DOI:
10.1039/C9AN01847F
(Paper)
Analyst, 2020,
145, 206-212
A rapid label- and enzyme-free G-quadruplex-based fluorescence strategy for highly-sensitive detection of HIV DNA†
Received
18th September 2019
, Accepted 28th October 2019
First published on 7th November 2019
Abstract
Because rapid, convenient, and selective methods for HIV detection are urgently needed, herein, a simple label-free and enzyme-free strategy is constructed for sensitive fluorescence detection of HIV DNA using the fluorescent intercalating dye thioflavin T (THT) as the detection signal source. This strategy utilizes a hairpin DNA sequence (H1) and two assistant strands. H1 is wisely designed with a G-quadruplex sequence in the stem. Target DNA, when present in solution, will hybridize with H1 to form H1/target duplexes and release the G-quadruplexes. Additionally, the assistant probes hybridize with the unfolded H1 to form a stable DNA double strand, resulting in the displacement of the target to participate in another similar reaction cycle. Consequently, many G-quadruplex structures are generated, leading to a significantly amplified fluorescence signal of THT. The linear range is from 0.1 nM to 50.0 nM with a limit of detection of 13 pM. Results can be achieved within 40 min, because the cyclic amplification involves only one DNA hairpin and two auxiliary chains. Furthermore, this platform exhibited good selectivity with one base mismatch or other DNA sequences. This strategy could be used as a simple, sensitive, and selective tool to detect other DNA biomarkers.
Introduction
According to recent statistics, approximately 36.9 million people are infected with HIV worldwide1. At present, the diagnosis of AIDS is mainly aimed at the detection of AIDS antibodies or antigens.2,3 Although these methods have good specificity, their sensitivity is unsatisfactory. Moreover, the low level of HIV antibodies in the initial window period also presents a great challenge to the early diagnosis of HIV.4 Therefore, it is urgent to develop a convenient, rapid, and sensitive method for the early diagnosis of HIV infection. Cell-free oligonucleotides in biofluids such as serum are alternative biomarkers. Rapid, quantitative, and sensitive detection of viral oligonucleotides can help diagnose the infection before symptoms occur, monitor disease progression, and identify viral subtypes and clades.5,6
In the past few years, many sensors have been constructed for HIV DNA detection, including colorimetric,7,8 fluorescent,9,10 electrochemical,11,12 and surface-enhanced Raman scattering.13,14 Among these biosensing assays, fluorescence-based methods hold promise because of the inherent merits of high sensitivity and simplicity, low sample volume, and quick response.15–17 However, the chemical modification of DNA strands by dyes is complex and expensive, and the fluorescent materials like quantum dots are also complex and time-consuming to synthesize, which limits the practical application of these probes.18 Thus, in view of the current limitations of HIV probes, we sought to design a G-quadruplex–THT-based fluorescent probe combined with our improved amplification strategy for highly sensitive detection of HIV DNAs.
Thioflavin T (THT), a label-free G-quadruplex fluorescent intercalating dye, exerts a double effect of shifting and amplifying, whereby it induces G-rich DNA to form a G-quadruplex configuration that subsequently produces significantly enhanced fluorescence signals.19 Compared with other DNA insertion dyes, such as thiazole orange, which can interact with other DNA forms including single-stranded or double-stranded DNA, THT has good specificity and interacts only with G-quadruplexes, and thus, it can be used for label-free specific detection.20,21 For example, Ling's group achieved highly specific detection of ssDNA using the G-quadruplex/THT structure,22 and a label-free and sensitive fluorescent HIV DNA detection platform using the G-quadruplex/THT structure by Zhang's group was also reported,23 but most of these methods require further improvement in sensitivity. In order to increase the sensitivity of fluorescent bioprobes, various signal amplification strategies are used, such as rolling circle amplification (RCA),24,25 catalytic hairpin assembly (CHA),26 hybridization chain reaction (HCR),27 polymerase chain reaction (PCR)28 and loop-mediated isothermal amplification (LAMP)29. Recently, there has also been a DNA walker strategy that amplifies signals using DNA enzymes or strand substitution combined with enzymes.30,31 Enzymes are used in many literature reports for amplification because of their high efficiency,32 but enzyme-assisted methods are often quite complex and expensive. CHA and HCR require at least two different hairpin DNA probes with long stems, which hinders the reaction kinetics and results in a time-consuming process. Therefore, an improved amplification method that can reduce the reaction kinetics hindrance is desirable.
Herein, we report a label-free, enzyme-free, and sensitive THT-based strategy in combination with a continuous amplification cycle for DNA detection. As a proof-of-concept, HIV DNA, which is one of the major causes of death in HIV-infected patients,33 was chosen as the model in this study. In this sensing platform, THT was selected as the detection signal source. The fluorescence from free THT is weak, but the fluorescence increases when THT combines with a G-quadruplex. The sensing system contains H1 and two single-stranded helper probes (helper A and helper B). The hairpin structure of H1 can be opened by HIV DNA and the strand displacement amplification can be promoted by helper probes. Moreover, due to the problem of slow reaction kinetics of the current enzyme-free amplification strategy, two auxiliary chains are used instead of a second hairpin, which not only ensures a low fluorescence background, but also promotes the amplification cycle of the entire system, and the problem of opening of multiple hairpins hindering the reaction kinetics can be avoided. Through this continuous amplification cycle, large quantities of G-quadruplex structures are produced, leading to a significant enhancement of fluorescence after association with THT. Thus, highly sensitive detection of targets can be achieved by monitoring the change in the fluorescence signal. The total reaction was carried out in a uniform solution without a complicated reaction process or the time-consuming cyclic reaction. The detection time is only 40 min, because cyclic amplification involves only one DNA hairpin and two auxiliary chains. Moreover, this detection platform could potentially be used in applications that detect other specific targets.
Experimental
Chemicals and materials
HIV DNA, H1, assistant probes (helper A and helper B), 1× Tris-EDTA buffer (1× TE) (10.0 mM Tris(hydroxymethyl)aminomethane-HCl (Tris-HCl), 1 mM ethylenediaminetetraacetic acid (EDTA)), 50× TE (2.0 mM Tris-HCl; 100.0 mM EDTA) and 10× Tris-MgSO4 buffer (10× TM) (500.0 mM Tris-HCl, 80.0 mM MgSO4) were ordered from Shanghai Sangon Co., Ltd (Shanghai, China). The sequences of the used oligonucleotides are listed in Table 1. Thioflavin T was purchased from J&K Scientific Ltd (Beijing, China). All oligonucleotides were HPLC-purified and freeze-dried by the supplier. Milli-Q ultrapure water (18.2 MΩ cm) was used for all experiments. Serum samples were obtained from healthy humans in Xiangtan University Hospital.
Table 1 Sequences of the used oligonucleotidesa
Oligonucleotides |
Sequence (5′-3′) |
The underlined bases represent the mismatched positions; the bold bases in H1 is the complete complementary sequence of target HIV DNA, and the italic part in H1 is the complete complementary part of A13 and B14.
|
HIV DNA |
TGTGTGAGGGCTGGGCCTTGG |
H1 |
TACCCTACCCAAGGCCCAGCCCTCACACAACTGATTGGGTAGGGTAGGGTAGGG |
Helper A(13) (A13) |
CTGGGCCTTGGGT |
Helper A(14) (A14) |
CTGGGCCTTGGGTA |
Helper A(15) (A15) |
CTGGGCCTTGGGTAG |
Helper B(12) (B12) |
GTTGTGTGAGGG |
Helper B(13) (B13) |
AGTTGTGTGAGGG |
Helper B(14) (B14) |
CAGTTGTGTGAGGG |
Single-base mismatch target (SM) |
TGTGT AGGGCTGGGCCTTGG |
Two-base mismatch target (DM) |
TGTGT AGGGCTGGG CTTGG |
Three-base mismatch target (TM) |
TGTGT AGGGCTG G CTTGG |
Four-base mismatch target (FM) |
TGTGT AGGGCT GG CTT G |
Noncomplementary target (NM) |
GGTTCCGTGTCGGGAGTGTAT |
All experiments were performed in accordance with the Guidelines of the Chinese Council on Animal Care and approved by the Ethics Committee of Xiangtan University. Informed consent was obtained from human participants of this study.
Instrumentation
The fluorescence spectra were obtained on a RF-5301PC spectrofluorometer (Shimadzu, Japan) using a quartz cuvette (1.0 cm × 1.0 cm). The spectrum of the sample was recorded in the wavelength range of 460–530 nm with excitation and emission wavelengths of 425 nm and 490 nm, respectively. A pHs-3C digital pH meter (Leici, Shanghai, China) was used to detect the pH values.
Fluorescence analysis procedure
All oligonucleotides were dissolved in 1× TE buffer to obtain stock solutions of 10.0 μM. Before experiments, H1 was annealed at 95 °C for 10 min followed by slowly decreasing the temperature to room temperature. The mixture included 50.0 nM H1, 25.0 nM helper A, 25.0 nM helper B, 50.0 mM K+, 2.0 μM THT, and a series of different concentrations of the target, and was incubated at 37 °C for 40 min. The reaction mixture was diluted with 70 μL 10× TM buffer and Milli-Q ultrapure water to a final volume of 250 μL, and the final concentrations of the target DNA varied from 0.1 nM to 50.0 nM. The fluorescence intensity was measured using an RF-5301PC spectrofluorometer.
Circular dichroism (CD) analysis
The circular dichroism (CD) spectra of the samples were obtained using a Chirascan spectrometer (Chirascan, Applied Photophysics, UK) at room temperature. First, 2.0 μM H1, 2.0 μM helper A, and 2.0 μM B were reacted in 10× TM buffer. The target (500.0 nM) was added to the entire reaction system. The CD spectrum was recorded in the region of 220–290 nm using a 1 mm path length quartz cuvette. The scanning mode of the instrument was set with the following parameters: scanning speed, 100 nm min−1; response time, 0.5 s; bandwidth, 1.0 nm. Each sample was scanned three times, and the spectra were averaged from three measurements.
Polyacrylamide gel electrophoresis (PAGE) analysis
12% native polyacrylamide gel was prepared using 5× TBE buffer. All of the DNA samples were incubated at 37 °C for 40 min. The loading samples were prepared by mixing 10 μL DNA samples, 2 μL 6× loading buffer and allowed to stand for 3 min before being injected into polyacrylamide gel. Gel electrophoresis was run at 100 V for 60 min in 1× TBE buffer and stained with Stain-All and then photographed after decolorization.
Tests using human serum samples
In order to certify the potential application of the designed method for complex samples, dilute serum from healthy humans was used as a model, and recovery experiments were carried out using the proposed strategy. Blood samples were provided by the Hospital of Xiangtan University, and then serum was extracted. After the serum was centrifuged, the supernatant was collected and diluted 400 times with working buffer. Various concentrations of the target were added into the diluted samples, and three samples at different concentrations were prepared and tested in parallel. The relative standard deviation and recovery were calculated according to the experimental results.
Results and discussion
Principle of the sensing platform
Herein, a label-free, enzyme-free fluorescence sensing method is developed that uses a chain displacement signal amplification strategy to detect HIV DNA. The detection principle is shown in Scheme 1. The sensing system contains DNA hairpin H1 and helper probes A and B, and the nucleic acid dye THT. H1 is composed of a sequence that is complementary to the target and also a G-rich sequence. The G-rich sequence is initially locked in the stem of H1 to inhibit the formation of the G-quadruplex structure. When the target is added to the solution, it hybridizes with H1 and unfolds the hairpin structure, and the G-rich sequence is released. Then, the auxiliary chain hybridizes with H1, and the target is displaced by a chain replacement reaction, accompanied by the formation of stable DNA double strands. The displaced target DNA is free to further associate with another H1, and consequently, massive amounts of G-quadruplexes are produced into which THT can intercalate, leading to a significantly enhanced fluorescence signal of THT for sensitive detection of the target.
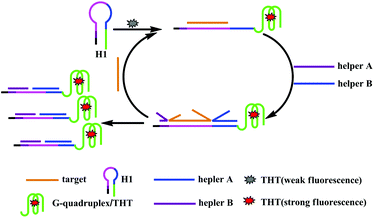 |
| Scheme 1 Schematic illustration of the label-free and enzyme-free fluorescence amplification strategy for HIV DNA detection. | |
The viability of the design
To verify the applicability of the proposed method, fluorescence experiments using the H1 probe in the absence/presence of the target and with/without the addition of helper A and B were performed, with the addition of THT in all four cases. Once the G-quadruplex is formed, the fluorescence will be enhanced. The fluorescence emission spectra are shown in Fig. 1. The system containing only H1 (curve a) exhibited very weak fluorescence emission, because the G-rich sequence was locked in the stem of H1, and this prevented the production of G-quadruplexes. Then, the fluorescence in the presence of H1 and two auxiliary chains was measured, and the fluorescence signal was found to be slightly higher than that in the presence of H1 only. This difference in the signal might be due to the formation of a small number of G-quadruplexes by hybridization of the auxiliary probes with H1, but the difference in the fluorescence intensity between the two cases was very small, indicating that the efficiency of opening the H1 hairpin by the two auxiliary chains is very low, and the influence of the auxiliary chains on the experiment can be neglected. However, the fluorescence emission of H1 was clearly enhanced when the target was added to the solution, because the hairpin structure of H1 was opened after the target hybridized with H1 and G-quadruplexes were formed. Under the action of THT, the G-quadruplex-THT complex was subsequently formed, and the fluorescence was enhanced. Furthermore, it can be seen from the figure that the fluorescence enhancement efficiency of the cyclic amplification strategy with helper A and helper B is significantly higher than that without their use, which indicates that helper A and helper B can effectively liberate the target from the target/H1 double chain, promote the generation of a large number of G-quadruplexes, and significantly enhance the fluorescence response. Experiments show that the proposed method can be used for target detection.
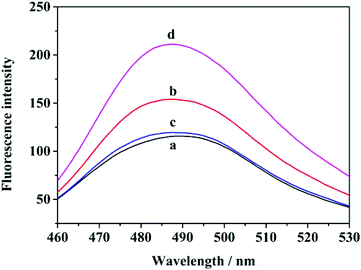 |
| Fig. 1 Typical fluorescence emission spectra of different samples. (a) H1 + THT; (b) H1 + target + THT; (c) H1 + A + B + THT; (d) H1 + A + B + target + THT. | |
CD assay
CD spectra were obtained for different samples to verify whether the G-quadruplex structures were formed (Fig. 2). When the sample consisted of only H1, there were weak positive peaks at about 270 nm and weak negative peaks at about 240 nm, and these peaks are characteristic of parallel G-quadruplexes.34–36 After the target was added to H1, the characteristic peak signal slightly increased due to the generation of H1/target duplexes and the release of the G-quadruplex structures. The peaks of sample H1 and helpers A and B at the same position are observed. Upon incubation of the target with H1, helper A and helper B, a significant increase in the intensity of CD peaks was observed, which indicated that more G-quadruplex structures were generated through strand displacement. These CD results were consistent with fluorescence experiments, further verifying the practicability of this amplification method for the DNA assay.
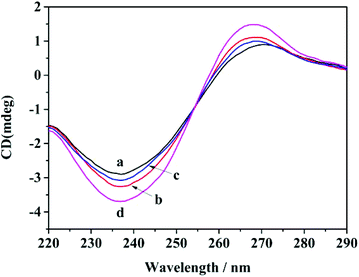 |
| Fig. 2 CD spectra of curves (a) H1, (b) H1 + target, (c) H1 + A + B, and (d) H1 + A + B + target. | |
PAGE analysis
The success of DNA hybridization was validated by PAGE. As shown in Fig. S1,† lanes 1–5 are H1, helperA + helper B, target, H1 + helperA + helper B + target, and H1 + target, respectively. The hybrid products of the target and hairpin H1 (lane 5) showed a band with much lower mobility than hairpin H1 and target (lanes 1 and 3), suggesting that H1 was successfully opened by the target and double-chain products were obtained. The mixtures with H1, target and auxiliary chains (lane 4) show a slightly lower mobility band than mixtures with H1 and target only (lane 5), suggesting that the displacement reaction was successful.
Length effect of the auxiliary DNA probe
The method strongly relies on the ability of the auxiliary DNA probes to quickly and effectively replace the target DNA, and the best performance of the sensor can be obtained by varying the length of the auxiliary DNA probes A and B. The base sequences of auxiliary chains with different lengths are listed in Table 1. Fig. 3A shows the fluorescence spectra of solutions including 50.0 nM H1, and 25.0 nM probes A and B with and without the addition of 50.0 nM target when the lengths of A and B were varied from 13 to 15 nt and 12 to 14 nt, respectively. Fig. 3B shows the change in F/F0 (F0 and F denote the fluorescence signals in the absence and in the presence of the target DNA, respectively), and the most optimal performance was obtained when the lengths of helper A and helper B were 13 nt and 14 nt, respectively. Therefore, the detection feasibility, sensitivity, and selectivity of the experiments were assessed by employing a combination of helper A13 and helper B14.
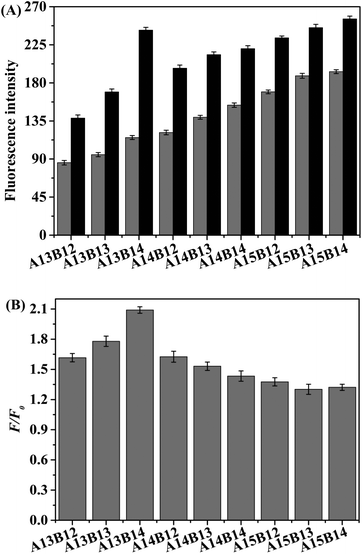 |
| Fig. 3 The effects of the length of helper DNA probes on signal generation. (A) Fluorescence intensities of sensing systems when challenged with helper probes of different lengths before and after the addition of 50 nM target DNA for 60 min. (B) Dependence of the F/F0 on the length of helper DNA probes. The sensing samples contained 50 nM H1, 25 nM helper A and 25 nM helper B. | |
Optimization of the reaction conditions
To obtain the optimal sensing performance of the fluorescence platform for target detection, the following experimental conditions were optimized: (a) concentrations of helpers A and B; (b) concentration of K+; (c) concentration of THT; (d) reaction temperature; (e) hybridization time. The corresponding data and figures are shown in the ESI (Fig. S2–S6†). In short, the following experimental conditions were found to give the best results: (a) concentrations of helpers A and B: 25.0 nM; (b) concentration of K+: 50.0 mM; (c) concentration of THT: 2.0 μM; (d) reaction temperature: 37 °C; (e) hybridization time: 40 min.
Performance of the HIV DNA assay
To validate the sensitivity of the proposed sensing assay, different concentrations of target were added to a mixture of H1 and the helper probes under optimal experimental conditions. Fig. 4 shows that the fluorescence intensity increases with the increase in the target concentration. The amount of G-quadruplexes/THT increased with the increase in the target concentration, leading to the corresponding enhancement of fluorescence signals at 490 nm. As illustrated in Fig. 4, the value of F − F0 was enhanced when the concentration of the target was increased from 100.0 pM to 50.0 nM. The correlation equation of the curve is F – F0 = 1.516C + 16.38 (R2 = 0.9939), where F and F0 denote the fluorescence intensity with and without the addition of the target, respectively, and C denotes the target concentration. It was calculated that the detection limit is as low as 13 pM, based on the 3σ/slope, where σ is the standard deviation of the blank measurements (n = 11). These results are comparable to most of the previous analyses shown in Table 2, demonstrating that this method offers an alternative to target assays with the merits of being sensitive, economical, and label-free.
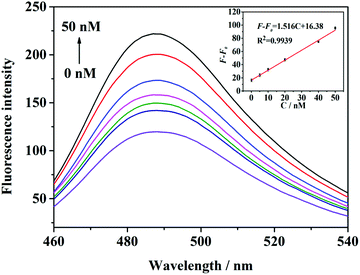 |
| Fig. 4 Fluorescence spectra of the sensing system in the presence of different concentrations of HIV DNA (0, 0.1, 5, 10, 20, 40, and 50 nM). Inset: Linear relationship of fluorescence intensity versus target concentration. The error bar represents the standard deviation of three measurements. | |
Table 2 Comparison of different methods for HIV DNA detection
Methods |
Linear range |
LOD |
Number of tags |
Amplification strategy |
Respond time |
Ref. |
Fluorescence |
50 pM–1 nM |
100 pM |
1 |
Nt.·BbvCI assisted |
60 min |
3
|
Electrochemical |
0.1fM–0.1 μM |
30 aM |
1 |
Exo-III assisted |
110 min |
7
|
Fluorescence |
1 nM–50 nM |
0.4 nM |
0 |
No |
50 min |
9
|
Fluorescence |
0.1–200 nM |
65 pM |
2 |
Nt.·BbvCI assisted |
30 min |
10
|
Colorimetric |
2.5–100 pM |
2.5 pM |
0 |
Exo-III assisted |
90 min |
11
|
Fluorescence |
5–200 nM |
2.4 nM |
0 |
No |
100 min |
23
|
Fluorescence |
1.0–500 nM |
0.5 nM |
0 |
No |
81 min |
37
|
Fluorescence |
10–200 pM |
3.5 pM |
1 |
Exo-III assisted |
>80 min |
38
|
Fluorescence |
0.1–50 nM |
13 pM |
0 |
Enzyme-free |
40 min |
This work |
Selectivity of the platform
The selectivity of the proposed fluorescence platform was further investigated by using five DNA sequences, including one base mismatch, two base mismatches, three base mismatches, four base mismatches, and completely mismatched DNA, and the five DNA sequences are shown in Table 1. As shown in Fig. 5, only the sample with one base mismatch elicited an obvious increase in fluorescence after the reaction with the target. However, the fluorescence response was negligible in the presence of the other mismatched DNA sequences. These results confirmed that the proposed target DNA detection strategy is highly selective and can distinguish well-matched DNA targets from mismatched sequences even if there is only one base mismatch. The specificity of this method is mainly due to the specific recognition of the target by molecular beacons.39 There is a dynamic competitive balance between the hybrid stem and the hybridization of the target and ring, and only perfectly complementary targets elicit a response. When the target contains a mismatched nucleotide or a deletion, the interaction between the hairpin and target is weaker than that of the intramolecular hybridization of hairpin. Thus, the hairpin structure of the molecular beacon cannot be destroyed and the fluorescence is not significantly enhanced.
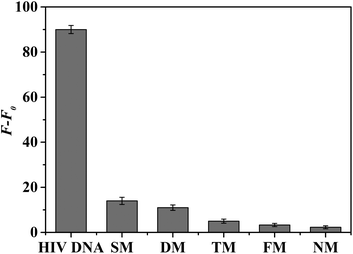 |
| Fig. 5 Selectivity investigation of the proposed strategy for HIV DNA detection based on fluorescence signals for the HIV DNA against single-base mismatch (SM), double-base mismatch (DM), three-base mismatch (TM), four-base mismatch (FM), and non-complementary (NM) DNA sequences. | |
The application of the platform in real sample analysis
To determine the potential application value of the designed method when complex samples are used, diluted serum from healthy humans was used as a model, and recovery experiments were carried out using the proposed strategy. The analytical results are shown in Table 3, which illustrates that satisfactory recoveries using this method were in the range of 90.00–99.00%, and the RSD value is between 1.3 and 3.5%, indicating that the method has certain potential for practical use with real samples.
Table 3 Results for the determination of HIV DNA in human serum
Sample (nM) |
Target DNA added (nM) |
Target DNA found (nM) |
Recovery (%) |
RSD (n = 3, %) |
1 |
0.20 |
0.18 |
90.00 |
3.5 |
2 |
2.00 |
1.98 |
99.00 |
2.3 |
3 |
20.00 |
19.25 |
96.25 |
1.3 |
Conclusions
In conclusion, we propose a simple and sensitive strategy for amplification detection of HIV DNA based on G-quadruplexes/THT in combination with a chain replacement signal amplification method. The strategy performed well for the detection of HIV DNA, with a limit of detection as low as 13 pM. The total reaction was carried out in a uniform solution without a complicated reaction process or time-consuming cyclic reactions. Furthermore, this method also exhibited excellent selectivity with the capability of discriminating single-base mismatches and other mismatched DNA sequences. Thus, the sensitive and selective strategy has potential applications in detecting other specific targets.
Conflicts of interest
There are no conflicts of interest to declare.
Acknowledgements
This work was supported by the National Natural Science Foundation of China (No. 21775132), the National Natural Science Foundation of Hunan province (No. 2018JJ2388), and the Hunan 2011 Collaborative Innovation Center of Chemical Engineering & Technology with Environmental Benignity and Effective Resource Utilization, and “1515” academic leader team program of Hunan Agricultural University.
Notes and references
- M. F. Fatin, A. R. Ruslinda, M. K. Md Arshad, K. K. Tee, R. M. Ayub, U. Hashim, A. Kamarulzaman and S. C. B. Gopinatha, Biosens. Bioelectron., 2016, 78, 358–366 CrossRef CAS.
- L. Wang, J. Tian, Y. Huang, X. Lin, W. Yang, Y. Zhao and S. Zhao, Microchim. Acta, 2016, 183, 2147–2153 CrossRef CAS.
- Y. Ma, X. Shen, Q. Zeng, H. Wang and L. Wang, Talanta, 2017, 164, 121–127 CrossRef CAS.
- T. N. Ly, S. Park and S. J. Park, Sens. Actuators, B, 2016, 237, 452–458 CrossRef CAS.
- H. Schwarzenbach, D. S. B. Hoon and K. Pantel, Nat. Rev. Cancer, 2011, 11, 426–437 CrossRef CAS.
- K. Zen and C. Zhang, Med. Res. Rev., 2012, 32, 326–348 CrossRef PubMed.
- W. Zhou, X. Gong, Y. Xiang, R. Yuan and Y. Chai, Biosens. Bioelectron., 2014, 55, 220–224 CrossRef CAS.
- X. Ma and P. Miao, J. Mater. Chem. B, 2019, 16, 1–6 Search PubMed.
- Y. D. Ye, L. Xia, D. D. Xu, X. J. Xing, D. W. Pang and H. W. Tang, Biosens. Bioelectron., 2016, 85, 837–843 CrossRef CAS.
- H. Zhu, M. Zhang, L. Zou, R. Li and L. Ling, Talanta, 2017, 173, 9–13 CrossRef CAS.
- Y. Wang, X. Bai, W. Wen, X. Zhang and S. Wang, ACS Appl. Mater. Interfaces, 2015, 7, 18872–18879 CrossRef CAS PubMed.
- D. Zhang, Y. Peng, H. Qi, Q. Gao and C. Zhang, Biosens. Bioelectron., 2009, 25, 1088–1094 CrossRef.
- Y. Liang, J. L. Gong, Y. Huang, Y. Zheng, J. H. Jiang, G. L. Shen and R. Q. Yu, Talanta, 2007, 72, 443–449 CrossRef CAS.
- J. Hu, P. C. Zheng, J. H. Jiang, G. L. Shen, R. Q. Yu and G. K. Liu, Analyst, 2010, 135, 1084–1089 RSC.
- A. H. Loo, Z. Sofer, D. Bousa, P. Ulbrich, A. Bonanni and M. Pumera, ACS Appl. Mater. Interfaces, 2016, 8, 1951–1957 CrossRef CAS.
- R. Liao, K. He, C. Chen, X. Chen and C. Cai, Anal. Chem., 2016, 88, 4254–4258 CrossRef CAS.
- L. Chen, L. Song, Y. Zhang, P. Wang, Z. Xiao, Y. Guo and F. Cao, ACS Appl. Mater. Interfaces, 2016, 8, 11255–11261 CrossRef CAS.
- P. Chen, P. Hu, K. Huang, E. Sawyer, K. Sun, B. Ying, X. Wei and J. Geng, RSC Adv., 2018, 8, 40564 RSC.
- X. H. Tan, Y. Wang, B. A. Armitage and M. P. Bruchez, Anal. Chem., 2014, 86, 10864–10869 CrossRef CAS.
- A. R. Faverie, A. Guedin, A. Bedrat, L. A. Yatsunyk and J.-L. Mergny, Nucleic Acids Res., 2014, 42, 65 CrossRef.
- J. Mohanty, N. Barooah, V. Dhamodharan, S. Harikrishna, P. I. Pradeepkumar and A. C. Bhasikuttan, J. Am. Chem. Soc., 2012, 135, 367–376 CrossRef.
- Y. Ling, X. Zhang, X. Chen, L. Liu, X. Wang, D. Wang, N. Li and H. Luo, New J. Chem., 2018, 42, 4714–4718 RSC.
- X. F. Zhang, H. M. Xu, L. Han, N. B. Li and H. Q. Luo, Anal. Sci., 2018, 34, 149–153 CrossRef CAS.
- Q. Xue, Y. Kong and H. Wang, Chem. Commun., 2017, 53, 10772–10775 RSC.
- W. Yang, Y. Shen, D. Zhang and W. Xu, Chem. Commun., 2018, 54, 10195–10198 RSC.
- J. Zhao, P. Jing, S. Xue and W. Xu, Biosens. Bioelectron., 2017, 87, 157–163 CrossRef CAS.
- W. Song, K. Zhu, Z. Cao, C. Lau and J. Lu, Analyst, 2012, 137, 1396–1401 RSC.
- P. Mestdagh, T. Feys, N. Bernard, S. Guerther, C. Chen, F. Speleman and J. Vandesompele, Nucleic Acids Res., 2008, 36, 143 CrossRef.
- M. Varona and J. L. Anderson, Anal. Chem., 2019, 91, 6991–6995 CrossRef CAS.
- X. Hua, E. Yang, D. Zhang, Y. Shen, J. Zhao and W. Xu, Sens. Actuators, B, 2019, 281, 864–870 CrossRef CAS.
- K. Yang, H. Wang, N. Ma, M. Zeng, H. Luo and D. He, ACS Appl. Mater. Interfaces, 2018, 10, 44546–44553 CrossRef CAS.
- P. Chen, K. Huang, P. Zhang, E. Sawyer, Z. Wu, X. Wei, B. Ying and J. Geng, Talanta, 2019, 203, 255–260 CrossRef CAS.
- K.-B. Lee, E.-Y. Kim, C.-A. Mirkin and S.-M. Wolinsky, Nano Lett., 2004, 4, 1869–1872 CrossRef CAS.
- X. Li, L. Gan, Q. Ou, X. Zhang, S. Cai, D. Wu, M. Chen, Y. Xia, J. Chen and B. Yang, Biosens. Bioelectron., 2015, 66, 399–404 CrossRef CAS.
- W. Liu, H. Zhu, B. Zheng, S. Cheng, Y. Fu, W. Li, T.-C. Lau and H. Liang, Nucleic Acids Res., 2012, 40, 4229–4236 CrossRef CAS.
- J. Kypr, I. Kejnovska, D. Renciuk and M. Vorlıckova, Nucleic Acids Res., 2009, 37, 1713–1725 CrossRef CAS.
- L. Ge, X. Sun, Q. Hong and F. Li, ACS Appl. Mater. Interfaces, 2017, 9, 13102–13110 CrossRef CAS.
- D. Fan, X. Zhu, Q. Zhai, E. Wang and S. Dong, Anal. Chem., 2016, 88, 9158–9165 CrossRef CAS.
- S. Tyagi and F. R. Kramer, Nat. Biotechnol., 1996, 14, 303–308 CrossRef CAS.
Footnote |
† Electronic supplementary information (ESI) available. See DOI: 10.1039/c9an01847f |
|
This journal is © The Royal Society of Chemistry 2020 |
Click here to see how this site uses Cookies. View our privacy policy here.