DOI:
10.1039/C9AN01653H
(Paper)
Analyst, 2020,
145, 107-114
Detection of breast cancer-derived exosomes using the horseradish peroxidase-mimicking DNAzyme as an aptasensor†
Received
27th August 2019
, Accepted 18th October 2019
First published on 21st October 2019
Abstract
Exosomes are membrane-enclosed phospholipid extracellular vesicles with a variety of tumor antigens which can be applied in the diagnosis and treatment of cancer due to the high secretion on the surface of cancer cells. Until now, many research studies on exosomes have been reported, but convenient and low-cost detection methods still need to be developed. Recently, we have developed a sensitive, simple and low-cost colorimetric aptasensor by designing a hairpin-like structure, which combined the highly specific MUC1 aptamer with a hemin/G-quadruplex for the detection of breast cancer exosomes. The hemin/G-quadruplex toward H2O2 reduction was used to generate an evidently strong colorimetric response owing to acting as a HRP-mimicking DNAzyme. The aptasensor is regarded as an “on–off” type switch, which strictly controls the process of reaction responding to the existence or not of exosomes. In our study, associated exosome detection limits are 3.94 × 105 particles per mL, which showed a higher sensitivity compared to commercial ELISA. Our method not only exhibited the advantages of being convenient and time-saving, with few instruments used, but also the signal generated using this method can be easily observed by the naked eye. Furthermore, the proposed strategy can well differentiate breast cancer patients from healthy individuals, demonstrating potential application in the analysis of clinical specimens.
1. Introduction
Breast cancer is one of the most common malignancies in the world, especially among women, and is the main cause of death next only to lung cancer.1,2 Hence early diagnosis of breast cancer is crucial to improve patient survival and develop curative treatments.3,4 In addition to the conventional serum markers like special proteins and nucleic acids, some microscale amounts of nanovesicles existing in the peripheral blood of breast cancer patients could serve as diagnostic biomarkers.5
Exosomes are nanovesicles secreted by almost all categories of cells of intracellular multivesicular bodies (MVBs) into the extracellular space when fusing with the plasma membrane.6 They distribute in all types of body fluids with diameters ranging from 50 to 150 nm. Initially, exosomes were regarded as “garbage bins” for transferring waste secreted by cells, and now they have been demonstrated to perform numerous functions involving intercellular communication and cellular immune response related to many pathophysiological conditions, including tumor progression, metastasis, etc.7–14 Due to their ability to carry enriched tumor-specific proteins, mRNA, DNA and microRNAs from parental cells, cancer-derived exosomes are elucidated as promising noninvasive biomarkers for identifying early stage tumors.5 Therefore, it is significant to develop effective analytical techniques for rapid and specific detection of breast cancer-derived exosomes.
Thus far, various approaches for detecting exosomes have been established, which include conventional nanoparticle tracking analysis (NTA),15,16 enzyme-linked immuno sorbent assay (ELISA),17 flow cytometry,18,19etc. These methods, however, still face some problems due to the complicated operation steps and expensive analytical instruments.20–22 Aptamers are not only a type of artificial nucleic acid ligand but also an emergent kind of immuno-affinity moiety that can function as effective molecular probes and exhibit high specificity and affinity toward a given target, endowing them with great potential for the detection of exosomes.23–26 They exhibit the advantages of easy synthesis, good biocompatibility, small size, and more importantly, high sensitivity, thus possessing broad biomedical applications on cell surfaces.27 Recently, aptasensors based on fluorescence,4 ultraviolet–vis spectroscopy,28 surface plasmon resonance (SPR),29 surface enhanced Raman scattering (SERS)30 and electrochemical detection31,32 were designed for exosome analysis. Most of the previous reports rely on the recognition between the most widely used CD63 aptamer and CD63-positive exosomes for signal generation.33 Nevertheless, CD63 is a member of the tetraspanin family existing on the surface of all exosomes with different expression levels. Hence, it is imperative to develop a more specific and simple method aiming at cancer-derived exosomes.
In addition, as a class of DNAzyme formed by guanine-rich nucleic acid sequences, hemin/G-quadruplex possesses horseradish peroxidase-mimicking catalytic activity and has been widely applied in sensor design for signal amplification. The hemin/G-quadruplex complex can efficiently catalyze the H2O2-mediated oxidation of several substrates accompanied by obvious color change, which is superior to native peroxidases because of their high chemical and thermal stability, low cost, simple preparation and easy modication.34,35
Herein, we chose mucin 1 (MUC1) protein as an ideal target molecule for the detection of exosomes secreted by MCF-7 breast cells (Scheme 1). MUC1 protein is a sort of transmembrane glycoprotein overexpressing on the MCF-7 cells compared to normal breast cells, specifically combining with corresponding aptamers with high affinity.4 Therefore, we designed a label-free aptasensor composed of the MUC1 aptamer and mimicking DNAzyme sequence at the end of the strand. The initial single-stranded DNA could form a hairpin structure in the case of 95 °C for 5 min, which will confine the G-rich mimicking DNAzyme sequence to the stem-loop structure.36 In the absence of target exosomes, the hairpin structure cannot function as a HRP-mimicking DNAzyme due to its spatial configurations. When the hairpin-like aptasensor specifically binds to the MUC1 expressed on the surface of MCF-7 exosomes, the specific interaction can unfold the original DNA hairpin and trigger the self-assembly of G-quadruplex subunits into a catalytically active G-quadruplex DNAzyme with the assistance of hemin. Subsequently, the reduction of H2O2 generates colorimetric signals and the blue colour change caused by the catalytic oxidation of ABTS can be easily observed by the naked eye, which might facilitate a visual analysis.37,38 The detection results could be readout directly through a microplate reader.9
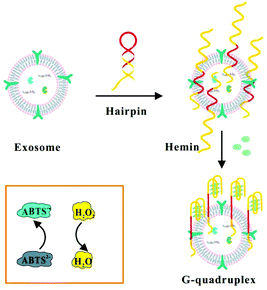 |
| Scheme 1 Schematic illustration of the assay for the detection of exosomes by binding to hairpin structures based on the combination of the MUC1 aptamer and G-quadruplex-mimetic enzyme. | |
2. Experimental section
2.1. Reagents and materials
4-(2-Hydroxyethyl)-1-piperazineethanesulfonic acid (HEPES), hemin, 2′-azino-bis (3-ethylbenzothiazoline-6-sulfonic acid) (ABTS), hydrogen peroxide (H2O2), RIPA lysis buffer and PMSF were purchased from Sigma Aldrich, Inc. (Saint Louis, MO, USA). Biotin-labeled mouse anti-human CD63 antibody (anti-CD63) was obtained from Biolegend (USA) and HRP-conjugated anti-mouse IgG was obtained from QF Biosciences Co., Ltd (Shanghai, China). Antibodies of CD63 and MUC1 were provided by Abcam (UK). A PS Capture Exosome ELISA Kit was purchased from Wako Pure Chemical Industries, Ltd. An ExoEasy Maxi Kit was purchased from Qiagen Translational Medicine Co. Ltd (Suzhou, China). A BCA Protein Assay Kit was purchased from Beyotime Biotechnology Co., Ltd (Shanghai, China). Dynabeads MyOne streptavidin magnetic beads (MBs), Dulbecco's modified Eagle's medium (DMEM), and fetal bovine serum (FBS) were obtained from Thermo Fisher Scientific Inc. (Waltham, MA, USA). Tween-20 was purchased from Aladdin Biochemical Technology Co., Ltd (Shanghai, China). Bovine serum albumin (BSA) and sodium dodecyl sulfate (SDS) were purchased from Sigma-Aldrich, Inc. (Saint Louis, MO, USA). A 100 kDa ultrafiltration device and 0.22 μm filters were purchased from Millipore Corp. (Bedford, MA, USA). All water throughout the experiment was purified with an electrical resistance of 18.2 MΩ cm using a Milli-Q water purification system (Millipore Corp., Bedford, MA, USA). All oligonucleotides (Table S1†) used in our study were synthesized by Sangon Biological Engineering Technology & Services Co., Ltd (Shanghai, China).
2.2 Instrumentation
Transmission electron microscopy (TEM) was performed on a JEOL JEM-1230 instrument (JEOL Ltd, Japan) using a working voltage of 120 kV and the sample was negatively stained with 2% aqueous uranyl acetate solution. Additionally, the size and particle concentration of exosomes were measured using nanoparticle tracking analysis (NTA, NS300, Malvern Instruments). The exosomal zeta potential was analyzed by dynamic light scattering (DLS, Zetasizer Nano ZS-90, Malvern Instruments). UV-vis absorption spectra were recorded by using a microplate reader (Bio-Tek Instrument, Winooski, USA) using a transparent 384-well microplate. The gels were visualized under a BioRad Molecular imager (Tanon-1600, China) and a Gel Image System (Tanon, China). MCF-7 cells were cultured in a constant temperature incubator (Eppendorf Galaxy 170S, Germany). FBS was ultracentrifuged using a Hitachi CP80MX with a P40ST rotor (Hitachi Aloka Medical, Tokyo, Japan). Mixing operations were performed on a HulaMixer sample mixer (Thermo Fisher Scientific Co., Ltd, Waltham, MA).
2.3. Preparation of the cell culture supernatant and collection of exosomes
We collected exosomes from the MCF-7 cell culture supernatant. The MCF-7 cells were cultured in DMEM, which was supplemented with 10% (v/v) FBS, and 1% (v/v) penicillin–streptomycin, and meanwhile, maintained at a temperature of 37 °C for 48 h with 5% CO2. After incubation for two or three days until they reached a confluency of 80%, the cell culture supernatant was collected into a 15 mL tube and centrifuged at 300g for 15 min at 4 °C to separate the floating cells and cell debris, followed by further centrifugation at 1200g for 20 min. After sequential centrifugation at 10
000g for 30 min, the medium supernatant was filtered through a 0.22 μm filter to thoroughly remove the large extracellular vesicles. The cell supernatant was discarded gently and then was washed with a large volume of PBS.39 Finally, we gathered the filtrate and stored it at −80 °C until use. Exosomes in the collected cell culture supernatant were extracted using an exoEasy Maxi Kit according to the manufacturer's instructions, and they were used as an exosome standard for subsequent experiments. The size distribution and concentration of purified exosomes were measured by qNano.
2.4. Preparation of bio-CD63 conjugated magnetic beads
To verify the combination of the DNA probe and exosomes, a control group was designed. First, 10 μL of streptavidin magnetic beads was added to 400 μL PBST (20 mM Tris-HCl, pH 7.4, 2 mM CaCl2, 150 mM NaCl, 0.0005% Tween 20) to give a primary wash and was subsequently resuspended in the PBST. Then, 2 μL of Bio-CD63 were added to the above mixed solution with gentle shaking for 30 min in order to obtain CD63-MB, followed by washing three times with washing buffer to remove superfluous Bio-CD63 and resuspending with 400 μL of buffer. Afterwards, the exosome samples were added and mixed gently with the above solution for 30 min, and incubated with 2 μL of DNA probe for 1 h at room temperature. Subsequently, the redundant solution was discarded and resuspended with 400 μL of buffer.
2.5. Analytical procedure
The specific procedure of our method for exosome detection was as follows. First, 2 μL of 1 mM DNA probe was incubated with 900 μL of 5 mM Hepes buffer, and then 20 μL of 20 mM MgCl2 were added to the above complexes, followed by incubation with 100 μL of different concentrations of exosomes. After incubation for 5 min at room temperature with gentle shaking, the DNA hairpin structure will be opened. Then, 1 μL of 1 μM hemin and 10 μL of 10 mM KCl were added into the mixture and incubated with the above complexes for 45 min to convert the exposed G4 sequence to a G-quadruplex. Next, the above mixture was mixed with a solution containing H2O2 (40 mM) and 2,2′-azino-bis (3-ethylbenzothiazoline-6-sulfonic acid) (ABTS) (40 mM). As a signal reporter, the HRP-mimicking activity of the G-quadruplex catalyzed the oxidation of ABTS, and the color depth increased in solution with the increase of exosome concentration. The maximum absorbance was at 420 nm observed for ABTS˙−. In another aspect, the catalytic oxidation of ABTS also caused a blue color change in solution which can be observed by the naked eye. Therefore, our method might provide a label-free visual method for the detection of exosomes.
2.6. ELISA for quantifying exosomes
Briefly, 100 μL of purified exosomes were directly anchored onto a Tim4-coated Exosome Capture 96 Well Plate at room temperature for 2 h. Then, 100 μL of control primary antibody anti-CD63 were added for 1 h at room temperature followed by three rinses with washing buffer, and the secondary antibody HRP-conjugated anti mouse IgG followed the same steps. Afterwards, 100 μL of TMB solution were mixed with the mixtures for 30 min and the plate was washed with washing buffer five times. When the reaction was completed, 100 μL of 1 M stop solutions-HCl was added, and absorbance was recorded at 450 nm using a microplate reader (BioTek, Synergy H1).
2.7. Western blot (WB)
The isolated exosomes and MCF-7 cells were added into the ice-cold RIPA lysis buffer containing PMSF (1 mM) at 4 °C for 30 min, respectively. All of the proteins lysates need to be resolved by sodium dodecyl sulfate polyacrylamide gel electrophoresis (SDS-PAGE) under nonreducing conditions, followed by being transferred onto PVDF. PDVF was then blocked with 5% (w/v) albumin bovine V in TBST (20 mM Tris-HCl, pH 7.6, 150 mM NaCl, and 0.1% Tween 20) for 2 h at room temperature. After successive incubation with primary antibody anti-CD63 overnight at 4 °C and secondary antibody HRP-conjugated anti-mouse IgG for 1 h at room temperature, immunoreactive bands were obtained and can be visualized by using a Gel Imaging System.
2.8. Clinical samples
We quantified exosomes in serum from six healthy individuals and six cancer patients to verify the application of our proposed method. All experiments were performed in accordance with the Guidelines for clinical trials of in vitro diagnosis (issued by China Food and Drug Administration, 2014), and approved by the ethics committee at Renji Hospital of Shanghai Jiao Tong University School of Medicine. Informed consent was obtained from human participants of this study. Prior to detection, serum was first centrifuged at 1200g for 20 min at 4 °C, followed by further centrifugation at 10
000g for 30 min at 4 °C. After centrifugation, the final serum-derived exosomes were obtained by using an ExoEasy kit. Then, the serum-derived exosomes were analyzed by our method and the results were compared with those measured by qNano.
3. Results and discussion
3.1. Exosome characterization
To investigate the morphology of MCF-7 exosomes and their relative purity, the MCF-7 exosomes were first isolated from the cell culture supernatant using a commercial ExoEasy kit, followed by being resuspended in PBS, and the well purified exosomes were then characterized by transmission electron microscopy (TEM).40 As shown in Fig. 1A, the captured exosomes have a typical saucer-like structure with an average diameter of 75 nm, whose size was within the characteristic diameter range. Then the effective exosomal markers CD9 and MUC1 were demonstrated by western blot analysis. CD9 is a kind of exosome-specific surface protein,41 while MUC1 is a major kind of transmembrane glycoprotein, both of which expressed on the surface of the MCF-7 cell membrane. As shown in Fig. 1B, CD9 and MUC1 were detected in the whole MCF-7 cell lysate and the isolated exosomes, which confirmed that the harvested vesicles from the cell supernatant were the MCF-7 exosomes according to their size and surface protein. NTA showed that the concentration of the MCF-7 exosomes was approximately 8.52 × 109 particles per mL and the size distribution was between 50 and 150 nm with an average diameter of 93 nm (Fig. S3B†), which verified that we have successfully extracted and purified MCF-7 exosomes. Zeta potential was around −12.2 mV, which similarly confirmed the size distribution of collected MCF-7 exosomes (Fig. S3A†).
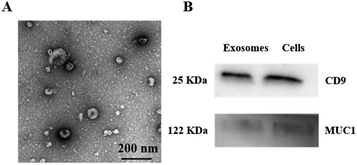 |
| Fig. 1 Characterization of purified MCF-7 exosomes. (A) TEM image of exosomes. (B) Western blot analysis of CD9 and MUC1 in exosomes and MCF-7 cells. | |
3.2. Optimization of the breast cancer exosome aptamer
In addition, the aptasensor pushes forward an immense influence on precise exosomes analysis; hence we designed another six single-stranded probes (Table S1†) according to the length and different cervical loop of the hairpin to screen out the optimal sequence in the absence and presence of exosomes. The optimum detection probe should have a stronger binding force to MCF-7 exosomes than others. As shown in Table S1,† the red marker is the MUC1 aptamer and the black marker represents the G-quadruplex sequence. Besides, the yellow part is the complementary area of the hairpin structure, including part of the aptamer and G-quadruplex sequence. When the cervical loop of the hairpin structure was 3
:
4, the absorbance could approach the highest level. Fig. S1† shows the relative signal, in which Aptasensor4 demonstrated the dramatically highest signal intensity among them. So, we selected Aptasensor4 as the probe in the following experiment and named it G-A. In addition, we designed another hairpin structure (Aptasensor R) that replaced the aptamer sequence with an arbitrary sequence in the neck region. As shown in Fig. S1,† the signal was similarly much lower than that of the hairpin structure containing the MUC1 aptamer.
3.3. Binding ability validation
In the presence of exosomes, the G-A specifically conjunct to the exosomes could significantly amplify the signal by catalyzing H2O2-mediated oxidation of ABTS. Fig. 2A shows a distinct color difference which verified the feasibility of the experiment. In order to demonstrate the binding ability between G-A and MCF-7 exosomes, we labeled the G-A with HRP and anti-CD63 modified magnetic beads which were introduced to capture exosomes beforehand. As illustrated in Fig. 2B, an obvious absorption pattern appeared with a maximum peak at 420 nm when the captured exosomes were incubated with HRP-labeled G-A (sample 4). The absence of any one of anti-CD63 (sample 3), exosomes (sample 4) and DNA probe (sample 5) will lead to a low signal, proving that the absorption band is derived from the bond between HRP-labeled G-A and exosomes. As a control, we also used an HRP-labeled random DNA (sample 2) to detect MCF-7 exosomes. As expected, the signal was much lower than that of HRP labeled G-A due to the specific recognition of the MUC1 aptamer in G-A.
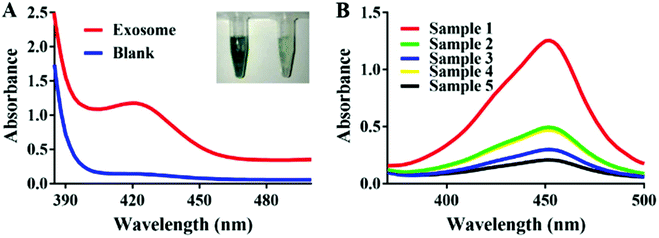 |
| Fig. 2 (A) Absorption spectra of the proposed method in the presence and absence of exosomes. (B) Comparison of absorption spectra of the reaction system including HRP-labeled DNA binding in exosomes, which were captured by anti-CD63 conjugated magnetic beads and four controls, which lack anti-CD63, exosomes, and DNA, respectively. Sample 1: Magnetic beads + antiCD63 + exosomes + DNA + HRP. Sample 2: Magnetic beads + antiCD63 + exosomes + RDNA + HRP. Sample 3: Magnetic beads + exosomes + HRP. Sample 4: Magnetic beads + anti-CD63 + DNA + HRP. Sample 5: Magnetic beads + anti-CD63 + exosomes + HRP. | |
3.4. Optimization of experimental conditions
As the most important and unique identifier in the reaction system, the sequence, length and concentration of the probe play key roles in affecting the signal strength. As shown in Fig. S2,† the absorption value reached a plateau after G-A concentration was increased to 50 nM, and a further increase of G-A concentration showed no significant influence on the relative signal growth rate; thus 50 nM was selected as the optimum DNA concentration in the following experiments. As the stabilizer of DNA hairpin and hemin/G-quadruplex-based DNAzyme, Mg2+ and hemin also exert an enormous effect on signal generation. As shown in Fig. S4A and B,† the signal is more pronounced in the case that the concentration of Mg2+ attains 30 mM, while from Fig. S4C and D,† we chose 2 μM as the optimized hemin concentration due to its highest relative signal. Similarly, the optimum concentration of ABTS (2 mM), which acted as the electronic intermediary in the reaction systems, was selected (Fig. S4E and F†). Meanwhile, we explored the influence of incubation time and temperature on the formation of the G-quadruplex and results prove that 45 min, 25 °C were the optimized parameters (Fig. S5A–C†). The whole reaction reached its maximum signal at the fifth minute according to the kinetic experiment (Fig. S6†).
3.5. Detection of exosomes
The sensitivity of the aptamer is also crucial to the progress of the experiment. To confirm whether our probe can successfully detect MCF-7 exosomes, we diluted exosomes to varying concentrations. Fig. 3A shows the absorbance changes in the proposed aptasensor responding to the distinct concentrations of exosomes. The UV-Vis absorption intensity increased significantly and showed a good linear relationship with the increasing exosome concentration, ranging from 8.3 × 105 to 5.3 × 107 exosomes per mL. Additionally, the detection limit of our assay was calculated as 3.94 × 105 particles per mL based on 3 times the standard deviations of the background, which verified that our proposed method had higher sensitivity than the recently reported methods for exosome detection. The designed strategy has advantages of lower cost and less time consumption over some of the above methods. It only took less than an hour to perform our whole experiment; thereby it can be used in circumstances when analysis results are needed urgently (Table 1). According to the seven concentrations of MCF-7 exosomes, the standard curve was calculated and the correlation equation could be displayed as y = 1 × 10−7x + 0.6541, (R2 = 0.9877), which confirmed that the designed G-quadruplex-mimetic enzyme reaction strategy can be smoothly applied in MCF-7 exosome detection. In comparison, the ELISA Kit based on a Tim4 coated microplate was also used to detect MCF-7 exosomes, which yielded lower sensitivity as well as a narrower linear range than our method (Fig. 3C and D).
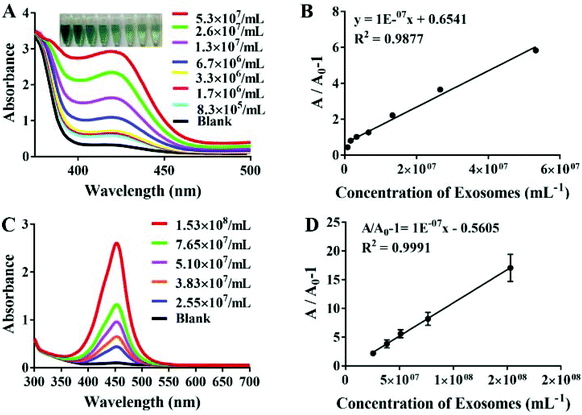 |
| Fig. 3 (A) UV–vis absorption spectra of the reaction system in the presence of different concentrations of exosomes; (B) the linear relationship of absorbance. The linear range of exosome concentration varied from 8.3 × 105 to 5.3 × 107 exosomes per mL; (C) absorption spectra of ELISA with different concentrations of MCF-7 exosomes; (D) the linear relationship of absorbance. The linear range of exosome concentration varied from 2.55 × 107 to 1.53 × 108 exosomes per mL. | |
Table 1 Comparison of our method and the recently reported methods for the detection of exosomes
Platform |
Linear range (particles per mL) |
LOD (particles per mL) |
Method |
Assay time |
Ref. |
B-Chol anchor assay with enzyme-linked HCR |
2.3 × 106–2.3 × 108 |
2.2 × 106 |
Colorimetric |
∼16.5 h |
20
|
Microfluidic-based mobile exosome detector (μMED) |
107–1011 |
1 × 107 |
Fluorescence |
∼1 h |
42
|
pH-Responsive bioassay |
5 × 106–1 × 109 |
4.46 × 106 |
PH |
∼2 h |
43
|
Paper-based aptasensor |
107–1011 |
1.1 × 106 |
Luminescence |
|
44
|
A dual functional platform |
106–1011 |
105 |
Colorimetric |
|
45
|
Aptasensor based on DNA-capped s-SWCNTs |
1.84 × 109–2.21 × 1010 |
5.2 × 108 |
Colorimetric |
40 min |
46
|
Aptasensor based on g-C3N4 nanosheets |
1.9 × 109–3.38 × 1010 |
1.35 × 109 |
Colorimetric |
∼30 min |
47
|
Copper-Mediated aptasensor |
7.5 × 107–1.5 × 1010 |
4.8 × 107 |
Fluorescence |
∼2 h |
9
|
G-A bioassay |
8.3 × 105–5.3 × 107 |
3.94 × 105 |
Colorimetric |
∼1 h |
This work |
3.6. Assay specificity
For the specificity study, four kinds of tumor cells and one kind of normal cell were employed to be treated under the same conditions. As can be shown in Fig. 4, the absorbance arranged in descending order was MCF-7 exosomes > SKBR3 exosomes > MD-MB231 exosomes > HepG2 exosomes > L-02 exosomes. The signal produced by exosomes from the tumor cells was significantly higher than that from the normal liver cell L-02, which signified that the MUC1 protein was over-expressed in these tumor exosomes, especially in breast cancer cells. Despite the fact that there was no significant difference in expression between cancer cells, our diagnostic approach can still play an indispensable role in cancer diagnosis.
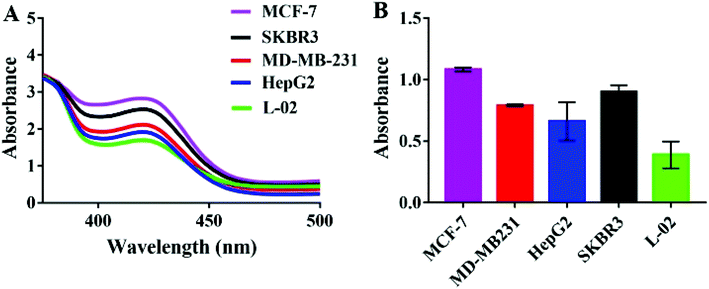 |
| Fig. 4 (A) On the basis of the fixed number of exosomes, absorption spectra response to exosomes isolated from MCF-7 cells, SKBR3 cells, MD-MB-231 cells, HepG2 cells and L-02 cells. (B) The histogram of specificity. Error bars correspond to the standard error of the mean of three independent replicates. | |
3.7. Detection of exosomes in human serum samples
In order to verify the possibility of applying this aptasensor for practical use, we performed exosome analysis by detecting twelve freshly collected clinical serum samples from six healthy individuals and six breast cancer patients. Briefly, we isolated exosomes from serum samples separately by employing an exoEasy Maxi Kit, followed by detecting the signal as described above to evaluate the practicality of our method. As shown in Fig. 5, the breast cancer group and the healthy group could be well separated by our strategy and the absorbance of MUC1 from exosomes in the breast cancer group (n = 6) was consistently higher than that of the healthy donor group (n = 6), indicating a difference between the two groups (Student's t-test, p < 0.05). MUC1 is a major kind of transmembrane glycoprotein generally overexpressing on the MCF-7 cells. Thus, we can detect large numbers of exosomes in serum samples of cancer patients. As the MUC1 may also exist on the surface of the normal cell-derived exosomes, the signal difference between healthy and patient groups is not too large. Meanwhile, we speculated that a relatively small number of clinical samples may also bring some deviations when exploring the differential expression of MUC1 protein in exosomes derived from different parents. To further evaluate the accuracy of our method, exosomes were quantified by NTA. The results showed that the concentrations of exosomes were equivalent to those obtained by NTA which demonstrates the relatively high accuracy of our method (Fig. 6).
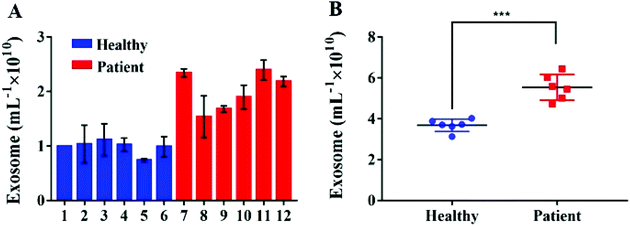 |
| Fig. 5 Quantitative detection of exosomes directly from clinical serum samples. (A) Serum sample diagnostic by our method discriminates between six normal controls and six breast cancer patients with high accuracy. (B) Boxplots overlaid with dot plots corresponding to the data (A). | |
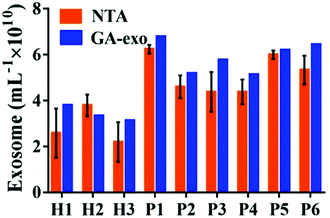 |
| Fig. 6 Comparison of exosomes detected by our method and NTA in serum samples from three healthy individuals and six breast cancer patients. | |
4. Conclusions
In conclusion, we have developed a sensitive, label-free colorimetric aptasensor by combining the MUC1 aptamer with a hemin/G-quadruplex for specific detection of breast cancer exosomes. The combined action of the hemin/G-quadruplex toward H2O2 reduction was used to generate an evidently strong colorimetric response due to acting as a HRP-mimicking DNAzyme. Our method not only exhibited the advantages of being convenient and time-saving, with few instruments used, but also the signal generated using this method can be easily observed by the naked eye. In addition, our diagnostic strategy based on exosomes has a lot of potential to be used for probing versatile targets owing to the substitutability of the target detector and can open up broad prospects for the diagnosis of cancer in the future.
Conflicts of interest
There are no conflicts to declare.
Acknowledgements
This work was supported by the National Natural Science Foundation of China (Grant No. 21705047, 21575089, and 21335003) and the Fundamental Research Funds for the Central Universities (Grant No. 222201814030).
Notes and references
- A. Gulmez, Curr. Probl. Cancer, 2019, 10, 51 Search PubMed
.
- W. Zhou, Y. F. Tian, B. C. Yin and B. C. Ye, Anal. Chem., 2017, 89, 6120–6128 CrossRef CAS
.
- J. L. Gonzalez-Hernandez, A. N. Recinella, S. G. Kandlikar, D. Dabydeen, L. Medeiros and P. Phatak, Int. J. Heat Mass Transfer, 2019, 131, 558–573 CrossRef
.
- J. Zhang, J. Shi, W. Liu, K. X. Zhang, H. J. Zhao, H. L. Zhang and Z. Z. Zhang, Sens. Actuators, B, 2018, 276, 552–559 CrossRef CAS
.
- L. Y. Zhai, M. X. Li, W. L. Pan, Y. Chen, M. M. Li, J. X. Pang and L. Zheng, ACS Appl. Mater. Interfaces, 2018, 10, 39478–39486 CrossRef CAS
.
- J. S. Zachary, L. Changwon, R. Tatu, P. C. Randy, H. Sidhartha, K. Alisha, L. Kit, S. Heikki, L. I. Elisa, V. Tapani, L. Timo, Y. Marjo and W. H. Sebastian, J. Extracell. Vesicles, 2015, 4, 28533 CrossRef
.
- Y. C. Yang, K. Eric, G. Yu, R. E. Mary, S. K. Patnaik and Y. Wu, ACS Appl. Mater. Interfaces, 2018, 10, 43375–43386 CrossRef CAS
.
- X. Doldan, P. Fagundez, A. Cayota, J. Laíz and J. P. Tosar, Anal. Chem., 2016, 88, 10466–10473 CrossRef CAS
.
- F. Sun, X. Y. Guo, J. Z. Wang, Y. Q. Wang and Q. Pan, Nanosci. Nanotechnol. Lett., 2018, 8, 1546–1553 CrossRef
.
- Z. Zhao, Y. Yang, Y. Zeng and M. He, Lab Chip, 2016, 16, 489 RSC
.
- S. A. Melo, H. Sugimoto, J. T. O'Connell, N. Kato, A. Villanueva, A. Vidal, L. Qiu, E. Vitkin, L. T. Perelman, C. A. Melo, A. Lucci, C. Ivan, G. A. Calin and R. Kalluri, Cancer Cell, 2014, 26, 707–721 CrossRef CAS
.
- G. Camussi, M. C. Deregibus, S. Bruno, V. Cantaluppi and L. Biancone, Kidney Int., 2010, 78, 838–848 CrossRef CAS
.
- J. P. Shu, S. S. Mo, Q. Wen, Y. Qin and L. Jiang, Nanosci. Nanotechnol. Lett., 2018, 10, 1598–1605 CrossRef
.
- W. R. Meng, Y. Y. Hao, C. S. He, L. Li and G. Q. Zhu, Mol. Cancer, 2019, 18, 57 CrossRef
.
- Y. Soo, Y. Song, Y. Zheng, E. C. Campbell, A. C. Riches, F. Gunn-Moore and S. J. Powis, Immunology, 2012, 136, 192 CrossRef
.
- W. Oosthuyzen, N. E. Sime, J. R. Ivy, E. J. Turtle, J. M. Street, J. Pound, L. E. Bath, D. J. Webb, C. D. Gregory, M. A. Bailey and J. W. Dear, Physiology, 2013, 591, 5833 CrossRef CAS
.
- Y. Weng, Z. Sui, Y. Shan, Y. Hu, Y. Chen, L. Zhang and Y. Zhang, Analyst, 2016, 141, 4640 RSC
.
- E. J. van der Vlist, E. N. Nolte-'t Hoen, W. Stoorvogel, G. J. Arkesteijn and M. H. Wauben, Nat. Protoc., 2012, 7, 1311 CrossRef
.
- E. N. Nolte-'t Hoen, E. J. van der Vlist, M. Aalberts, H. C. Mertens, B. J. Bosch, W. Bartelink, E. Mastrobattista, E. V. van Gaal, W. Stoorvogel, G. J. Arkesteijn and M. H. Wauben, Nanomedicine, 2012, 85, 712–720 CrossRef
.
- F. He, H. Liu, X. G. Guo, B. C. Yin and B. C. Ye, Anal. Chem., 2017, 89, 12968–12975 CrossRef CAS PubMed
.
- Y. Wan, G. Cheng, X. Liu, S. J. Hao, N. Merisa, C. D. Zhu, Y. Q. Xia, W. Q. Li, Z. G. Wang, W. L. Zhang, J. R. Shawn, S. Aswathy, A. Istvan, P. B. Chandra and S. Y. Zheng, Nat. Biomed. Eng., 2017, 1, 0058 CrossRef
.
- L. Huang, D. B. Wang, S. Netrapal, F. Yang, N. Gu and X. E. Zhang, Nanoscale, 2018, 10, 20289–20295 RSC
.
- K. O'Sullivan, Anal. Bioanal. Chem., 2002, 372, 44–48 CrossRef
.
- L. Wang, Y. C. Yang, Y. F. Liu, L. M. Ning, Y. Xiang and G. X. Li, Chem. Commun., 2019, 55, 2708 RSC
.
- Q. Wang, L. Y. Zou, X. H. Yang, X. F. Liu, W. Y. Nie, Y. Zheng, Q. Cheng and K. M. Wan, Biosens. Bioelectron., 2019, 135, 129–136 CrossRef CAS
.
- S. Wan, L. Q. Zhang, S. Wang, Y. Liu, C. C. Wu, C. Cui, H. Sun, M. L. Shi, Y. Jiang, L. Li, L. P. Qiu and W. H. Tan, J. Am. Chem. Soc., 2017, 139, 5289–5292 CrossRef CAS PubMed
.
- L. Li, Y. Jiang, C. Cui, Y. Yang, P. H. Zhang, K. Stewart, X. S. Pan, X. W. Li, L. Yang, L. P. Qiu and W. H. Tan, J. Am. Chem. Soc., 2018, 140, 13335–13339 CrossRef CAS PubMed
.
- H. Wu, Y. L. Liu, H. Y. Wang, J. Wu, F. F. Zhu and P. Zou, Biosens. Bioelectron., 2016, 81, 303–308 CrossRef CAS
.
- H. Im, H. Shao, Y. I. Park, V. M. Peterson, C. M. Castro, R. Weissleder and H. Lee, Nat. Biotechnol., 2014, 32, 490–495 CrossRef CAS PubMed
.
- Z. L. Wang, S. F. Zong, Y. J. Wang, N. Li, L. Li, J. Lu, Z. Y. Wang, B. A. Chen and Y. P. Cui, Nanoscale, 2018, 10, 9053 RSC
.
- S. Wang, L. Zhang, S. Wan, S. Cansiz, C. Cui, Y. Liu, R. Cai, C. Hong, I. T. Teng, M. Shi, Y. Wu, Y. Dong and W. Tan, ACS Nano, 2017, 11, 3943 CrossRef CAS
.
- Y. G. Zhou, R. M. Mohamadi, M. Poudineh, L. Kermanshah, S. Ahmed, T. S. Safaei, J. Stojcic, R. K. Nam, E. H. Sargent and S. O. Kelley, Small, 2016, 12, 727 CrossRef CAS
.
- X. C. Yu, L. He, M. Pentok, H. W. Yang, Y. L. Yang, Z. Y. Li, N. Y. He, Y. Deng, S. Li, T. H. Liu, X. Y. Chen and H. W. Luo, Nanoscale, 2019, 11, 15589 RSC
.
- Y. L. Yuan, Y. Q. Chai, R. Yuan, Y. Zhuo and X. X. Gan, Chem. Commun., 2013, 49, 7328–7330 RSC
.
- S. P. Li, L. Q. Wang, Y. Q. Hao, L. L. Zhang, B. B. Zhou, L. Deng and Y. N. Liu, RSC Adv., 2014, 4, 23185 RSC
.
- P. Liang, P. Q. Ma, H. Liu, X. G. Guo, B. C. Yin and B. C. Ye, Angew. Chem., Int. Ed., 2017, 129, 9205–9209 CrossRef
.
- R. R. Huang, L. He, Y. Y. Xia, H. P. Xu, C. Liu, H. Xie, S. Wang, L. J. Peng, Y. F. Liu, Y. Liu, N. Y. He and Z. Y. Li, Small, 2019, 15, e1900735 CrossRef
.
- R. D. Li, B. C. Yin and B. C. Ye, Biosens. Bioelectron., 2016, 86, 1011–1016 CrossRef CAS
.
- S. Ramesh, P. Radhika, W. Kounosuke, Z. H. Lu and Y. Y. Mo, Mol. Cancer, 2014, 13, 256 CrossRef
.
- Z. Y. Li, C. Y. Hu, J. Jia, Y. Y. Xia, H. Xie, M. J. Shen, R. R. Huang, L. He, C. Liu, S. Wang, B. J. Chen and N. Y. He, J. Biomed. Nanotechnol., 2019, 5, 1090–1096 CrossRef
.
- W. Nakai, Y. Takeshi, D. Diego, M. Yuji, N. Takahiro, I. Naoko, N. Ken, S. Yoshifusa and H. Rikinar, Sci. Rep., 2016, 6, 33935 CrossRef CAS
.
- J. Ko, M. A. Hemphill, D. Gabrieli, L. Wu, V. Yelleswarapu, G. Lawrence, W. Pennycooke, A. Singh, D. F. Meaney and D. Issadore, Sci. Rep., 2016, 6, 31215 CrossRef CAS
.
- Y. Yang, C. Li, H. Shi, T. Chen, Z. Wang and G. Li, Talanta, 2019, 192, 325–330 CrossRef CAS
.
- X. Chen, J. Lan, Y. Liu, L. Li, L. Yan, Y. Xia, F. Wu, C. Li, S. Li and J. Chen, Biosens. Bioelectron., 2018, 102, 582–588 CrossRef CAS
.
- P. Li, X. Y. Yu, W. J. Han, Y. Kong, W. Y. Bao, J. Q. Zhang, W. C. Zhang and Y. Q. Gu, ACS Sens., 2019, 4, 1433–1441 CrossRef CAS
.
- Y. K. Xia, M. M. Liu, L. L. Wang, A. Yan, W. H. He, M. Chen, J. M. Lan, J. X. Xu, L. H. Guan and J. H. Chen, Biosens. Bioelectron., 2017, 92, 8–15 CrossRef CAS
.
- Y. M. Wang, J. W. Liu, G. B. Adkins, W. Shen, M. P. Trinh, L. Y. Duan, J. H. Jiang and W. Zhong, Anal. Chem., 2017, 89, 12327–12333 CrossRef CAS
.
Footnote |
† Electronic supplementary information (ESI) available. See DOI: 10.1039/c9an01653h |
|
This journal is © The Royal Society of Chemistry 2020 |