahara, N. Kasagi, H. Yamane, A. Ojida and I. Hamachi, J. Am. Chem. Soc., 2004, 126, 12204–12205 CrossRef CAS PubMed.
- P. D. Wadhavane, M. A. Izquierdo, F. Galindo, M. I. Burguete and S. V. Luis, Soft Matter, 2012, 8, 4373–4381 RSC.
- C. Fan, L. Liao, C. Zhang and L. Liu, J. Mater. Chem. B, 2013, 1, 4251–4258 RSC.
- A. K. Geim and K. S. Novoselov, Nat. Mater., 2007, 6, 183–191 CrossRef CAS PubMed.
- M. Yi and Z. Shen, J. Mater. Chem. A, 2015, 3, 11700–11715 RSC.
- W. Luo, Y. Wang, E. Hitz, Y. Lin, B. Yang and L. Hu, Adv. Funct. Mater., 2017, 27, 1701450 CrossRef.
- Y. Zhu, S. Murali, W. Cai, X. Li, J. W. Suk, J. R. Potts and R. S. Ruoff, Adv. Mater., 2010, 22, 3906–3924 CrossRef CAS PubMed.
- X. Huang, Z. Yin, S. Wu, X. Qi, Q. He, Q. Zhang, Q. Yan, F. Boey and H. Zhang, Small, 2011, 7, 1876–1902 CrossRef CAS PubMed.
- X. T. Zheng, A. Ananthanarayanan, K. Q. Luo and P. Chen, Small, 2015, 11(14), 1620–1636 CrossRef CAS PubMed.
- Y. Hernandez, V. Nicolosi, M. Lotya, F. M. Blighe, Z. Sun, S. De, I. T. McGovern, B. Holland, M. Byrne, Y. K. Gun’ko, J. J. Boland, P. Niraj, G. Duesberg, S. Krishnamurthy, R. Goodhue, J. Hutchison, V. Scardaci, A. C. Ferrari and J. N. Coleman, Nat. Nanotechnol., 2008, 3, 563–568 CrossRef CAS PubMed.
- A. Reina, X. Jia, J. Ho, D. Nezich, H. Son, V. Bulovic, M. S. Dresselhaus and J. Kong, Nano Lett., 2009, 9(1), 30–35 CrossRef CAS PubMed.
- X. Li, C. W. Magnuson, A. Venugopal, J. An, J. W. Suk, B. Han, M. Borysiak, W. Cai, A. Velamakanni, Y. Zhu, L. Fu, E. M. Vogel, E. Voelkl, L. Colombo and R. S. Ruoff, Nano Lett., 2010, 10, 4328–4334 CrossRef CAS PubMed.
- Z. Chen, W. Ren, L. Gao, B. Liu, S. Pei and H.-M. Cheng, Nat. Mater., 2011, 10, 424–428 CrossRef CAS PubMed.
- M. J. McAllister, J.-L. Li, D. H. Adamson, H. C. Schniepp, A. A. Abdala, J. Liu, M. Herrera-Alonso, D. L. Milius, R. Car, R. K. Prud’homme and I. A. Ahsay, Chem. Mater., 2007, 19, 4396–4404 CrossRef CAS.
- S. Haar, M. S. Gemayel, Y. Shin, G. Melinte, M. A. Squillaci, O. Ersen, C. Casiraghi, A. Ciesielski and P. Samorí, Sci. Rep., 2015, 5, 16684–16693 CrossRef CAS PubMed.
- A. Ciesielski and P. Samorí, Chem. Soc. Rev., 2014, 43, 381–398 RSC.
- H. Wang, B. Xia, Y. Yan, N. Li, J.-Y. Wang and X. Wang, J. Phys. Chem. B, 2013, 117, 5606–5613 CrossRef CAS PubMed.
- S. Ahadian, M. Estile, V. J. Surya, J. Ramón-Azcón, X. Liang, H. Shiku, M. Ramalingam, T. Matsue, Y. Sakka, H. Bae, K. Nakajami, Y. Kawazoec and A. Khademhosseini, Nanoscale, 2015, 7, 6436–6443 RSC.
- M. Lotya, Y. Hernandez, P. J. King, R. J. Smith, V. Nicolosi, L. S. Karlsson, F. M. Blighe, S. De, Z. Wang, I. T. McGovern, G. S. Duesberg and J. N. Coleman, J. Am. Chem. Soc., 2009, 131, 3611–3620 CrossRef CAS PubMed.
- H. Chang, G. Wang, A. Yang, X. Tao, X. Liu, Y. Shen and Z. Zheng, Adv. Funct. Mater., 2010, 20, 2893–2902 CrossRef CAS.
- M. Lotya, P. J. King, U. Khan, S. De and J. N. Coleman, ACS Nano, 2010, 4, 3155–3162 CrossRef CAS PubMed.
- R. J. Smith, P. J. King, M. Lotya, C. Wirtz, U. Khan, S. De, A. O’Neill, G. S. Duesberg, J. C. Grunlan, G. Moriarty, J. Chen, J. Wang, A. I. Minett, V. Nicolosi and J. N. Coleman, Adv. Mater., 2011, 23, 3944–3948 CrossRef CAS PubMed.
- C. V. Subbarao, I. P. K. Chakravarthy, A. V. S. L. Sai Bharadwaj and K. M. M. K. Prasad, Chem. Eng. Technol., 2012, 2, 225–237 CrossRef.
- X. Wang, P. F. Fulvio, G. A. Baker, G. M. Veith, R. R. Unocic, S. M. Mahurin, M. Chi and S. Dai, Chem. Commun., 2010, 46, 4487–4489 RSC.
- X. Zhou, T. Wu, K. Ding, B. Hu, M. Hou and B. Han, Chem. Commun., 2010, 46, 386–388 RSC.
- S. Ravula, S. N. Baker, G. Kamath and G. A. Baker, Nanoscale, 2015, 7, 4338–4353 RSC.
- T. Kim, H. Lee, J. Kim and K. S. Suh, ACS Nano, 2010, 4, 1612–1618 CrossRef CAS PubMed.
- X. Wang and P. Wu, ACS Appl. Mater. Interfaces, 2018, 10, 2504–2514 CrossRef CAS PubMed.
- B. Dong, J. Zhang, L. Zheng, S. Wang, X. Li and T. Inoue, J. Colloid Interface Sci., 2008, 319, 338–343 CrossRef CAS PubMed.
- W. Jiang and J. Hao, Langmuir, 2008, 24, 3150–3156 CrossRef CAS PubMed.
- P. A. Hassan, G. Fritz and E. W. Kaler, J. Colloid Interface Sci., 2003, 257, 154–162 CrossRef CAS PubMed.
- J. Li, M. Zhao and L. Zheng, Colloids Surf., A, 2012, 396, 16–21 CrossRef CAS.
- J. I. Paredes and S. Villar-Rodila, Nanoscale, 2016, 8, 15389–15413 RSC.
- S. Wang, M. Yi and Z. Shen, RSC Adv., 2016, 6, 56705–56710 RSC.
- F. Bonaccorso, A. Lombardo, T. Hasan, Z. Sun, L. Colombo and A. C. Ferrari, Mater. Today, 2012, 15, 564–589 CrossRef CAS.
- A. I. Rusanov, Colloid J., 2016, 78, 669–673 CrossRef CAS.
Footnote |
† Electronic supplementary information (ESI) available. See DOI: 10.1039/c9cp04449c |
|
This journal is © the Owner Societies 2020 |