DOI:
10.1039/C9TC03582F
(Paper)
J. Mater. Chem. C, 2019,
7, 10851-10859
High-efficiency pure blue thermally activated delayed fluorescence emitters with a preferentially horizontal emitting dipole orientation via a spiro-linked double D–A molecular architecture†
Received
3rd July 2019
, Accepted 1st August 2019
First published on 5th August 2019
Abstract
Blue as one of the three primary colors is of great significance for lighting and full-color displays, and the development of blue emitters with satisfactory color purity and high efficiency is still a formidable challenge for organic light-emitting diodes (OLEDs). In this study, a spiro-linked double donor–acceptor (D–A) molecular architecture is introduced to selectively improve the horizontal emitting dipole orientation of blue emitters and thereby boost the electroluminescence (EL) performance of blue OLEDs. To testify the validity, a thermally activated delayed fluorescence (TADF) emitter, namely SBA-2DPS, is designed by connecting two D–A pairs with a sp3 hybrid carbon atom. Compared to the prototypical emitter DMAC-1DPS, the shape of SBA-2DPS is elongated dramatically without expanding the degree of π-conjugation owing to the σ-spacer linkage. Consequently, a high horizontal dipole ratio (Θ‖ = 87%) is achieved for SBA-2DPS. Besides, SBA-2DPS exhibits high thermal stability, pure blue emission and distinct TADF characteristics. OLEDs based on SBA-2DPS exhibit an external quantum efficiency (ηext) of 25.5% and favorable Commission International de l’Eclairage (CIE) coordinates of (0.15, 0.20). Such an inspiring performance is rationalized by their high out-coupling efficiency (Φout = 38.2%) and the optical microcavity effect. Unambiguously, this finding demonstrates the validity of the spiro-linked double D–A molecular architecture in constructing good blue TADF emitters with a preferentially horizontal emitting dipole orientation for high-efficiency blue OLEDs.
Introduction
Organic light-emitting diodes (OLEDs) have been regarded as a promising light source for flat-panel displays and solid-state lighting owing to their various advantages, such as being flexible and lightweight and having low-power-consuming characteristics.1–3 Since first reported by Tang and Van Slyke, the common goal for OLEDs is to boost their electroluminescence (EL) performance, especially external quantum efficiencies (ηexts).4–7 As an important factor to characterize the EL efficiencies of OLEDs, ηext is determined using the following factors:8where γ is the charge balance factor, which can be close to unity by device optimization; ηr is the fraction of radiative excitons determined by spin statistics; ΦPL refers to the photoluminescence quantum yield (PLQY) and can also approach 100% with a suitable molecular design; Φout represents the optical out-coupling efficiency, which is normally less than 25% for conventional planar OLEDs with isotropically oriented emitters.9 With respect to conventional fluorescent emitters, only 25% of singlet excitons can be harvested by radiative processes, leading to poor device performance with an upper limit ηext of 5%.10,11 To address this issue, many efforts have been devoted to exploring novel emitters with a higher ηr by harnessing 75% of triplet excitons.12–14 Among them, the most successful one is phosphorescent heavy-metal complexes that could harness both singlet and triplet excitons by spin–orbital coupling.15,16 However, the introduction of noble metals such as iridium (Ir) and platinum (Pt) raises the material cost and thus restricts their commercial applications.17 In this context, thermally activated delayed fluorescence (TADF) emitters have emerged as a promising alternative due to their ability to harvest triplet excitons by a reverse intersystem crossing (RISC) process without involving noble metals.6,18–20 Apart from ηr, another key factor in determining ηext is Φout according to eqn (1). As mentioned above, the value of Φout is usually less than 25% for conventional planar OLEDs with isotropically oriented emitters. However, this value can be upgraded to over 45% for emitters with a perfectly horizontal emitting dipole orientation even in the absence of optical out-coupling structures, mainly due to the reduction of surface plasmon coupling at the organic/cathode interface and more favorable radiation patterns of horizontal dipoles for direct optical out-coupling.21 Therefore, enhancing the horizontal dipole ratios of emitters has become an effective approach for achieving high Φouts in OLEDs.
Taking ηr and Φout into account, developing TADF emitters with a preferentially horizontal emitting dipole orientation will further boost their EL performance.20,22–24 Some strategies have been proposed to increase the horizontal dipole ratios of TADF emitters.25 In some cases, when a transition dipole moment (TDM) locates along the long axis of a molecule or in the molecular plane, the horizontal dipole ratio can be promoted by altering the molecular shapes, such as elongating the molecular length or broadening the molecular planarity.26 However, these strategies may enlarge the degree of π-conjugation of TADF molecules and cause a redshift in their emission spectra, making them unsuitable for designing blue TADF emitters, especially in the pure blue or deep blue region.27 Therefore, a judicious strategy is highly desirable for modulating the molecular shapes of blue TADF emitters to achieve high horizontal dipole ratios while maintaining their limited molecular conjugation.
In this study, a spiro-linked double D–A molecular architecture was introduced to construct rod-like TADF emitters, which will selectively elongate the molecular shape, but barely affect the degree of π-conjugation owing to the σ-spacer linkage between two counterparts. As shown in Fig. 1a, a TADF emitter, namely SBA-2DPS, possessing diphenyl sulfone (DPS) as an electron acceptor (A) unit and spirobiacridine (SBA) as an electron donor (D) unit was designed and synthesized. For comparison, a prototypical molecule DMAC-1DPS was also synthesized and had a single counterpart possessing the DPS acceptor and a 9,9-dimethyl-9,10-dihydroacridine (DMAC) donor. Further studies using molecular simulations and experiments demonstrated that the molecular length of SBA-2DPS was significantly elongated without extending the π-conjugation compared to DMAC-1DPS. As a result, SBA-2DPS showed blue emission with a moderate ΦPL value and distinct TADF properties like DMAC-1DPS, but exhibited a much higher horizontal dipole ratio Θ‖ of 87% in comparison with 59% for DMAC-1DPS. Consequently, OLEDs employing SBA-2DPS as an emitter showed pure blue emission with Commission International de l’Eclairage (CIE) coordinates of (0.15, 0.20), and exhibited excellent EL performance with a peak ηext of 25.5%. This performance is outstanding compared with that of the best blue TADF emitters with similar color coordinates.28,29 These results testify the validity of the spiro-linked double D–A molecular architecture in developing pure blue TADF emitters with high Θ‖ and ηext.
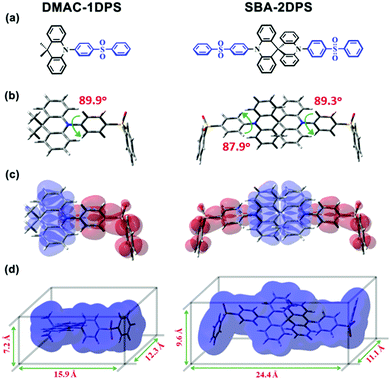 |
| Fig. 1 (a) Molecular structures, (b) optimized ground state structures, (c) the HOMO (blue) and LUMO (red) distributions with optimized ground state structures, (d) isodensity surface of the designed molecules with ρ = 0.001 e Bohr−3. | |
Results and discussion
DFT calculations
It is known that the well-separated frontier molecular orbitals (FMOs) can result in a small energy gap (ΔEST) between the lowest excited singlet (S1) and triplet (T1) states.30,31 Therefore, density functional theory (DFT) calculations were performed to predict the FMOs and electronic properties of the designed molecules at first. As shown in Fig. 1b, both molecules showed a highly twisted structure in the ground state with dihedral angles, between the DMAC or SBA moiety and the DPS unit, of 89.9° for DMAC-1DPS, and 87.9°/89.3° for SBA-2DPS. Accordingly, the highest occupied molecular orbitals (HOMOs) of both emitters were mainly located on the DMAC or SBA moieties, while their lowest unoccupied molecular orbitals (LUMOs) were mainly localized on the DPS moiety, exhibiting a small overlap between the HOMOs and LUMOs (Fig. 1c). Furthermore, time-dependent DFT (TD-DFT) calculations based on the optimized ground structure were conducted to study their excited states. As summarized in Table 1, the energy levels of S1/T1 were estimated to be 3.42/3.27 eV for DMAC-1DPS and 3.31/3.23 eV for SBA-2DPS. In comparison with DMAC-1DPS, the π-conjugation of SBA-2DPS is barely affected owing to the σ-spacer linkage of the sp3 hybrid carbon atom. Correspondingly, the ΔEST values for both molecules were calculated to be 0.15 eV for DMAC-1DPS and 0.08 eV for SBA-2DPS, suggesting that the designed molecules could be potential TADF materials.32 Moreover, considering the correlation between molecular orientation and molecular shape, the spatial ranges of both molecules were quantified using the isodensity surface at ρ = 0.001 e Bohr−3 generated by the Marching Tetrahedra (MT) approach with their optimized ground state structures (Fig. 1d and Table 1).33 Along the x axis in molecular cartesian coordinates, Xmax and Xmin were estimated to be 12.2 Å and −12.2 Å for SBA-2DPS, and 8.1 Å and −7.8 Å for DMAC-1DPS, respectively. Apparently, the molecular length of SBA-2DPS along its long axis is 1.53 fold longer than that of DMAC-1DPS. Meanwhile, the aspect ratios of length to width for both molecules were also determined to be 2.20 for SBA-2DPS and 1.29 for DMAC-1DPS. These results manifest that SBA-2DPS possesses a more linear shape than DMAC-1DPS, which indicates that SBA-2DPS has a better horizontal molecular orientation.34
Table 1 Key data obtained from the DFT and TD-DFT calculations
Compound |
HOMOa [eV] |
LUMOa [eV] |
Energy levelb [eV] |
X
max/Xminc [Å] |
Y
max/Yminc [Å] |
Z
max/Zminc [Å] |
S1 |
T1 |
ΔEST |
Obtained from DFT calculations.
Obtained from TD-DFT calculations with the optimized ground state structure.
The spatial range of the emitter's isodensity surface in the Cartesian axes generated by the Marching Tetrahedra (MT) approach. Xmax/Xmin, Ymax/Ymin and Zmax/Zmin are the maximum/minimum values of the isodensity surface in the x axis, y axis and z axis, respectively.
|
DMAC-1DPS
|
−5.07 |
−1.58 |
3.42 |
3.27 |
0.15 |
8.1/−7.8 |
6.4/−5.9 |
3.6/−3.6 |
SBA-2DPS
|
−5.17 |
−1.63 |
3.31 |
3.23 |
0.08 |
12.2/−12.2 |
5.4/−5.7 |
4.8/−4.8 |
Synthesis and characterization
DMAC-1DPS and SBA-2DPS were synthesized by Pd-catalyzed C–N coupling reaction of 1-bromo-4-(phenylsulfonyl)benzene with 9,9-dimethyl-9,10-dihydroacridine or 9,9′(10H,10′H)-spirobiacridines. After purification by vacuum sublimation, the chemical structures of the target compounds were characterized by 1H NMR, 13C NMR and high resolution mass spectrometry (HRMS). Their molecular structures were further identified by single crystal X-ray crystallographic analysis. As shown in Fig. 2, DMAC-1DPS and SBA-2DPS exhibited a twisted configuration with large dihedral angles between the electron donor and acceptor units of 83.8° for DMAC-1DPS, and 77.3° and 78.0° for SBA-2DPS. Different from the predicted quasi-plane structures of both molecules in DFT simulations, their donor units preferred crooked configurations in the single crystal, which releases the steric hindrance between acridine units and DPS units. Moreover, SBA-2DPS possessed a much longer molecular length than DMAC-1DPS in the crystal structure, which is in accordance with the DFT results. According to the packing diagram shown in Fig. 2c and d, no obvious π–π stacking contact was observed for both molecules and SBA-2DPS aligned orderly parallel to its long axis.
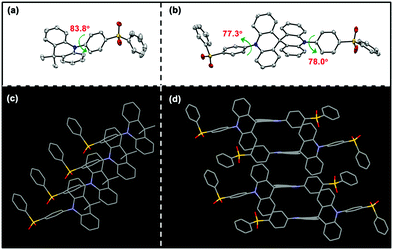 |
| Fig. 2 Oak ridge thermal ellipsoid plot (ORTEP) diagrams of (a) DMAC-1DPS and (b) SBA-2DPS; (c) molecular packing diagram of DMAC-1DPS and (d) molecular parking diagram of SBA-2DPS without showing hydrogen atoms and solvent molecules (solvent molecules: chloroform). | |
Thermal and electrochemical properties
To evaluate the thermal properties of DMAC-1DPS and SBA-2DPS, thermogravimetric analysis (TGA) and differential scanning calorimetry (DSC) were performed, and the key data are summarized in Table S1 (ESI†). As depicted in Fig. 3, SBA-2DPS exhibited high thermal stability with a decomposition temperature (Td, with 5% weight loss) of 476 °C, whereas DMAC-1DPS showed a much lower Td of 295 °C. From the DSC curves, the glass transition temperatures (Tgs) of both compounds were determined to be 69 °C and 176 °C for DMAC-1DPS and SBA-2DPS, respectively. In comparison to the prototypical molecule DMAC-1DPS, the improved thermal stability of SBA-2DPS is attributed to its more rigid structure and larger molecular weight.35
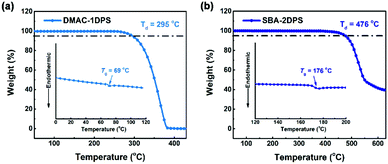 |
| Fig. 3 TGA traces of (a) DMAC-1DPS and (b) SBA-2DPS recorded at a heating rate of 10 °C min−1. Inset: DSC curves recorded at a heating rate of 10 °C min−1. | |
As shown in the cyclic voltammetry curves of both compounds (Fig. S1a, ESI†), DMAC-1DPS showed a one-electron oxidation process arising from the DMAC unit, whereas SBA-2DPS displayed a double-electron oxidation process, which may be related to the asymmetrically spatial configuration of its donor SBA moiety.36 From the onset voltages of the oxidation curves, the HOMO levels were determined to be −5.40 eV for DMAC-1DPS and −5.49 eV for SBA-2DPS. The deeper HOMO level of SBA-2DPS may be ascribed to the weaker electron-donating ability of the SBA moiety. To verify this conjecture, the cyclic voltammograms of SBA and DMAC moieties were obtained. As shown in Fig. S1b (ESI†), the HOMO levels were estimated to be −5.25 eV for SBA and −5.18 eV for DMAC moieties, which coincide well with our expectation. From the estimated energy gaps (Egs) and HOMO levels of both compounds, their LUMO levels were estimated to be −2.34 eV for DMAC-1DPS and −2.42 eV for SBA-2DPS. This trend coincides well with the DFT results.
Photophysical properties
As shown in Fig. 4a, a broad and weak absorption around 365 nm was observed for both emitters in toluene solution, which could be assigned to the charge transfer (CT) type transition from the acridine units to the DPS unit. Besides, DMAC-1DPS and SBA-2DPS showed distinct absorption signals in the shorter wavelength region, which may originate from the local transition of the DPS units and the donor units. With respect to photoluminescence (PL), DMAC-1DPS and SBA-2DPS showed pure blue emission in toluene with main peaks at 452 nm and 442 nm (Fig. 4a and Table 2), respectively. Their photophysical properties in doped films were also studied by doping these emitters into a widely used host material of bis(2-(diphenylphosphino)phenyl)ether oxide (DPEPO).37 In comparison with their PL spectra in toluene, the emission peaks of DMAC-1DPS and SBA-2DPS in doped films slightly bathochromically shifted to 468 nm and 459 nm (Table 2), respectively. Notably, the emission spectra of SBA-2DPS is hypsochromic compared with those of DMAC-1DPS, no matter in toluene or in the DPEPO host, which may be due to the weaker electron-donating ability of the SBA moiety (as confirmed by CV) and thus weaker intramolecular charge transfer (ICT) transition between the DPS and SBA units.38 According to the onset wavelengths of fluorescence and phosphorescence spectra (Fig. 4b), the S1/T1 values of both emitters were estimated to be 3.04/2.95 eV for SBA-2DPS, and 2.98/2.91 eV for DMAC-1DPS (Table 2). Correspondingly, ΔESTs was determined to be 0.09 eV for SBA-2DPS and 0.07 eV for DMAC-1DPS. Such small values were favourable for boosting the RISC process from T1 to S1 with thermal aid. Indeed, the RISC process of both emitters was confirmed by their transient PL decay curves in the doped films as depicted in Fig. 4c. Obviously, their fluorescence intensity showed second-order exponential decays with a delayed ratio over 50% and the lifetimes of the prompt and delayed components were fitted to be 19.0 ns/4.3 μs for SBA-2DPS and 20.4 ns/4.2 μs for DMAC-1DPS (Table 2). Moreover, the PLQYs of SBA-2DPS and DMAC-1DPS in the DPEPO host (30 wt%) were measured to be 60% and 61% under argon conditions, suggesting that the emission capability of double D–A emitters is rarely affected. To gain a deeper understanding on their emission properties, related photophysical rate constants were further calculated according to previous reports by assuming that most of the triplet states can return to singlet states through RISC and major nonradiative losses occur in singlet states.18 As summarized in Table S2 (ESI†), the radiative decay rate from the S1 state (kr,S) of SBA-2DPS was estimated to be 1.37 × 107 s−1, which is comparable with the values of conventional fluorescent materials. Meanwhile, its nonradiative decay rate from the S1 state (knr,S) was determined to be 9.11 × 106 s−1. As for DMAC-1DPS, kr,S and knr,S were estimated to be 0.97 × 107 s−1 and 6.17 × 106 s−1, respectively. Although there is a slight difference in kr,S for DMAC-1DPS and SBA-2DPS, the ratios of kr,S to knr,S for both emitters are similar (1.50 for SBA-2DPS and 1.56 for DMAC-1DPS), leading to similar PLQY values. Moreover, DMAC-1DPS and SBA-2DPS exhibited an effective RISC process with the corresponding rates (kRISCs) of 7.37 × 105 s−1 and 5.38 × 105 s−1, respectively, consistent with their small ΔEST.39
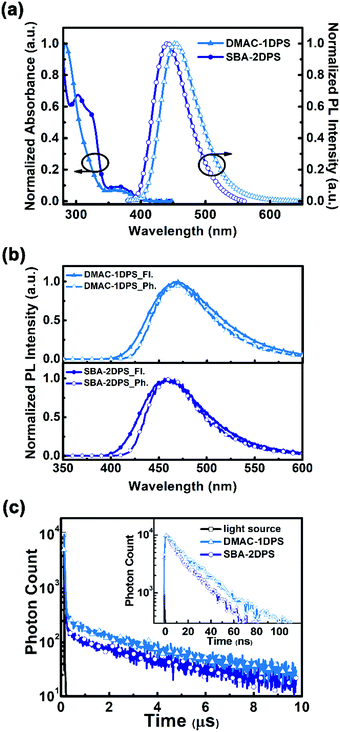 |
| Fig. 4 (a) Normalized UV-vis absorption spectra and PL spectra measured in toluene solutions (1 × 10−5 M) at room temperature. (b) Normalized fluorescence (300 K) and phosphorescence (77 K) spectra of DMAC-1DPS and SBA-2DPS in DPEPO films with 30 wt% concentration. (c) Transient PL spectra of DMAC-1DPS and SBA-2DPS in DPEPO films with 30 wt% concentration at room temperature. | |
Table 2 The summary of the photophysical properties of DMAC-1DPS and SBA-2DPS
Compound |
λ
abs
[nm] |
λ
em
[nm] |
λ
em
[nm] |
τ
p
[ns] |
τ
d
[μs] |
Φ
PL
[%] |
Φ
PF
[%] |
Φ
DF
[%] |
S1/T1e [eV] |
ΔESTf [eV] |
Θ
‖
[%] |
Measured in toluene (1 × 10−5 M) at room temperature.
Measured in doped DPEPO films (with 30 wt% doping concentration).
Lifetime of the prompt and delayed component in transient PL measured in doped DPEPO films (with 30 wt% doping concentration).
Photoluminescence quantum yield, the quantum yield of the prompt fluorescence and delayed fluorescence measured in doped DPEPO films (with 30 wt% doping concentration) at room temperature.
Obtained from the onset of the fluorescence spectra and phosphorescence spectra doped DPEPO films of these emitters.
Calculated from S1 and T1.
Horizontal dipole ratio measured in doped DPEPO films (with 30 wt% doping concentration) at room temperature.
|
DMAC-1DPS
|
283 |
452 |
468 |
20.4 |
4.2 |
61 |
20 |
41 |
2.98/2.91 |
0.07 |
59 |
SBA-2DPS
|
303/367 |
442 |
459 |
19.0 |
4.3 |
60 |
26 |
34 |
3.04/2.95 |
0.09 |
87 |
In order to study their emitting dipole orientations, the angle- and polarization-resolved PL spectra of SBA-2DPS and DMAC-1DPS (30 wt% doped in the DPEPO host) were characterized. The p-polarized PL intensities versus emission angles for both emitters measured at the PL peak wavelength are shown in Fig. 5a and c. In comparison with the simulated curves with different horizontal dipole ratios Θ‖(Θ‖ = 100% represents the fully horizontal dipole orientation and Θ‖ = 67% represents the isotropic dipole orientation), the Θ‖ of both emitters were extracted as 87% and 59% for SBA-2DPS and DMAC-1DPS, respectively. These results clearly established that the SBA-2DPS exhibited a preferentially horizontal emitting dipole orientation in the DPEPO host; in contrast, the emitting dipole of DMAC-1DPS tended to orient more randomly (slightly vertically) in the DPEPO host. To gain deeper insight into the relationship between their Θ‖ and molecular structure, TDMs of SBA-2DPS and DMAC-1DPS were simulated with the nuclear ensemble approach based on the optimized S1 state geometries (Fig. 5b and d).40 Although the TDM vectors mainly aligned in the D–A direction for both emitters, smaller components in the y–z plane were observed for SBA-2DPS, suggesting that the TDM orientation of SBA-2DPS is more aligned to the molecular axis. As SBA-2DPS tends to lie upon the substrate in parallel with the longest axis due to its rod-like shape, the TDMs achieve a preferentially horizontal orientation. Therefore, it is reasonable to attribute the high Θ‖ of SBA-2DPS to the synergetic result of elongating molecular shape and tuning TDM direction. Besides, the high Tg (176 °C) of SBA-2DPS may also contribute to its high Θ‖ by decreasing the surface mobility and orientational rearrangement during the vacuum deposition.41–43 The Θ‖ of SBA-2DPS in the DPEPO host as a function of doping concentration is also shown in Fig. S2 (ESI†). With the doping concentration varying from 12 wt% to 50 wt%, the Θ‖ only showed a slight change from 86% to 89%. This suggests that the horizontal emitting dipole orientation is the intrinsic characteristic of SBA-2DPS. Overall, high Θ‖ was achieved for the blue emitter SBA-2DPS and could be useful in promoting the optical out-coupling efficiency in OLEDs.
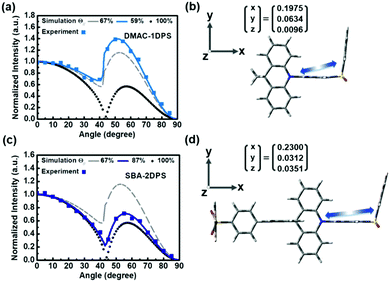 |
| Fig. 5 Measured (symbols) p-polarized PL intensity (at PL peak wavelength) versus emission angle curves for emitting layers (a) DPEPO: 30 wt% DMAC-1DPS (c) DPEPO: 30 wt% SBA-2DPS, and simulated curves for the fully horizontal dipole orientation (Θ‖ = 100%) and the isotropic dipole orientation (Θ‖ = 67%). The direction of the calculated S0–S1 transition dipole moment (as indicated by the arrow) of (b) DMAC-1DPS and (d) SBA-2DPS relative to the coordinates of the molecular structure. | |
Electroluminescence properties
To evaluate the performance of SBA-2DPS upon electrical excitation, we fabricated multilayer OLEDs with structures consisting of indium tin oxide (ITO)/MoO3 (1 nm)/di-[4-(N,N-ditolyl-amino)-phenyl]-cyclohexane (TAPC) (40 nm)/N,N-dicarbazolyl-3,5-benzene (mCP) (10 nm)/bis(2-(diphenylphosphino)phenyl)ether oxide (DPEPO): 30 wt% SBA-2DPS (20 nm)/tris-[3-(3-pyridyl)mesityl]borane (3TPYMB) (50 nm)/LiF (1 nm)/Al, as shown in Fig. 6a. ITO and Al served as the anode and the cathode, respectively. MoO3 and LiF were used as hole and electron-injection layers, respectively. TAPC and mCP served as hole-transporting layers (HTLs). DPEPO was employed as the host owing to its high energy level of T1.37 3TPYMB was the electron-transporting layer (ETL). For comparison, the reference device with an identical structure but DMAC-1DPS as a TADF dopant was also fabricated and tested.
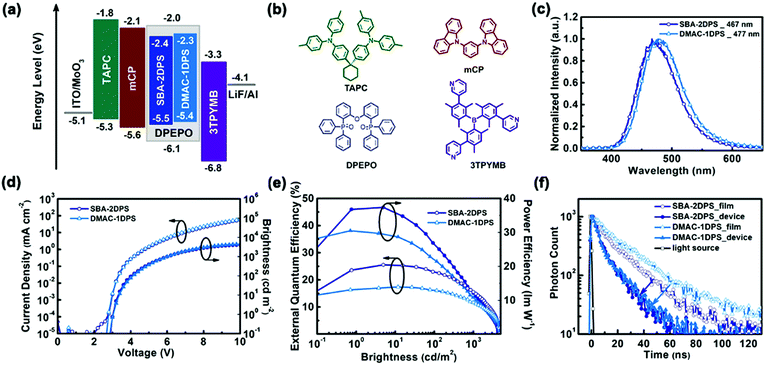 |
| Fig. 6 (a) The energy level diagrams of the devices. (b) Chemical structures of the materials used. (c–e) Electroluminescence spectra, current–voltage–luminance characteristics, external quantum efficiency and power efficiency versus luminance curves of devices based on SBA-2DPS and DMAC-1DPS. (f) The transient PL decay curves of SBA-2DPS and DMAC-1DPS measured in the single-layer doped films and the complete devices. | |
The representative EL characteristics of SBA-2DPS- and DMAC-1DPS-based devices are shown in Fig. 6c–e, and the key EL data are summarized in Table 3. As shown in Fig. 6c, the EL spectra of SBA-2DPS and DMAC-1DPS were similar to their PL spectra, with their emission peaks slightly red-shifting to 467 nm and 477 nm, respectively, corresponding to favorable Commission International de l’Eclairage coordinates of (0.15, 0.20) for SBA-2DPS and (0.16, 0.26) for DMAC-1DPS. Only the emission originating from SBA-2DPS or DMAC-1DPS was observed in the EL spectra, indicating the efficient energy transfer from the host to the TADF dopants. The turn-on voltages of both devices were as low as 2.8 V. DMAC-1DPS-based devices achieved a maximum current efficiency (ηc) of 31.0 cd A−1, a maximum power efficiency (ηp) of 30.5 lm W−1 and a maximum ηext of 17.4% as shown in Fig. 6d and e. In comparison, devices based on SBA-2DPS showed a much superior performance with a maximum ηc of 38.1 cd A−1, a maximum ηp of 37.4 lm W−1 and a maximum ηext of 25.5%. To the best of our knowledge, the high ηext of SBA-2DPS is superior to most reported TADF devices with coordinate y values ≤ 0.2.28,29,44 Considering that the TADF characteristics and PLQY of SBA-DPS are similar to those of DMAC-1DPS, the superior performance of SBA-DPS-based devices could be attributed to their higher horizontal dipole ratio (Θ‖ = 87%), which will boost the optical out-coupling efficiency (Φout) of the devices. Indeed, the Φout values were estimated to be 38.2% and 24.7% for SBA-DPS and DMAC-1DPS-based devices (Table 3) by using the classical oscillating dipole model and Θ‖, which indicates the great importance of the horizontal dipole orientation in improving Φout. In this case, the upper limit of ηexts can be calculated by ηext = ΦPL × Φout by assuming perfect carrier balance and unit exciton utilization in eqn (1). Unexpectedly, the theoretically predicted upper limits of ηext (22.9% for SBA-2DPS devices and 15.1% for DMAC-1DPS devices, Table 3) were slightly lower than their actual experimental maximum ηexts, indicating that additional mechanisms may contribute to the obtained device performance. Some previous studies have revealed that the effective emission quantum yield (qeff) of an emitter in the device configuration may exceed the measured ΦPL, owing to the modification of the spontaneous radiative transition rate by the dipole-field interaction induced by the optical microcavity effect.45–47 To verify the influence of optical microcavities on our system, the transient PL decay curves of SBA-2DPS and DMAC-1DPS in the completed device configurations were obtained. As shown in Fig. 6f, the prompt decay lifetimes significantly decreased from 19.0 ns (in the thin film) to 13.0 ns (in the device configuration) for SBA-2DPS and 20.4 ns to 12.1 ns for DMAC-1DPS. These results confirm the non-negligible effect of optical microcavity on enhancing the quantum yield of these emitters. To further estimate qeff in the device, the Purcell factor (F), defined as the modification ratio of the emitter radiative transition rate in the device to that in the thin-film configuration, was simulated to be 1.48 for SBA-2DPS and 1.71 for DMAC-1DPS (Table 3).48–50 According to previous studies,20,46qeff values were then calculated to be 68.9% for SBA-2DPS and 72.8% for DMAC-1DPS, which surpass their thin-film ΦPL values measured on the fused silica substrate. Correspondingly, the upper limit ηext values were recalculated with the effective emission quantum yield (qeff × Φout = 26.3% for SBA-2DPS-based devices and 18.0% for DMAC-1DPS-based devices), which were slightly higher than the experimental values. Thus, it is reasonable to regard the optical microcavity effect as another underlying mechanism that contributes to the high EL performance of SBA-2DPS-based devices, in addition to the preferentially horizontal emitting dipole orientation. Nevertheless, a much superior EL performance was achieved for molecule SBA-2DPS compared to its prototypical molecule DMAC-1DPS. This demonstrates the validity of the spiro-linked double D–A molecular architecture on constructing excellent TADF emitters with a preferentially horizontal emitting dipole orientation for highly efficient blue OLEDs.
Table 3 The key electroluminescence data
Device |
V
on
[V] |
ELpeak [nm] |
η
c
[cd A−1] |
η
p
[lm W−1] |
η
ext
[%] |
Φ
out
[%] |
Φ
PL
Φ
out [%] |
F
|
q
eff
[%] |
q
eff
Φ
out [%] |
CIE (x,y) |
The turn-on voltage recorded at a brightness of 0.1 cd m−2.
The maximum value, values at 100 and 1000 cd m−2.
The calculated optical out-coupling efficiency.
The calculated Purcell factor in the device configuration relative to that in thin films.
The calculated effective radiative quantum efficiency in the device configuration.
|
SBA-2DPS
|
2.8 |
467 |
38.1, 34.1, 23.5 |
37.4, 26.4, 12.3 |
25.5, 22.9, 15.6 |
38.2 |
22.9 |
1.48 |
68.9 |
26.3 |
0.15, 0.20 |
DMAC-1DPS
|
2.8 |
477 |
31.0, 29.0, 20.9 |
30.5, 21.8, 10.9 |
17.4, 16.2, 11.6 |
24.7 |
15.1 |
1.71 |
72.8 |
18.0 |
0.16, 0.26 |
Conclusions
In summary, a spiro-linked double D–A molecular architecture has been introduced to construct blue TADF emitters with a preferentially horizontal emitting dipole orientation. Using this strategy, a rod-liked TADF emitter, namely SBA-2DPS, has been designed and synthesized. Compared with the prototypical molecule DMAC-1DPS, SBA-2DPS exhibited a much elongated configuration while maintaining relatively high S1/T1 energy levels owing to the σ-spacer linkage. Favourably, SBA-2DPS exhibited higher thermal stability, maintained the PLQY and showed a distinct TADF feature. Most importantly, SBA-2DPS also showed a preferentially horizontal emitting dipole orientation with a high Θ‖ of 87%. Compared with DMAC-1DPS-based devices, blue OLEDs employing SBA-2DPS as the emitter exhibited a significantly higher ηext value of 25.5% with CIE coordinates of (0.15, 0.20) in pure blue, suggesting the great contribution of the horizontal emitting dipole orientation to enhancing the OLED performance. Further analysis has revealed that the optical microcavity effect also played an important role in boosting the internal quantum efficiency of SBA-2DPS in devices and thus the device performance. Overall, this work demonstrated the great potential of spiro-linked double D–A emitters in achieving highly efficient blue OLEDs. Further work on developing deep-blue TADF emitters with a higher Θ‖ and a higher PLQY using this strategy is in progress.
Experimental section
All reagents were used as received from commercial sources and used as received unless otherwise stated. 1-Bromo-4-(phenylsulfonyl)benzene51 and 9,9′(10H,10′H)-spirobiacridines (SBA)52 were synthesized according to the previous literature.
Synthesis of 9,9-dimethyl-10-(4-(phenylsulfonyl)phenyl)-9,10-dihydroacridine (DMAC-1DPS)
1-Bromo-4-(phenylsulfonyl)benzene (977 mg, 3.3 mmol), 9,9-dimethyl-9,10-dihydroacridine (627 mg, 3.0 mmol), sodium tert-butoxide (317 mg, 3.3 mmol), palladium(II) acetate (20 mg, 0.09 mmol) and tri-tert-butylphosphine tetrafluoroborate (78 mg, 0.27 mmol) were dissolved in dry toluene (15 mL) under an argon atmosphere. After stirring at 110 °C for 24 hours, the suspension was cooled to 298 K, mixed thoroughly with dichloromethane and washed with water three times. After drying with anhydrous Na2SO4, the organic phase was concentrated and the crude product was purified by column chromatography on silica gel with petroleum ether/dichloromethane (2
:
1 by vol.) as the eluent. Finally, the product was obtained as a white powder (1.16 g, yield: 91%). 1H NMR (400 MHz, CDCl3 + TMS, 298 K) δ (ppm): 8.17 (d, J = 8 Hz, 2H), 8.07 (d, J = 8 Hz, 2H), 7.68–7.58 (m, 3H), 7.51–7.46 (m, 4H), 7.00–6.95 (m, 4H), 6.27–6.23 (m, 2H), 1.66 (s, 6H). 13C NMR (100 MHz, CDCl3, 298 K) δ (ppm): 146.4, 141.3 140.6, 140.2, 133.5, 131.4, 131.0, 130.4, 129.5, 127.9, 126.4, 125.4, 121.6, 114.8, 36.2, 30.9. HRMS (electrospray ionization (ESI)): m/z [M + H]+ calcd for C27H24NO2S+: 426.1522; found: 426.1522.
Synthesis of 10,10′-bis(4-(phenylsulfonyl)phenyl)-10H,10′H-9,9′-spirobiacridine (SBA-2DPS)
SBA-2DPS was prepared according to a similar procedure to DMAC-1DPS. Yield: 87%. 1H NMR (400 MHz, CDCl3 + TMS, 298 K) δ (ppm): 8.28 (d, J = 8 Hz, 4H), 8.10 (d, J = 8 Hz, 4H), 7.69–7.60 (m, 10H), 7.09 (d, J = 8 Hz, 4H), 6.87 (t, J = 8 Hz, 4H), 6.75 (t, J = 8 Hz, 4H), 6.14 (d, J = 8 Hz, 4H). 13C NMR (100 MHz, CDCl3, 298 K) δ (ppm): 146.0, 141.8, 141.0, 137.7, 133.7, 132.8, 132.8, 131.7, 130.7, 129.6, 128.1, 126.8, 121.5, 113.8, 46.7. HRMS (ESI): m/z [M + H]+ calcd for C49H35N2O4S2+: 779.2033; found: 779.2013.
Author contributions
X. Zeng, S. Gong, C.-C. Wu and C. Yang wrote the manuscript. X. Zeng, X. Xiao and L. Zhan synthesized the compounds. X. Zeng measured the photophysical, thermal and electrochemical properties of the compounds. K.-C. Pan, W.-K. Lee and C.-C. Wu fabricated and characterized the devices. F. Ni, S. Gong and C. Yang conceived the original idea for investigation. Y. Xiang and Y. Zhang performed the X-ray single crystal diffraction analysis. W. Zeng performed the quantum chemical calculations. All authors discussed the progress of research and reviewed the manuscript.
Conflicts of interest
There are no conflicts to declare.
Acknowledgements
The authors gratefully acknowledge financial support from the National Key R&D Program of China (2016YFB0401002), the National Natural Science Foundation of China (51873158, 51573141, 91833304 and 91433201), the National Basic Research Program of China (973 Program 2015CB655002), the Shenzhen Peacock Plan (KQTD20170330110107046), the Key Technological Innovation Program of Hubei Province (2018AAA013), and the Natural Science Foundation for Distinguished Young Scholars of Hubei Province (2017CFA033). C. C. Wu acknowledges the support from the Ministry of Science and Technology of Taiwan (MOST 105-2221-E-002-162-MY3 and 107-2221-E-002-160-MY3). The numerical calculations in this paper have been done using the supercomputing system at the Supercomputing Center of Wuhan University.
Notes and references
- M. C. Gather, A. Köhnen and K. Meerholz, Adv. Mater., 2011, 23, 233–248 CrossRef CAS PubMed.
- T. Sekitani and T. Someya, Adv. Mater., 2010, 22, 2228–2246 CrossRef CAS PubMed.
- C. W. Tang and S. A. VanSlyke, Appl. Phys. Lett., 1987, 51, 913–915 CrossRef CAS.
- M. A. Baldo, D. F. O'Brien, Y. You, A. Shoustikov, S. Sibley, M. E. Thompson and S. R. Forrest, Nature, 1998, 395, 151–154 CrossRef CAS.
- J. Partee, E. L. Frankevich, B. Uhlhorn, J. Shinar, Y. Ding and T. J. Barton, Phys. Rev. Lett., 1999, 82, 3673–3676 CrossRef CAS.
- H. Uoyama, K. Goushi, K. Shizu, H. Nomura and C. Adachi, Nature, 2012, 492, 234–238 CrossRef CAS PubMed.
- Y. Xu, X. Liang, X. Zhou, P. Yuan, J. Zhou, C. Wang, B. Li, D. Hu, X. Qiao, X. Jiang, L. Liu, S.-J. Su, D. Ma and Y. Ma, Adv. Mater., 2019, 31, 1807388 CrossRef PubMed.
- T. Tsutsui, E. Aminaka, C. P. Lin and D.-U. Kim, Philos. Trans. R. Soc., A, 1997, 355, 801–814 CrossRef CAS.
- K. Meerholz and D. C. Müller, Adv. Funct. Mater., 2001, 11, 251–253 CrossRef CAS.
- M. A. Baldo, D. F. O’Brien, M. E. Thompson and S. R. Forrest, Phys. Rev. B: Condens. Matter Mater. Phys., 1999, 60, 14422–14428 CrossRef CAS.
- Y. Tao, K. Yuan, T. Chen, P. Xu, H. Li, R. Chen, C. Zheng, L. Zhang and W. Huang, Adv. Mater., 2014, 26, 7931–7958 CrossRef CAS PubMed.
- N. A. Kukhta, T. Matulaitis, D. Volyniuk, K. Ivaniuk, P. Turyk, P. Stakhira, J. V. Grazulevicius and A. P. Monkman, J. Phys. Chem. Lett., 2017, 8, 6199–6205 CrossRef CAS PubMed.
- A. Tsuboyama, H. Iwawaki, M. Furugori, T. Mukaide, J. Kamatani, S. Igawa, T. Moriyama, S. Miura, T. Takiguchi, S. Okada, M. Hoshino and K. Ueno, J. Am. Chem. Soc., 2003, 125, 12971–12979 CrossRef CAS PubMed.
- Q. Zhang, B. Li, S. Huang, H. Nomura, H. Tanaka and C. Adachi, Nat. Photonics, 2014, 8, 326–332 CrossRef CAS.
- Y. Tao, C. Yang and J. Qin, Chem. Soc. Rev., 2011, 40, 2943–2970 RSC.
- L. Xiao, Z. Chen, B. Qu, J. Luo, S. Kong, Q. Gong and J. Kido, Adv. Mater., 2011, 23, 926–952 CrossRef CAS PubMed.
- W.-Y. Wong and C.-L. Ho, J. Mater. Chem., 2009, 19, 4457–4482 RSC.
- K.-C. Pan, S.-W. Li, Y.-Y. Ho, Y.-J. Shiu, W.-L. Tsai, M. Jiao, W.-K. Lee, C.-C. Wu, C.-L. Chung, T. Chatterjee, Y.-S. Li, K.-T. Wong, H.-C. Hu, C.-C. Chen and M.-T. Lee, Adv. Funct. Mater., 2016, 26, 7560–7571 CrossRef CAS.
- K. Wu, T. Zhang, Z. Wang, L. Wang, L. Zhan, S. Gong, C. Zhong, Z.-H. Lu, S. Zhang and C. Yang, J. Am. Chem. Soc., 2018, 140, 8877–8886 CrossRef CAS PubMed.
- W. Zeng, H.-Y. Lai, W.-K. Lee, M. Jiao, Y.-J. Shiu, C. Zhong, S. Gong, T. Zhou, G. Xie, M. Sarma, K.-T. Wong, C.-C. Wu and C. Yang, Adv. Mater., 2018, 30, 1704961 CrossRef PubMed.
- S.-Y. Kim, W.-I. Jeong, C. Mayr, Y.-S. Park, K.-H. Kim, J.-H. Lee, C.-K. Moon, W. Brütting and J.-J. Kim, Adv. Funct. Mater., 2013, 23, 3896–3900 CrossRef CAS.
- D. H. Ahn, S. W. Kim, H. Lee, I. J. Ko, D. Karthik, J. Y. Lee and J. H. Kwon, Nat. Photonics, 2019, 13, 540–546 CrossRef CAS.
- T.-A. Lin, T. Chatterjee, W.-L. Tsai, W.-K. Lee, M.-J. Wu, M. Jiao, K.-C. Pan, C.-L. Yi, C.-L. Chung, K.-T. Wong and C.-C. Wu, Adv. Mater., 2016, 28, 6976–6983 CrossRef CAS PubMed.
- C. Mayr, S. Y. Lee, T. D. Schmidt, T. Yasuda, C. Adachi and W. Brütting, Adv. Funct. Mater., 2014, 24, 5232–5239 CrossRef CAS.
- K.-H. Kim and J.-J. Kim, Adv. Mater., 2018, 30, 1705600 CrossRef PubMed.
- D. Yokoyama, J. Mater. Chem., 2011, 21, 19187–19202 RSC.
- M. Liu, R. Komatsu, X. Cai, K. Hotta, S. Sato, K. Liu, D. Chen, Y. Kato, H. Sasabe, S. Ohisa, Y. Suzuri, D. Yokoyama, S.-J. Su and J. Kido, Chem. Mater., 2017, 29, 8630–8636 CrossRef CAS.
- T.-T. Bui, F. Goubard, M. Ibrahim-Ouali, D. Gigmes and F. Dumur, Appl. Sci., 2018, 8, 494 CrossRef.
- M. Y. Wong and E. Zysman-Colman, Adv. Mater., 2017, 29, 1605444 CrossRef PubMed.
- F. B. Dias, T. J. Penfold and A. P. Monkman, Methods Appl. Fluoresc., 2017, 5, 012001 CrossRef PubMed.
- A. Pershin, D. Hall, V. Lemaur, J.-C. Sancho-Garcia, L. Muccioli, E. Zysman-Colman, D. Beljonne and Y. Olivier, Nat. Commun., 2019, 10, 597 CrossRef PubMed.
- M. K. Etherington, J. Gibson, H. F. Higginbotham, T. J. Penfold and A. P. Monkman, Nat. Commun., 2016, 7, 13680 CrossRef CAS PubMed.
- T. Lu and F. Chen, J. Mol. Graphics Modell., 2012, 38, 314–323 CrossRef CAS PubMed.
- C. S. Oh, C.-K. Moon, J. M. Choi, J.-S. Huh, J.-J. Kim and J. Y. Lee, Org. Electron., 2017, 42, 337–342 CrossRef CAS.
- C. Fan, Y. Chen, Z. Liu, Z. Jiang, C. Zhong, D. Ma, J. Qin and C. Yang, J. Mater. Chem. C, 2013, 1, 463–469 RSC.
- P. L. dos Santos, J. S. Ward, D. G. Congrave, A. S. Batsanov, J. Eng, J. E. Stacey, T. J. Penfold, A. P. Monkman and M. R. Bryce, Adv. Sci., 2018, 5, 1700989 CrossRef PubMed.
- C. Han, Y. Zhao, H. Xu, J. Chen, Z. Deng, D. Ma, Q. Li and P. Yan, Chem. – Eur. J., 2011, 17, 5800–5803 CrossRef CAS PubMed.
- I. S. Park, S. Y. Lee, C. Adachi and T. Yasuda, Adv. Funct. Mater., 2016, 26, 1813–1821 CrossRef CAS.
- X. Cai, X. Li, G. Xie, Z. He, K. Gao, K. Liu, D. Chen, Y. Cao and S.-J. Su, Chem. Sci., 2016, 7, 4264–4275 RSC.
- W. Zeng, S. Gong, C. Zhong and C. Yang, J. Phys. Chem. C, 2019, 123, 10081–10086 CrossRef CAS.
- S. S. Dalal and M. D. Ediger, J. Phys. Chem. Lett., 2012, 3, 1229–1233 CrossRef CAS.
- C. Mayr and W. Brütting, Chem. Mater., 2015, 27, 2759–2762 CrossRef CAS.
- D. Yokoyama, A. Sakaguchi, M. Suzuki and C. Adachi, Appl. Phys. Lett., 2008, 93, 173302 CrossRef.
- X.-L. Chen, J.-H. Jia, R. Yu, J.-Z. Liao, M.-X. Yang and C.-Z. Lu, Angew. Chem., Int. Ed., 2017, 129, 15202–15205 CrossRef.
- A. Dodabalapur, L. J. Rothberg, T. M. Miller and E. W. Kwock, Appl. Phys. Lett., 1994, 64, 2486–2488 CrossRef CAS.
- T. D. Schmidt, D. S. Setz, M. Flämmich, J. Frischeisen, D. Michaelis, B. C. Krummacher, N. Danz and W. Brütting, Appl. Phys. Lett., 2011, 99, 163302 CrossRef.
- N. Takada, T. Tsutsui and S. Saito, Appl. Phys. Lett., 1993, 63, 2032–2034 CrossRef CAS.
- H. Becker, S. E. Burns and R. H. Friend, Phys. Rev. B: Condens. Matter Mater. Phys., 1997, 56, 1893–1905 CrossRef CAS.
- X.-W. Chen, W. C. H. Choy, C. J. Liang, P. K. A. Wai and S. He, Appl. Phys. Lett., 2007, 91, 221112 CrossRef.
- B. C. Krummacher, S. Nowy, J. Frischeisen, M. Klein and W. Brütting, Org. Electron., 2009, 10, 478–485 CrossRef CAS.
- G. Zhou, Q. Wang, X. Wang, C.-L. Ho, W.-Y. Wong, D. Ma, L. Wang and Z. Lin, J. Mater. Chem., 2010, 20, 7472–7484 RSC.
- M. Ooishi, M. Seino, R. Imachi, T. Ishida and T. Nogami, Tetrahedron Lett., 2002, 43, 5521–5524 CrossRef CAS.
Footnote |
† Electronic supplementary information (ESI) available: Material synthesis, X-ray structural analysis, photophysical characterization, device fabrication and measurements, DSC curves, analysis of rate constants, and 1H and 13C NMR spectra. CCDC 1868667 and 1868668. For ESI and crystallographic data in CIF or other electronic format see DOI: 10.1039/c9tc03582f |
|
This journal is © The Royal Society of Chemistry 2019 |