Targeted hexagonal Pd nanosheet combination therapy for rheumatoid arthritis via the photothermal controlled release of MTX†
Received
1st September 2018
, Accepted 10th November 2018
First published on 27th November 2018
Abstract
Methotrexate (MTX) is a drug that is used for the clinical treatment of rheumatoid arthritis (RA), a stubborn disease caused by over-immunization. However, the toxicity that arises as a result of poor selectivity to inflammatory cells severely limits the application of MTX. Therefore, new therapeutic strategies are needed for treating RA. Here, we describe the design and synthesis of a nanotherapy agent, Pd-Cys@MTX@RGD, which can target inflammatory cells and control MTX release. The novel hexagonal palladium (Pd) nanosheets were used as a near-infrared (NIR) photothermal agent modified with arginine-glycineaspartic acid (RGD) peptides on the surface to enhance the ability of the nanosheet targeting of inflammatory cells. In subsequent experiments, the Pd-Cys@MTX@RGD nanosheets were observed to greatly reduce the toxicity of MTX, showing controlled MTX release under irradiation of 808 nm (0.3 W cm−2). Moreover, taking advantage of the fact that MTX can be combined with multiple therapeutic methods, the photothermal therapy (PTT) of Pd nanosheets provided a compensatory effect to enhance the therapeutic efficacy of MTX. Under combination therapy, Pd-Cys@MTX@RGD was shown to effectively inhibit the inflammatory response induced by vascular endothelial growth factor (VEGF) and IL-1β. And, in vivo, multifunctional Pd-Cys@MTX@RGD effectively inhibited the symptoms of RA by inhibiting the expression of pro-inflammatory cytokines (TNF-α,COX-2). We hope that the construction of nanomaterials can add potential value to the design of chemical drugs and therapeutic strategies for RA.
1. Introduction
Rheumatoid arthritis (RA) is an autoimmune disease that results in both the structural deformation and degeneration of joint tissues.1–3 Today, the clinical treatment of RA mainly focuses on the use of chemical drugs, with methotrexate (MTX) being the main drug of choice.4–6 However, with widespread use, the dose of MTX monotherapy for RA treatment has shown an increasing trend.6–8 The data showed current recommendations for MTX monotherapy suggest initiation of 15 mg per week orally, and will increase rapidly to 25–30 mg per week or the highest tolerable dose with the treatment.4,5,8 Despite its good therapeutic efficacy, the long-term administration of MTX may cause serious side effects, including infection, hepatitis, and bone marrow suppression. Therefore, to overcome these issues and reduce the dose dependency, it is necessary to adopt an appropriate strategy that can both effectively treat the symptoms of RA and reduce the toxicity of MTX by allowing the dose to be reduced.
Recently, functional drug-delivery systems (DDSs) based on various functional nanoparticles have been widely explored for applications in some conditions, such as cancer9–12 and bacterial infections.13–15 Multifunctional delivery not only allows the function of the drug carrier, improving the biocompatibility and release of the drug, but also allows combination therapy.9,16,17 Many research studies have shown that the therapeutic efficiency of MTX can be enhanced by combining it with other therapeutic methods.17–19 Therefore, we have designed a nanocarrier that can deliver a low-dose of MTX with a low risk of toxic side effects, but also has a highly therapeutic effect. Among the many delivery methods, nanomaterials combined with photothermal therapy provide a novel strategy for treating disease. Near-infrared (NIR) resonant nanomaterials, such as Au nanomaterials20–23 and carbon nanotubes,24–26 have been widely studied because they strongly absorb NIR light and produce localized cytotoxic heat under irradiation. In 2011, Zheng's group prepared novel ultra-thin hexagonal palladium nanosheets, which they named palladium blue.20,27–29 These highly dispersed hexagonal ultra-thin palladium nanosheets exhibited an adjustable localized surface plasmon resonance (LSPR) peak and good photothermal stability in the near-infrared region, and showed outstanding photothermal therapy effects in in vitro cell experiments. However, these inorganic photothermal nanomaterials are limited by their lack of biocompatibility and targeting ability. And, so far, many studies using these nanomaterials have been mainly focused on cancer treatments.
Therefore, in order to overcome the low targeting ability of MTX to the site of inflammation, we used a RGD polypeptide, which binds Rvβ3 integrins expressed on angiogenic vascular endothelial cells30,31 at sites of inflammation, as the modifier to enhance the targeting ability of Pd nanosheets towards inflammatory cells (Scheme 1). The photothermal agent Pd nanosheets loaded with low dose MTX and modified with the RGD peptide was used to obtain a multifunctional nanotherapy system that not only effectively reduced the side effects of MTX by allowing a low loading dose, but also enhanced the therapeutic effect towards RA by combining it with photothermal therapy.
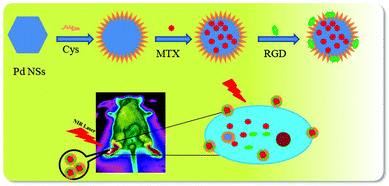 |
| Scheme 1 An illustration of the process of the synthesis of the Pd-Cys@MTX@RGD nanosheets and it being used in the therapy of RA mice under 808 nm irradiation. | |
2. Materials and methods
The chemicals were purchased from Macklin reagent, all of which were reagent grade and used without further purification, unless otherwise indicated. The TNF-α and COX-2 antibodies were purchased from Bioss. Reagent grade water was obtained using a Milli-Q water purification system (Millipore, Bedford, USA).
2.1 Preparation of Pd and Pd-Cys@MTX@RGD nanosheets
The Pd nanosheets were synthesized according to a previously reported method.32 In detail, palladium acetylacetonate (20 mg), polyvinylpyrrolidone (PVP, MW = 35
000, 70 mg) and sodium bromide (62 mg) were dissolved in 8 mL of N,N-dimethylacetamide (DMAC). Water (4 mL) was added into the solution, which was then stirred for 24 h. After stirring, CO was then added to the solution, which was then heated to 100 °C and stirred for 3 h. The colour of the solution became black, indicating that the reaction was complete. The solution was then washed and collected by washing three times with acetone, ethanol and water and centrifuging at 10
000 rmp.
The palladium nanosheets (20 mg) and cysteine (Cys, 80 mg) were mixed in ultra-pure water, then sonicated for 10 minutes and stirred for 5 h. After stirring, the Cys-modified Pd nanosheets were washed and collected by centrifuging at 10
000 rmp. Then, 1 mL of MTX (50 μg mL−1) was added to 10 mL of the Pd-Cys solution (2 mg mL−1) and sonicated for 2 h, after which the resulting precipitate was collected by centrifugation. The precipitate was then dissolved in 10 mL of PBS and mixed with 10 mg of the RGD peptide. The precipitate was collected by centrifugation to obtain the Pd-Cys@MTX@RGD nanosheets, which were washed three times and then freeze-dried. The nanosheets were characterized using transmission electron microscopy (TEM), and Fourier-transform infrared (FTIR) and ultraviolet-visible (UV-vis) spectroscopies.
2.2 Detecting the photothermal effect
Different concentrations of Pd (10, 5, 2.5 μg mL−1) and Pd-Cys@MTX@RGD nanosheet (10 μg mL−1) solutions were prepared. 1 mL of solution was placed in a quartz cuvette and irradiated for 10 min with an 808 nm laser (0.3 W cm−2). The change in temperature was recorded at different times using an infrared thermal imaging camera. To evaluate the photothermal stability of Pd and the Pd-Cys@MTX@RGD nanosheets, 1 mL solutions of the Pd (10 μg mL−1) and Pd-Cys@MTX@RGD nanosheets (10 μg mL−1) were exposed to the 808 nm laser (0.3 W cm−2) for 10 min, after which time the laser was turned off. Once the solution became cool, the solution was re-exposed to the laser again. The change in temperature was recorded while repeating this process 10 times.
2.3 Detecting the MTX release from the nanosheets
To evaluate the MTX release behavior of Pd-Cys@MTX and Pd-Cys@MTX@RGD, 1 mL aqueous solutions of Pd-Cys@MTX (10 μg mL−1) and Pd-Cys@MTX@RGD (10 μg mL−1) were deposited into the wells of a 96-well cell culture plate. The wells were treated with or without 808 nm NIR irradiation, and the release of MTX was detected by UV-vis at 302 nm.
2.4 Cell cultures
The HUVEC, HEK 293T, and RAW 264.7 cells lines were purchased from the Company Limited of Science light Biology Science & Technology (Shanghai, China) and cultured in 25 cm2 flasks at 37 °C in a humidified atmosphere containing 5% CO2 in Dulbecco's modified Eagle's medium (DMEM) containing 10% fetal bovine serum (FBS) and 1% penicillin/streptomycin.
2.5 Cellular uptake
The HUVECs and HEK 293T cells were seeded in a small confocal dish at a density of 5 × 104 cells per well and cultured for 12 h. Pd-Cys@MTX and Pd-Cys@MTX@RGD nanosheets labeled with fluorescein isothiocyanate (FITC) were added to the wells and incubated for 6 h, respectively, and then observed using a confocal laser scanning microscope (CLSM) excitation wavelength of 488 nm and an emission wavelength of 560–615 nm.
2.6 MTT methods
The cell viability was measured using a colorimetric assay for 96-well plates with 2-(4-iodophenyl)-3-(4-nitrophenyl)-5-(2,4-disulfophenyl)-2H-tetrazolium monosodium salt (CCK-8) reagent. Each plate contained blanks, controls, and two substance dilution series, each with three replicates. Cells were added to the plates at a concentration of 5 × 103 cell per mL (in DMEM/F12 with 10% FBS) and cultured for 24 h. Then, 10 μL of MTX, Pd-Cys, Pd-Cys@MTX and Pd-Cys@MTX@RGD at different concentrations were added, and the other two groups Pd-Cys@MTX and Pd-Cys@MTX@RGD were incubated for 24 h under 808 nm (0.3 W cm−2) irradiation, after which 10 μL of CCK-8 was added, and the cells were incubated for an additional 1 h. The cell viability was measured at 450 nm using a microplate reader.
2.7
In vivo photoacoustic imaging
2D image acquisition was performed using a photoacoustic enhanced ultrasound Nexus 128 system (ENDRA Life Sciences Inc., Ann Arbor, Michigan USA). The RA mice were intravenously injected with 100 μL of Pd-Cys and Pd-Cys@MTX@RGD (2.5 mg kg−1) and anesthetized under isoflurane. The mice were placed into a 3D hemispherical detector after injection and then the photoacoustic (PA) signals were recorded for 6 h. The cartilage tissue was detected by a tunable laser operating at a wavelength of 650 nm.
2.8
In vivo inhibiting inflammation studies
Female DBA mice were purchased from the Guangdong Medical Experimental Animal Center, and the animal care procedures used were in accordance with the institutional guidelines and approved by the Animal Care and Use Committee of Jinan University.
Briefly, 200 μg of bovine type II collagen (2 mg mL−1, Chondrex, Redmond, WA, USA) emulsified in 100 μL of complete Freund's adjuvant (4 mg mL−1, Chondrex) was injected intradermally at the base of the tail of DBA/1 J mice. Next, a booster intradermal injection of 100 μg of bovine type II collagen in incomplete Freund's adjuvant (Condrex, Japan) was administered to mice 21 days after the primary immunization. When the arthritis was fully developed, saline (group 1), Pd-Cys nanosheets (groups 2 and 3), Pd-Cys@MTX nanosheets (group 4), or Pd-Cys@MTX@RGD nanosheets (group 5) and MTX (group 6) were intravenously administered to the mice, and groups 3, 4 and 5 were exposed to 808 nm (0.3 W cm−2) irradiation for 10 min at 1 day post intravenous injection of the nanosheets (n = 5 mice in each group). After injection, the mice were observed twice a week for 4 weeks. The clinical index is the sum of the clinical scores for the paws (maximum score = 4). The evaluated paws were scored from 0 to 4 according to the following scale: 0 = no evidence of erythema and swelling, 1 = erythema and mild swelling, 2 = erythema and mild swelling extending from the ankle to the tarsals, 3 = erythema and moderate swelling extending from the ankle to the metatarsal joints, and 4 = erythema and severe swelling encompassing the ankle, foot, and digits or ankylosis of the limb.
2.9 Histopathology evaluation
The mice were anesthetized and sacrificed at 28 days after each group treatment. The paws were extracted, fixed, decalcified and embedded in paraffin for histopathological testing. The paw tissues were sectioned (5 μm thick) and stained with hematoxylin and eosin (H&E) to observe the degree of cartilage erosion. To evaluate the level of inflammation in the paws, immunohistochemistry and immunofluorescence were used. Deparaffinized sections of joints were incubated with two specific antibodies of conjugated TNF-α and COX-2. The frozen sections (9 μm) of the extracted paws were fixed in 4% paraformaldehyde (PFA) for 30 min at 25 °C, and then incubated with rabbit anti-mouse TNF-α (1
:
500, bs-5780R, BIOSS ANTIBODIES), and rat anti-mouse COX-2 (1
:
500, bs-20449R, BIOSS ANTIBODIES). All histologic studies were preformed independently and assessed blindly by three individual assessors.
3. Results and discussion
3.1 The synthesis and characterization of the Pd-Cys@MTX@RGD nanosheets
The inset in Fig. 1 shows that the Pd nanosheets reduced by CO form a uniformly dispersed blue solution. The size of the hexagonal Pd nanosheets was determined as 24 nm by TEM (Fig. 1A) and the results were found to correspond to the results of dynamic light scattering (DLS) measurements (Fig. S1A, ESI†). From the SEM images, the morphology of the Pd and Pd-Cys nanosheets can be observed as uniform hexagons and the size of Pd-Cys can be seen to be slightly larger than that of the Pd nanosheets due to the modification by cysteine. Moreover, after loading Pd-Cys@MTX@RGD with MTX and RGD, the particle size further increased to 50 nm (Fig. S3, ESI†). After being modified with the cysteine, the morphology and size of the Pd-Cys nanosheets did not change significantly. Furthermore, to confirm the interaction between the components of Pd-Cys@MTX@RGD, FTIR and zeta potential measurements were carried out. As shown in the FTIR spectrum in Fig. 1B, for the cysteine-modified Pd nanosheets, the peak at 2550 cm−1 disappeared, indicating that the cysteine thiol (–SH) was conjugated to the Pd nanosheets. It can also be observed that the absorption peak of MTX is present in the Pd-Cys@MTX@RGD spectrum, indicating that MTX was successfully loaded on the Pd nanosheets. Moreover, the absorption peak at 1650 cm−1 shows that the –CONH2 group in Pd-Cys@MTX@RGD was enhanced after the RGD peptide was attached. Therefore, we can speculate that cysteine and RGD are linked to the Pd nanosheets through –CONH2 bonds. The zeta potential measurements gave a value of 13 mV for the Pd nanosheets and the potential was observed to be stable at −18 mV after Cys modification. However, the potential of Pd-Cys@MTX was 2 mV, due to the positive charge of MTX (Fig. S2, ESI†). Therefore, we speculate that MTX is loaded on Pd-Cys nanoparticles through electrostatic interactions. The zeta potential of Pd-Cys@MTX@RGD was observed to become −3 mV after RGD modification (Fig. 1C). Furthermore, we tested the particle size and potential changes of Pd-Cys@MTX@RGD (20 μg mL−1) in various solutions at 0, 24, 48 and 72 h, respectively. No significant change in the size and potential was observed, and furthermore, it was also found that the nanosheets did not appear to coagulate (see the photographs in Fig. S4, ESI†). Therefore, Pd-Cys@MTX@RGD was determined to be stable in solution. After calculating the concentration standard curve it was determined that the loading dose of MTX in Pd-Cys@MTX@RGD was 2.55 μg mL−1 when 5 μg mL−1 of MTX was added to the solution and the loading efficiency was 51% (Fig. 1D). And, when the Pd-Cys@MTX@RGD was irradiated at 808 nm by laser (0.3 W cm−2) it was observed that the MTX was rapidly released from the nanosheets and was completely released after 6 h (Fig. 1E).
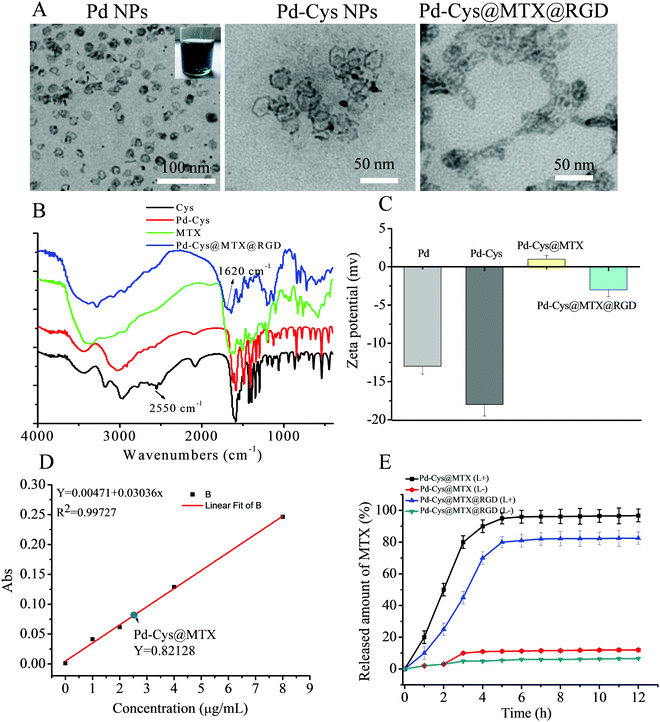 |
| Fig. 1 (A) TEM images of Pd, Pd-Cys and Pd-Cys@MTX@RGD nanosheets, (B) FTIR spectra of Cys, MTX, Pd-Cys and Pd-Cys@MTX@RGD, (C) zeta potential values of Pd, Pd-Cys, Pd-Cys@MTX and Pd-Cys@MTX@RGD nanosheets, (D) linear regression curves of different concentrations of MTX, and (E) the release of MTX from Pd-Cys@MTX and Pd-Cys@MTX@RGD with/without 808 nm (0.3 W cm−2) laser irradiation. | |
3.2 The toxicity and targeting of Pd-Cys@MTX@RGD nanosheets
In order to determine the biocompatibility of Pd-Cys@MTX@RGD nanosheets, we measured the toxicity of the Pd-Cys nanosheets, Pd-Cys@MTX, Pd-Cys@MTX@RGD and MTX to HEK 293T cells and HUVECs, detected using the CCK-8 method. It can be observed from Fig. 2 that MTX is toxic to HEK 293T cells and HUVECs in a concentration dependent manner. At a concentration of only 5 μg mL−1, the cell viability of HEK 293 T cells (Fig. 2A) and HUVECs (Fig. 2B) was 94.3% and 93.1%, respectively. Meanwhile, when the concentration was increased to 80 μg mL−1 the cell viability of the HEK 293 T cells and HUVECs reduced to 49.6% and 39.1%, respectively. In further experiments, the results of the nanocarrier Pd-Cys showed no significant toxicity to normal cells, and after being further loaded with MTX, the Pd-Cys@MTX and Pd-Cys@MTX@RGD nanosheets also showed negligible toxicity. However, the toxicity of the Pd-Cys@MTX and Pd-Cys@MTX@RGD nanosheets to HEK 293 T cells (Fig. 2A) and HUVECs (Fig. 2B) was significantly enhanced under 808 nm (0.3 W cm−2) irradiation, due to the MTX release upon irradiation. This further indicates that MTX release by the Pd-Cys nanosheets can be controlled by NIR. On the other hand, to verify the specific targeting of the Pd-Cys@MTX@RGD nanosheets to the HUVECs, we observed the cellular uptake of Pd-Cys@MTX and Pd-Cys@MTX@RGD labeled with FITC by the HEK 293 T cells and HUVECs, respectively. The results showed there was no difference between Pd-Cys@MTX and Pd-Cys@MTX@RGD in the case of the HEK 293 T cells; the fluorescence intensities of Pd-Cys@MTX and Pd-Cys@MTX@RGD were 20.3 and 23.1, respectively. However, with respect to the HUVECs, the cellular uptake of Pd-Cys@MTX@RGD was significantly stronger than that of Pd-Cys@MTX (Fig. 2C), and the fluorescence intensities of Pd-Cys@MTX and Pd-Cys@MTX@RGD were 5.8 and 45.6, respectively. Furthermore, the cell uptake efficiencies of Pd-Cys@MTX and Pd-Cys@MTX@RGD were detected by flow cytometry analysis (Fig. S5, ESI†), and the results showed that there was no obvious difference between the Pd-Cys@MTX and Pd-Cys@MTX@RGD cell uptake efficiencies towards the HEK 293 T cells, but for the HUVECs, the Pd-Cys@MTX@RGD uptake was 6.5 times greater than that of Pd-Cys@MTX. It has previously been proven that RGD in Pd-Cys@MTX@RGD can specifically target HUVECs through the binding of Rvβ3 integrins.30–33 Therefore, the Pd-Cys@MTX@RGD nanosheets effectively reduce the side effects of MTX by controlling the release of MTX and enhancing the targeting of inflammatory cells.
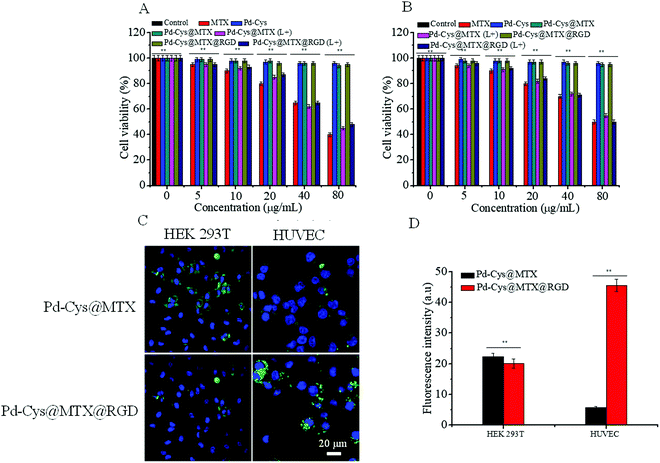 |
| Fig. 2 (A and B) The cell viability of MTX, Pd-Cys, Pd-Cys@MTX and Pd-Cys@MTX@RGD with/without 808 nm (0.3 W cm−2) irradiation. (C) Cell uptake of Pd-Cys@MTX and Pd-Cys@MTX@RGD to HEK 293T cells and HUVECs. (D) Quantification of the fluorescence intensity of Pd-Cys@MTX and Pd-Cys@MTX@RGD in the corresponding confocal microscopy images. (P* ≤ 0.05). | |
3.3 The photothermal effect and photoacoustic images of Pd nanosheets
Pd nanosheets have been used as novel therapeutic agents with good photothermal effect.20,33 In order to measure the photothermal effect of hexagonal Pd nanosheets and loaded nanoparticles after loading and modification, we used a 808 nm (0.3 W cm−2) laser for 10 min to observe the change in temperature. As shown in Fig. 3A, the Pd nanosheets (10 μg mL−1) exhibited good photothermal effect, as the temperature was observed to reach 53.8 °C after 10 min of irradiation, and showed concentration dependency (Fig. 3C), demonstrating that the hexagonal Pd nanosheets have the potential to be used in photothermal therapy. On the other hand, the same concentration of Pd-Cys@MTX@RGD nanosheets also showed a good photothermal effect, with the temperature reaching 49.7 °C after 10 min, a temperature lower than that of the Pd nanosheets, probably due to the surface modifier being able to absorb NIR. Furthermore the photothermal imaging demonstrated the photothermal effect of the Pd and Pd-Cys@MTX@RGD nanosheets. It can be observed from Fig. 3B that the temperature of the aqueous solution showed little change after 10 min of irradiation, but the Pd and Pd-Cys@MTX@RGD solution nanosheets results were contrary to those of the aqueous solution. The temperature of the Pd and Pd-Cys@MTX@RGD solutions reached 50 and 48 °C, respectively, after 808 nm irradiation for 10 min (Fig. 3C). In addition, photothermal stability is a very important property of photothermal agents. To investigate the photothermal stability of the Pd and Pd-Cys@MTX@RGD nanosheets, we measured the photothermal effect of phthalocyanine green (ICG) and the Pd and Pd-Cys@MTX@RGD nanosheets with repeated irradiation. The results showed that for the Pd and Pd-Cys@MTX@RGD nanosheets, the photothermal conversion rate of ICG showed an obvious decrease after repeated rounds of irradiation, but the Pd and Pd-Cys@MTX@RGD nanosheets remained stable, indicating that the Pd and Pd-Cys@MTX@RGD nanosheets have a stable photothermal effect (Fig. 3D). The photoacoustic imaging ability is another important property of photothermal materials. Therefore, we investigated the photoacoustic imaging of the Pd nanosheets. As shown in Fig. 3E, the photoacoustic imaging signal of the Pd nanosheets is concentration dependent. After excitation with an 808 nm laser, the Pd nanosheets showed a strong photothermal images signal at 10 μg mL−1. This indicates that the Pd nanosheets have a good, stable photothermal effect and good photoacoustic imaging.
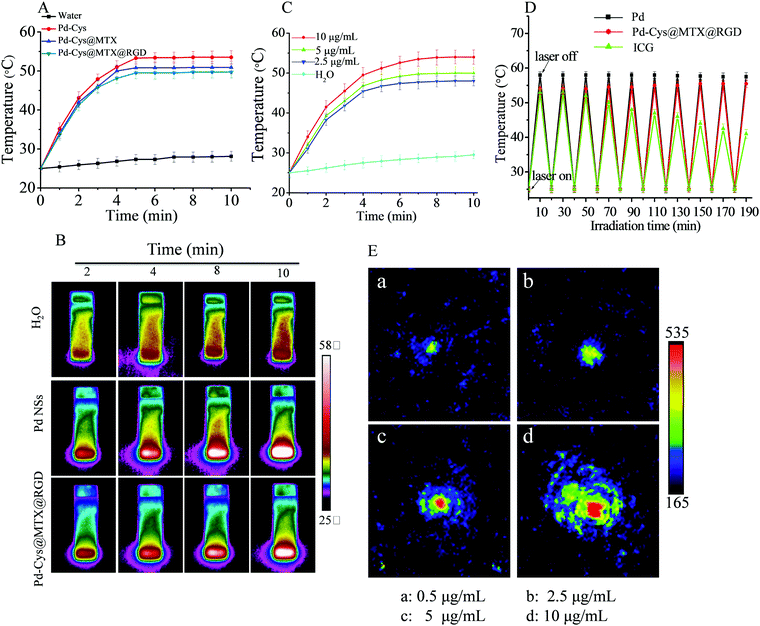 |
| Fig. 3 (A) Temperature profiles of the Pd and Pd-Cys@MTX@RGD nanosheets irradiated at 808 nm for 10 min (0.3 W cm−2). (B) The photothermal images of the Pd and Pd-Cys@MTX@RGD nanosheets irradiated at 808 nm (0.3 W cm−2). (C) Temperature profiles of different concentrations of Pd nanosheets irradiated at 808 nm (0.3 W cm−2) for 10 min. (D) The photothermal stability of the Pd and Pd-Cys@MTX@RGD nanosheets irradiated at 808 nm (1 W cm−2). (E) Photoacoustic signal images of Pd nanosheets excited at 808 nm. | |
3.4 The anti-inflammation properties of Pd-Cys@MTX@RGD in vitro
The vascular endothelial growth factor (VEGF) can promote the proliferation of HUVECs and angiogenesis, which can lead to the proliferation of joint synovial tissue and aggravation of arthritic progression.33 Therefore, inhibiting the proliferation of HUVECs induced by VEGF is an important step in inhibiting the inflammatory response. Firstly, we measured the proliferation of HUVECs with different treatments and the results showed that the proliferation rate of the HUVECs induced with VEGF was significantly increased to 150%. However, after treatment with 10 μg mL−1 MTX, the proliferation rate was reduced to 70%. On the other hand, after 808 nm laser irradiation the Pd-Cys nanosheets showed little inhibition to HUVECs, with a proliferation rate of 95%. Furthermore, the proliferation rate of 10 μg mL−1 Pd-Cys@MTX was 63%, 1.1 times that of MTX. We speculated that is due to the synergistic effect of MTX and PTT. However, it is interesting that the inhibition rate was very low for Pd-Cys@MTX without 808 nm laser irradiation, which may be due to a low quantity of MTX being released. Finally, after treatment with the Pd-Cys@MTX@RGD nanosheets, the proliferation rate of the HUVECs was only 45% under 808 nm irradiation, which was significantly higher than other experimental groups, thus demonstrating that the therapeutic effect of Pd-Cys@MTX@RGD nanosheets was effectively enhanced under the controlled release of MTX release and targeting of the RGD. Therefore, the Pd-Cys@MTX@RGD nanosheets have the potential to inhibit neovascularization and relieve synovitis in RA.
Furthermore, to investigate the anti-inflammatory properties of the Pd-Cys@MTX@RGD nanosheets, we investigated the expression of pro-inflammatory cytokines (TNF-α, COX-2) in RAW 264.7 cells after treatment with various therapies, and the results are shown in Fig. 4B. In the control groups, the levels of TNF-α and COX-2 were increased by nearly 50-fold compared with the normal levels after being induced with IL-1β. After the addition of MTX and nanotherapy, the expressions of TNF-α and COX-2 in inflammatory RAW 264.7 cells were inhibited with varying degrees. Among them, the expressions of TNF-α and COX-2 were significantly decreased after treatment with 10 μg mL−1 MTX. However, the Pd-Cys nanosheets showed a weak therapeutic effect after 808 nm laser irradiation indicating that photothermal therapy can slightly suppress the expression of TNF-α and COX-2, while by irradiating the Pd-Cys@MTX with an 808 nm laser the effect was significantly enhanced under MTX and photothermal therapies. However, without irradiation, Pd-Cys@MTX showed weak inhibition. Moreover, it was found that the Pd-Cys@MTX@RGD nanosheets showed the best therapeutic effect, where the expression of TNF-α and COX-2 were regulated to a nearly normal level. Therefore, in summary, the results show that Pd-Cys@MTX@RGD effectively inhibited the two characteristic inflammatory responses through the precise control of MTX release.
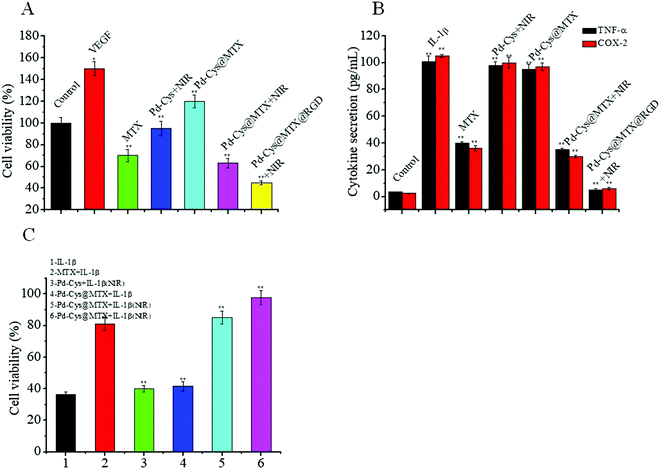 |
| Fig. 4 (A) HUVEC proliferation efficiency upon treatment with MTX, Pd-Cys, Pd-Cys@MTX and Pd-Cys@MTX@RGD with/without 808 nm (0.3 W cm−2) irradiation. (B) The effects of various treatments in inhibiting macrophage inflammatory cytokines (TNF-α and COX-2). (C) The RAW 264.7 cell viability of MTX, Pd-Cys, Pd-Cys@MTX and Pd-Cys@MTX@RGD with/without 808 nm (0.3 W cm−2) irradiation. (p* ≤ 0.1, p** ≤ 0.05). | |
3.5 Detection of photothermal and photoacoustic imaging in vivo
The photothermal and photoacoustic imaging properties of Pd nanosheets can not only provide a diagnostic platform for disease, but can also be used to observe the accumulation of a drug in a lesion. In order to investigate the imaging ability and accumulation of Pd-Cys@MTX@RGD nanosheets in RA, the RA mice were induced by bovine collagen II and randomly divided into three groups (5 mice per group). The groups were then injected with 100 μL of saline, Pd-Cys and Pd-Cys@MTX@RGD (5 mg kg−1), respectively. Post injection 6 h, all groups being irradiated with an 808 nm laser for 10 min, the results showed that the photothermal effect of the saline was the weakest because no photothermal agent was used (Fig. 5A). In the Pd-Cys group, the photothermal effect was significantly enhanced, with the temperature reaching 44.8 °C (Fig. 5B). Moreover, Pd-Cys@MTX@RGD showed a stronger photothermal effect, with the temperature reaching 50.2 °C due to more Pd-Cys@MTX@RGD nanosheets accumulating in the inflamed paws with high targeting capability. Next, we detected the photoacoustic imaging of the above groups. As shown in Fig. 5C, a weak photoacoustic signal was exhibited at the paws of the RA mice without treatment. As in the Pd-Cys and Pd-Cys@MTX@RGD groups, the photoacoustic signal was significantly increased because the Pd nanosheets are strong NIR absorbers. Moreover, the strongest signal was observed in the Pd-Cys@MTX@RGD group, which almost covered the entire inflamed area in the paw. This further demonstrates that the Pd-Cys nanosheets and Pd-Cys@MTX@RGD have photothermal and photoacoustic capabilities, and that compared to Pd-Cys, the targeting ability of the Pd-Cys@MTX@RGD nanosheets is stronger, and the imaging signal in the inflamed area was stronger.
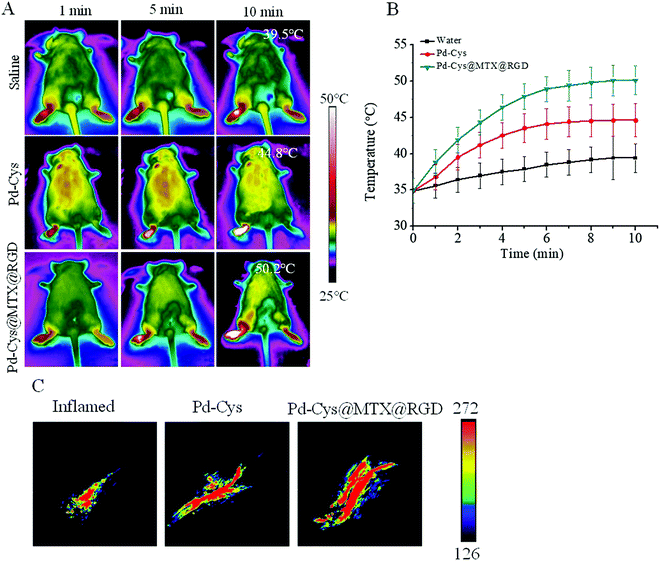 |
| Fig. 5 (A) Photothermal images of the Pd-Cys and Pd-Cys@MTX@RGD nanosheets in RA mice irradiated at 808 nm for 10 min (0.3 W cm−2). (B) Temperature profiles of the Pd-Cys and Pd-Cys@MTX@RGD nanosheets in RA mice irradiated at 808 nm for 10 min. (C) Photoacoustic signal images of the Pd-Cys and Pd-Cys@MTX@RGD nanosheets in RA mice excited with 808 nm irradiation. | |
3.6 The anti-inflammatory properties of Pd-Cys@MTX@RGD in vivo
In order to study the therapeutic effect of the Pd-Cys@MTX@RGD nanosheets in vivo, the RA mice were randomly divided into six groups (5 mice per group), and saline (G1), Pd-Cys + NIR (G2), Pd-Cys@MTX (G3), Pd-Cys@MTX + NIR (G4), Pd-Cys@MTX-RGD + NIR (G5) and MTX (G6) were injected into the RA mice at a dose of 100 μL (5 mg kg−1) every three days for two weeks. The inflammatory response in the paws was counted by measuring the clinical index, after 28 days. As shown in Fig. 6A, it can be observed that the clinical index continued to increase for the saline (G1) group and that the Pd-Cys + NIR (G2) combined with photothermal therapy treated mice showed a slight decrease. However, the mice treated with Pd-Cys@MTX showed an obvious difference with or without 808 nm irradiation. Under 808 nm irradiation, the clinical index of Pd-Cys@MTX + NIR (G4) decreased to 1.9, close to that of the therapeutic level of MTX (G6), while for the Pd-Cys@MTX (G3) without 808 nm irradiation mice, the clinical index was 2.9 due to the low release efficiency of MTX. Finally, the Pd-Cys@MTX-RGD (G5) almost suppressed the inflammatory response, with a clinical index of nearly 0. This is because in the presence of RGD targeting, MTX was precisely released into the inflamed region under NIR irradiation, by the synergistic effect of photothermal therapy.
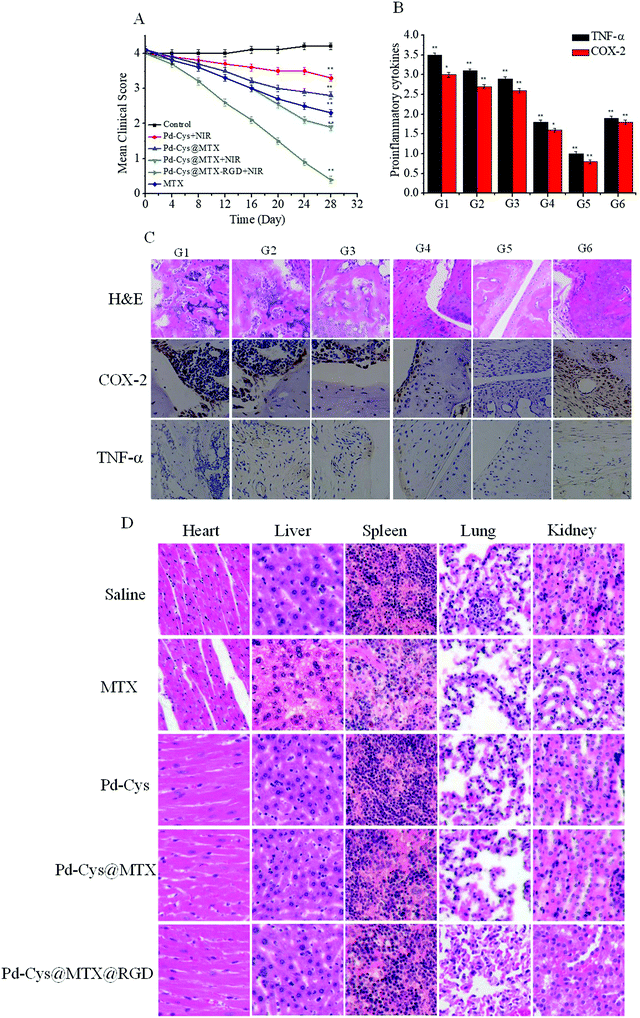 |
| Fig. 6 (A) Mean clinical score measurements of the various groups during treatment. (B) The joints were harvested and analyzed for pro-inflammatory cytokine (TNF-α and COX-2) levels. (C) Histology of the paws extracted from a normal mouse and RA mice after each treatment. H&E and immunohistochemical staining for TNF-α and COX-2 stained representative joint sections from the experiments. (D) Histological sections of major organs extracted 28 days after the various treatments. The images were acquired at 400× magnification. | |
To further investigate the therapeutic effect, we detected histological changes and pro-inflammatory cytokines (TNF-α, COX-2) in the paws in collagen-induced arthritis (CIA) mice using H&E staining and immunohistochemistry, and the results are shown in Fig. 6B. The joints of the paws treated with saline (G1) had severe articular cartilage erosion demonstrated by the fact that the intra-articular cartilage in the interface bone tissue appeared blurred in the image and there was a large amount of H&E staining into the inflamed tissue. And, from the immunohistochemistry imaging, high expression levels of COX-2 and TNF-α were observed for the saline group (G1). Compared with the saline group (G1), the cartilage erosion in the other groups (G2–G6) was inhibited to different extents. Among them, the therapeutic effect of Pd-Cys@MTX-RGD (G5) was the best, combining MTX with photothermal therapy. The H&E staining showed that the interface of the cartilage and bone tissue was clear and that COX-2 and TNF-α were completely inhibited after treatment with Pd-Cys@MTX-RGD. The results indicated that combination therapy with Pd-Cys@MTX-RGD effectively inhibited the inflammatory response and protected the articular cartilage from erosion. We then detected the toxicity to the major organs of each of the above treatments. The H&E stained sections of each major organ showed that the MTX caused liver and spleen toxicity (Fig. 6D). However, the Pd-Cys nanosheets showed good biocompatibility after loading with MTX, and Pd-Cys@MTX and Pd-Cys@MTX@RGD without NIR laser irradiation also showed low toxicity to the organs.
4. Conclusion
We designed and synthesized Pd-Cys@MTX@RGD nanosheets with photothermal effect. The Pd-Cys@MTX@RGD nanosheets effectively reduced the toxicity of MTX through controlled release and high targeting ability. Pd nanosheets are good NIR absorbers, and the Pd-Cys@MTX@RGD nanosheets showed a strong photoacoustic signal. Moreover, in anti-inflammation studies, the Pd-Cys@MTX@RGD nanosheets were found to significantly inhibit the proliferation of HUVECs induced by VEGF and the expression of pro-inflammatory cytokines in RAW 264.7 cells (TNF-α, COX-2) through the combination therapies of MTX and PTT. In vivo measurements showed that the Pd-Cys@MTX@RGD nanosheets accumulated in the paws of RA mice, and in further experiments were found to greatly reduce the inflammatory response, cartilage erosion and toxicity of MTX. Therefore, the nanosheets synthesized in this study were found to effectively reduce the toxicity of traditional drugs through a novel method, which offers valuable therapeutic advantages for the further development of PTT.
Conflicts of interest
The authors declare no competing financial interest.
Acknowledgements
This work was supported by the National Natural Science Foundation of China (21877051, 81803027 and 21371075), the Natural Science Foundation of Guangdong Province (2018A030310628) and the Planned Item of Science and Technology of Guangdong Province (2016A020217011).
References
- G. S. Firestein, Nature, 2003, 423, 356 CrossRef CAS PubMed.
- I. B. McInnes and G. Schett, Nat. Rev. Immunol., 2007, 7, 429 CrossRef CAS.
- Y. Liu, L. Ma, H. Zhou, X. Zhu, Q. Yu, X. Chen, Y. Zhao and J. Liu, J. Mater. Chem. B, 2018, 6, 3497 RSC.
- K. G. Saag, G. G. Teng, N. M. Patkar, J. Anuntiyo, C. Finney, J. R. Curtis, H. E. Paulus, A. Mudano, M. Pisu, M. Elkins-Melton, R. Outman, J. J. Allison, M. S. Almazor, S. L. Bridges, W. W. Chatham, M. Hochberg, C. Maclean, T. Mikuls, L. W. Moreland, J. O’Dell, A. M. Turkiewicz and D. E. Furst, Arthritis & Rheumatism-Arthritis Care & Research, 2008, 59, 762 Search PubMed.
- J. S. Smolen, R. Landewe, J. Bijlsma, T. Stamm, T. Takeuchi, R. Westhovens, M. de Wit and D. van der Heijde, Ann. Rheum. Dis., 2017, 76, 960 CrossRef PubMed.
- J. Swierkot and J. Szechinski, Advances in Clinical and Experimental Medicine, 2008, 17, 387 Search PubMed.
- K. Visser, W. Katchamart, E. Loza, J. Zochling and M. Dougados, Ann. Rheum. Dis., 2009, 68, 1086 CrossRef CAS.
- M. F. Bakker, J. W. G. Jacobs, P. M. J. Welsing, S. M. M. Verstappen, J. Tekstra, E. Ton, M. A. W. Geurts, J. H. van der Werf, G. A. van Albada-Kuipers, Z. Veen, M. J. van der Veen, C. M. Verhoef, F. Lafeber, J. W. J. Bijlsma and C. Utrecht Rheumatoid Arthrit, Ann. Intern. Med., 2012, 156, 329 CrossRef.
- J. H. Sun, W. Zhang, D. Y. Zhang, J. L. Shen, C. P. Tan, L. N. Ji and Z. W. Mao, J. Biomater. Appl., 2018, 32, 1253 CrossRef CAS PubMed.
- D. Y. Zhang, Y. Zheng, H. Zhang, L. He, C. P. Tan, J. H. Sun, W. Zhang, X. Y. Peng, Q. Q. Zhan, L. N. Ji and Z. W. Mao, Nanoscale, 2017, 9, 18966 RSC.
- J. L. Shen, H. R. Liu, C. F. Mu, J. Wolfram, W. Zhang, H. C. Kim, G. X. Zhu, Z. B. Hu, L. N. Ji, X. W. Liu, M. Ferrari, Z. W. Mao and H. F. Shen, Nanoscale, 2017, 9, 5329 RSC.
- X. Z. Ai, L. N. Lyu, Y. Zhang, Y. X. Tang, J. Mu, F. Liu, Y. X. Zhou, Z. H. Zuo, G. Liu and B. G. Xing, Angew. Chem., Int. Ed., 2017, 56, 3031 CrossRef CAS.
- H. Wang, P. H. Li, D. Q. Yu, Y. Zhang, Z. Z. Wang, C. Q. Liu, H. Qiu, Z. Liu, J. S. Ren and X. G. Qu, Nano Lett., 2018, 18, 3344 CrossRef CAS PubMed.
- H. W. Ji, L. Wu, F. Pu, J. S. Ren and X. G. Qu, Adv. Healthcare Mater., 2018, 7 Search PubMed.
- N. Huang, X. Chen, X. Zhu, M. Xu and J. Liu, Biomaterials, 2017, 141, 296 CrossRef CAS.
- Y. Zhou, H. J. Sun, F. M. Wang, J. S. Ren and X. G. Qu, Chem. Commun., 2017, 53, 10588 RSC.
- G. Burmester, A. J. Kivitz, H. Kupper, U. Arulmani, S. Florentinus, S. L. Goss, S. S. Rathmann and R. M. Fleischmann, Ann. Rheum. Dis., 2015, 74, 1037 CrossRef CAS PubMed.
- S. A. Bergstra, C. F. Allaart, T. Stijnen and R. Landewe, Arthritis Care Res., 2017, 19, 258 CrossRef PubMed.
- G. Chen, M. Xu, S. Zhao, J. Sun, Q. Yu and J. Liu, ACS Appl. Mater. Interfaces, 2017, 9, 33645 CrossRef CAS.
- T. S. Hauck, T. L. Jennings, T. Yatsenko, J. C. Kumaradas and W. C. W. Chan, Adv. Mater., 2008, 20, 3832 CrossRef CAS.
- X. Huang, I. H. El-Sayed, W. Qian and M. A. El-Sayed, J. Am. Chem. Soc., 2006, 128, 2115 CrossRef CAS.
- X. Wu, T. Ming, X. Wang, P. N. Wang, J. F. Wang and J. Y. Chen, ACS Nano, 2010, 4, 113 CrossRef CAS.
- J. Y. Chen, D. L. Wang, J. F. Xi, L. Au, A. Siekkinen, A. Warsen, Z. Y. Li, H. Zhang, Y. N. Xia and X. D. Li, Nano Lett., 2007, 7, 1318 CrossRef CAS.
- N. W. S. Kam, M. O’Connell, J. A. Wisdom and H. Dai, Proc. Natl. Acad. Sci. U. S. A., 2005, 102, 11600 CrossRef CAS.
- H. K. Moon, S. H. Lee and H. C. Choi, ACS Nano, 2009, 3, 3707 CrossRef CAS.
- M. Q. Chu, J. L. Peng, J. J. Zhao, S. L. Liang, Y. X. Shao and Q. Wu, Biomaterials, 2013, 34, 1820 CrossRef CAS.
- W. Fang, S. Tang, P. Liu, X. Fang, J. Gong and N. Zheng, Small, 2012, 8, 3816 CrossRef CAS.
- S. Shi, X. Chen, J. Wei, Y. Huang, J. Weng and N. Zheng, Nanoscale, 2016, 8, 5706 RSC.
- Z. X. Zhao, S. G. Shi, Y. Z. Huang, S. H. Tang and X. L. Chen, ACS Appl. Mater. Interfaces, 2014, 6, 8878 CrossRef CAS.
- R. O. Hynes, Nat. Med., 2002, 8, 918 CrossRef CAS.
- M. A. Dechantsreiter, E. Planker, B. Mathä, E. Lohof, G. Hölzemann, A. Jonczyk, S. L. Goodman and H. Kessler, J. Med. Chem., 1999, 42, 3033 CrossRef CAS.
- X. Huang, S. Tang, B. Liu, B. Ren and N. Zheng, Adv. Mater., 2011, 23, 3420 CrossRef CAS.
- H.-N. Woo, J.-S. Park, A. R. Gwon, T. V. Arumugam and D.-G. Jo, Biochem. Biophys. Res. Commun., 2009, 390, 1093 CrossRef CAS.
Footnote |
† Electronic supplementary information (ESI) available. See DOI: 10.1039/c8tb02302f |
|
This journal is © The Royal Society of Chemistry 2019 |