PAF-1@cellulose nanofibril composite aerogel for highly-efficient removal of bisphenol A†
Received
6th October 2018
, Accepted 21st November 2018
First published on 21st November 2018
Abstract
Bisphenol A (BPA), an endocrine disrupting compound (EDC) that affects the human nervous system, is used worldwide and is difficult to remove from water. PAF-1@cellulose nanofibril (CNF) composite aerogels are prepared through a double-cross-linking method and applied as adsorbents to remove BPA from water. With the successful combination of the high surface area of PAF-1 and the bulk skeleton of CNFs, as well as strong interactions between BPA molecules and the networks, the resulting composite aerogels can remove 77.7% of BPA, dissolved in water, within 10 seconds at an adsorption rate of 532.2 mg g−1 min−1, and their removal efficiency reaches 97.0% after 1 hour of adsorption. The adsorption capacity of PAF-1@CNF composite aerogels towards BPA is estimated to be as high as 1000 mg g−1 according to the Langmuir adsorption model.
Introduction
Bisphenol A (BPA) is a chemical compound primarily used to produce polycarbonate plastics and epoxy resins since 1960s.1 About 2.7 million tons of BPA-based polymers are manufactured every year and widely utilized in food containers and packaging materials.2 Although the U.S. Food and Drug Administration (FDA) stated the safety of BPA, detectable levels of BPA were found in human urine, plasma, placenta and even in the blood of newborns.3 More and more scientific studies proved that BPA can obstruct the human nervous system and endocrine system via imitating and disturbing the biological activities of estrogen hormones.4 Owing to the widespread usage of BPA products in drinking water and beverage bottles but difficult degradation in water,5 the removal of BPA from aquatic environments is of great concern to the public and regulatory agencies.6 Nowadays, physical adsorption is the most common method to remove BPA from water,7,8 because of its simple and low energy-consuming operations.9 Various solid adsorbents, such as activated carbon,10,11 carbon nanotubes,12 graphene oxides,13 zeolites,14 bentonites,15 chitosan,16 graphene,17 and metal–organic frameworks (MOFs),18 were fabricated and used to separate BPA from aqueous solutions. However, the limited adsorption capacities and heavy metal ions contained in MOFs inspired researchers to investigate alternative metal-free materials with high surface areas and stabilities.
Porous aromatic frameworks (PAFs)19 wholly constructed from light elements with high specific surface areas and high chemical and thermal stabilities are good candidates and can be particularly useful for the removal of organic compounds in water,20 since their constituent benzene rings in the frameworks would exert strong π–π interactions with the organic compounds.21 PAFs such as PAF-1,22 PAF-5,23 and PAF-45 (ref. 24) are expected to offer high adsorption capacities and, at the same time, a large resistance against the harsh conditions in practice. In particular, PAF-1, which has a specific surface area up to 5600 m2 g−1 (BET model), is an ideal material for BPA adsorption. However, on the other hand, the powder form of PAFs limits their applicability and recycling possibility in the purification of water from organic contaminants, which is also a common challenge must be addressed for other solid adsorbents.
Cellulose nanofibrils (CNFs), the extracts of plant cell walls,25 offer a great possibility to fabricate micro- and nano-structured aerogels,26 in which CNFs self-assemble and form the bulk skeleton via hydrogen bonds of their hydroxyl groups.27 As a class of bio-friendly bulk nanomaterials of low cost, light weight, large aspect ratio, non-toxicity, and flexibility,28 CNF aerogels are ideal substrates to host porous adsorbents to fabricate composite aerogels. MOFs, such as ZIF-8, UiO-66, and MIL-100, were incorporated into CNF aerogels to adsorb Cr(VI) ions from water. And the adsorption capacity of the UiO-66 aerogel is about 18 mg g−1.29 Herein, by successfully combining the ultra-high surface area of PAF-1 and the bulk skeleton of CNFs, we fabricated PAF-1@CNF composite aerogel monoliths through a double-cross-linked (physical and chemical cross-linked) method.30 The resulting composite aerogels show both a high adsorption capacity and a fast adsorption rate for the removal of BPA from water.
Experimental
Chemicals and materials
All the chemicals were purchased from commercial producers and used as received without further purification. Epichlorohydrin (ECH, ≥99.5%, GC), chromium(III) nitrate nonahydrate (99%), 1,4-benzene dicarboxylic acid (H2BDC, 99%), and bis(1,5-cyclooctadiene) nickel(0) ([Ni(cod)2], 96%) were purchased from Aladdin. 1,5-Cyclooctadiene (cod, 99+%) and 2,2′-bipyridyl (99+%) were purchased from Acros. Biphenyl (99%) was purchased from 3A Chemical. Aluminum nitrate nonahydrate (98%), aluminum chloride, and tetrafluoroterephthalonitrile (TFTPN, 98%) were purchased from Alfa Aesar. 5,5′,66′-tetrahydroxy-3,3,3′,3′-tetramethyl-1,1′-spirobisindane (TTSBI, 97%), N,N-dimethylformamide (DMF, 99.8%, extra dry, with molecular sieves), and dichloromethane (99.9%, extra dry, with molecular sieves) were purchased from Energy Chemical. Potassium carbonate (≥99%) was purchased from Sigma-Aldrich. 2,2-Di-(4-hydroxyphenyl) propane (BPA, CP) and tert-butanol (CP) were purchased from Sinoparm Chemical Reagent Co. Ltd. Brilliant blue G (BB-G) and acid red 94 (AR-94) were purchased from TCI Co. Ltd. Methylene blue (MB) and rhodamine B (R-B) were purchased from Tianjin Guangfu Chemical Co. Ltd. Dialysis tubing (molecular weight cutoff: 8000–14
000) was purchased from Solarbio. Ethyl alcohol, methyl alcohol, acetone, tetrahydrofuran, trichloromethane, DMF (A.R.), hydrochloric acid (HCl), and hydrofluoric acid (HF) were purchased from Beijing Chemical Works. Zeolite-X was purchased from Luoyang Jianlong Chemical Co. Ltd. Activated carbon was purchased from the Gongyi Beishankou Hongchang water purification material factory.
Preparation of cellulose nanofibrils (CNFs) and porous materials
Cellulose nanofibrils were prepared by chemical pretreatment and high-intensity ultrasonication following the protocol reported in the literature.31 Tetrakis(4-bromophenyl) methane, PAF-1,22 and other porous materials, including PAF-45,24 MIL-101 (Cr),32 MIL-53 (Al),33 and PIM-1
34 were synthesized according to the protocols reported in the literature.
Synthesis of CNF composite aerogels loaded with porous materials
Taking, for example, the PAF-1@CNF composite aerogel, 0.05 g of PAF-1 were dispersed in 4 mL of DMF under stirring at room temperature. 0.05 g of CNFs were dispersed in 50 mL of distilled water, which was facilitated by high-intensity ultrasound by means of a common ultrasonic generator. The CNF aqueous dispersion was added into the PAF-1 DMF dispersion under stirring within 20 min, followed by the addition of 0.5 mL of ECH. The resulting mixtures were further stirred for 24 h to form a homogeneous suspension, which, subsequently, was subjected to solvent exchange with t-BuOH via dialysis. The dispersion of the PAF-1/CNF mixtures with a cellulose faction of 0.5 vol% in t-BuOH was poured into a mold, followed by being frozen by liquid nitrogen and dried for 12 h at 16 Pa by using a freeze-dryer to produce PAF-1@CNF composite aerogels with open porous structures. A similar protocol was applied to produce CNF composite aerogels loaded with other porous materials.
Characterization
Fourier transform infrared (FTIR) spectra were recorded on a Nicolet Impact 410 Fourier transform infrared spectrometer. Thermogravimetric analysis (TGA) data were obtained on a Mettler Toledo thermal analyzer at a heating rate of 10 °C min−1 under an air atmosphere. Powder X-ray diffraction (PXRD) data were obtained on a Riguku D/MAX2550 diffractometer by using CuKα radiation at 40 kV, 200 mA. Scanning electron microscopy (SEM) images were recorded by means of a Hitachi SU8010. N2 adsorption–desorption isotherms were conducted on a Quantachrome Autosorb iQ SN analyzer at 77 K. UV-vis spectroscopy was measured on a Cary 60 Varian and UV-vis spectrometer in the wavelength range of 200–800 nm.
Adsorption kinetics
The kinetic study of the batch adsorption of adsorbents, including the as-prepared pristine and composite CNF aerogels as well as porous material powders, was carried out to assess their adsorption performance towards BPA in water. 1 mg of the aerogels was placed into a 20 mL glass vial at ambient temperature, in which there was 5 mL of BPA aqueous solution (0.1 mmol L−1) and a magnetic stirring bar. All the adsorption experiments were carried out at the same stirring speed. A series of aliquots of the aerogel/BPA mixture dispersions were taken out by means of a syringe at different points of adsorption time and filtered rapidly through 0.22 μm inorganic membrane filters. The remaining BPA concentration of each sample was determined with the aid of UV-vis absorption spectroscopy, in which the intensity of the maximum absorption peak at 276 nm was used for a quantitative assessment.
The removal efficiency of BPA (in %) by adsorbents was calculated by the equation:
where
C0 (mmol L
−1) and
Ct (mmol L
−1) are the initial and remaining concentration of BPA in water, respectively, after adsorption for a certain time.
The amount of BPA, which was adsorbed into adsorbents, was calculated by the equation:
where
qt (mg g
−1) is the amount of BPA adsorbed by 1 g of the adsorbents after adsorption time
t (min),
C0 (mmol L
−1) and
Ct (mmol L
−1) are the initial and remaining concentration of BPA in water, respectively,
Mw (g mol
−1) is the molar mass of the BPA, V (mL) is the volume of the BPA solution and
m (g) is the adsorbent mass.
The adsorption rate of adsorbents was approximately described by Ho and McKay's pseudo-second-order adsorption model,35 with the equation below:
where
qt and
qe are the adsorption amount of BPA (mg g
−1) after the adsorption was carried out for a given time
t (min) and reached the equilibrium state, respectively, and
kobs is the second-order rate constant (g mg
−1 min
−1).
Adsorption isotherm
1 mg of the adsorbents was placed into a 20 mL glass vial, followed by the addition of 5 mL of the aqueous solution of BPA with different initial concentrations. After stirring for 48 h, the dispersion was filtered through a 0.22 μm inorganic membrane filter and the filtered solution was assessed by means of UV-vis absorption spectroscopy. The Langmuir adsorption model36 was applied to fit the adsorption isotherm by the following equation:
where qe (mg g−1) is the equilibrium adsorption amount of BPA, qmax,e (mg g−1) is the maximum adsorption capacity, c (mmol L−1) is the remaining BPA concentration after the adsorption equilibrium was reached, and K (mol−1) is the adsorption equilibrium constant.
Reuse of PAF-1@CNF composite aerogels
10 mg of the PAF-1@CNF composite aerogels were placed into a 50 mL glass vial, in which there were 20 mL of BPA aqueous solution (0.1 mmol L−1) and a magnetic stirring bar at ambient temperature. The mixture was stirred for 2 h, and then filtered through a Whatman filter paper. The residual BPA concentration in the filtrate was determined by means of UV-vis absorption spectroscopy. The PAF-1@CNF composite aerogels were regenerated by washing with 10 mL of methanol for 1 h, followed by filtration and freeze-drying. The adsorption/desorption cycle was performed twelve times to study the reusability of the PAF-1@CNF composite aerogels.
Dye adsorption
1 mg of adsorbent was placed into a 20 mL glass vial, followed by the addition of 5 mL of an aqueous solution of the dyes (0.1 mmol L−1). After stirring for 2 h at room temperature, the dispersion was filtered through a 0.22 μm inorganic membrane filter and the filtered solution was collected. The remaining dye concentration of each sample was determined by UV-vis absorption spectroscopy, in which the intensity of the maximum absorption peak at 664 nm (MB), 550 nm (R-B), 582 nm (BB-G) and 549 nm (AR-94) was used for the quantitative assessment. The dye removal efficiency (%) was calculated using the equation:
where C0 (mmol L−1) and Ct (mmol L−1) are the initial and remaining concentrations of dye in water, respectively, after adsorption for a certain time.
Results and discussion
Fig. 1 demonstrates the typical process to produce PAF-1@CNF composite aerogels. The suspension of PAF-1 in DMF and CNFs in distilled water was mixed into a homogeneous suspension to have the PAF-1 particles fixed on the CNF skeleton, which is driven by van der Waals interactions, while CNFs connected with each other through hydrogen bonds. Subsequently, epichlorohydrin (ECH) was added into PAF-1/CNFs mixture to create covalent cross-linkages between the CNFs and then form a stable PAF-1@CNF composite gel. Afterwards, the solvent mixture soaked within the resulting PAF-1@CNF gels was replaced by t-BuOH, and a freeze-drying method was employed to produce the composite aerogels. By adjusting the weight ratio of PAF-1 to CNFs, PAF-1@CNF aerogels loaded with PAF-1 contents of 20 wt%, 33.3 wt%, and 50 wt% were fabricated. The structural features of the resulting PAF-1@CNF composite aerogels were characterized by means of scanning electron microscopy (SEM), thermogravimetric analysis (TGA), and N2 sorption isotherms. As shown in the SEM images (Fig. S1†), PAF-1 particles are located uniformly in the interspace of the CNF aerogel. When the loading amount reaches 50 wt%, CNFs are completely covered by PAF-1. Powder loadings higher than 50 wt% were not considered because of the lower stability of the obtained aerogels. In the TGA curves (Fig. S2a†), for instance, with a PAF-1 loading of 50 wt%, mass losses at temperatures over 220 °C (48.1%) and 410 °C (47.9%) were observed, which are attributed to CNF decomposition and PAF-1 framework collapse, respectively. N2 adsorption–desorption isotherms (Fig. S2b†) indicate rising sorption amounts in PAF-1@CNF composite aerogels with an increase of the PAF-1 loadings. The composite aerogel with 50 wt% PAF-1 presents the highest BET surface area of 2203 m2 g−1 and a relatively low density of 0.015 g cm−3. The powder X-ray diffraction (PXRD) technique and Fourier transform infrared (FTIR) spectroscopy were used to assess the chemical nature of the PAF-1@CNF composite aerogel with a 33.3% PAF-1 content. PXRD patterns (Fig. S2c†) reveal that the crystallinity of the CNFs is retained in the resulting composite aerogel. In FTIR spectra (Fig. S2d†), the primary vibration peaks of the benzene constituents of PAF-1 at 3033 cm−1, 1489 cm−1, are 809 cm−1 are present in the spectrum of the composite aerogel, suggesting that the PAF-1 particles are successfully deposited in CNF networks. In comparison with physically cross-linked CNF aerogels, the characteristic bands of the double-cross-linked PAF-1@CNF composite aerogel are sharper, indicating the formation of covalent bonding in the networks.37 The stretching band of the hydroxyl moieties of CNF aerogel networks blue-shifted from 3430 cm−1 in the pristine physically cross-linked CNF aerogel to 3350 cm−1 in the PAF-1@CNF composite aerogel, which manifests, after the PAF-1@CNF composite aerogel is covalently cross-linked, as strong hydrogen bonds between the hydroxyl moieties that are left generated within the CNF aerogel networks.31 SEM images of networks in different stages clearly show that the addition of ECH induces chemical cross-linking and causes a noticeable transformation of reasonably aligned nanofibrillar cellulose structures (Fig. 2a) to randomly porous structures in the pristine double-cross-linked CNF aerogel (Fig. 2b). Fig. 2c indicates that the uniform PAF-1 particles coat onto the cross-linked CNFs networks. All the results above suggest a successful fabrication of a PAF-1@CNF composite aerogel with both physical and chemical cross-linking.
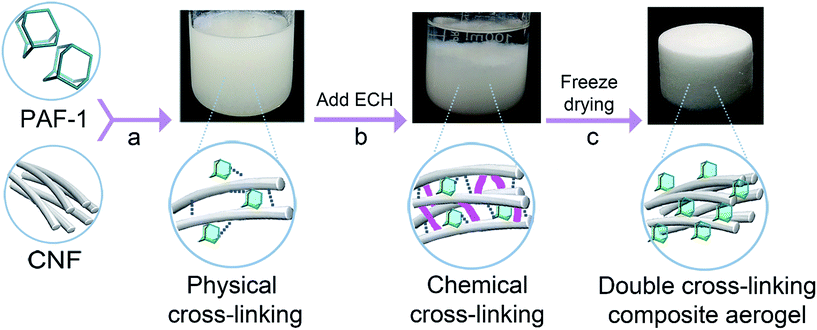 |
| Fig. 1 Schematic illustration of the preparation pathway of the PAF-1@CNF composite aerogel. (a) PAF-1 and CNFs are mixed in solvent to form a physically cross-linked hydrogel. (b) The addition of ECH to create a chemical cross-linkage within the hydrogel networks. (c) The PAF-1@CNF composite hydrogel is transformed to the composite aerogel by freeze-drying. | |
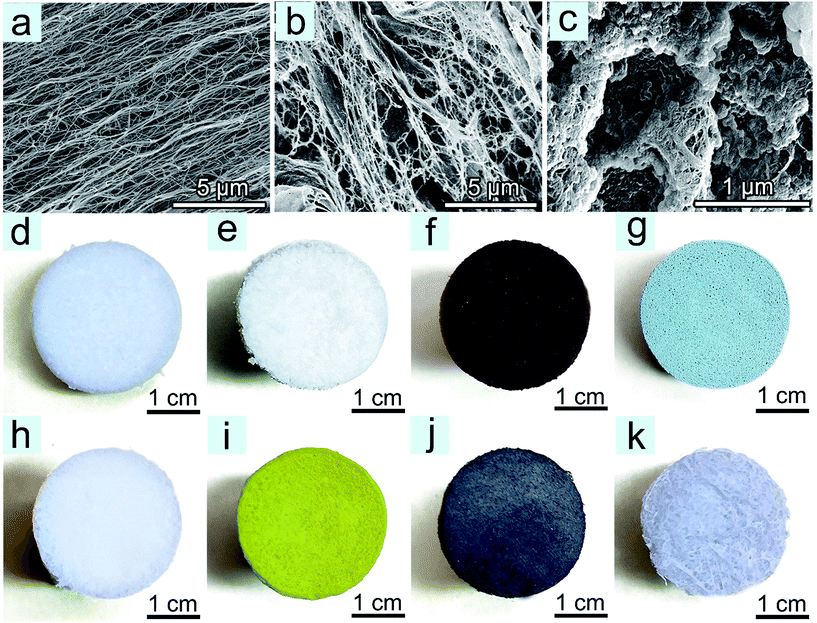 |
| Fig. 2 SEM images of (a) a pristine physically cross-linked CNF aerogel, (b) a double-cross-linked CNF aerogel, and (c) a PAF-1@CNF composite aerogel. Optical photographs of (d) a pristine double-cross-linked CNF aerogel and composite aerogels of (e) PAF-1@CNF, (f) PAF-45@CNF, (g) MIL-101@CNF, (h) MIL-53@CNF, (i) PIM-1@CNF, (j) activated carbon@CNF, and (k) zeolite-X @CNF. | |
The PAF-1 offers a great number of benzene rings and the CNF aerogel bears hydroxyl groups left over after ECH-induced covalent cross-linking, and at the same time the BPA molecule bears two benzene rings and two phenolic groups. Therefore, BPA can interact with benzene moieties of PAF-1 via π–π interactions and the hydroxyl groups of the CNF components via hydrogen bonding in PAF-1@CNF composite aerogels. To evaluate the BPA removal abilities of PAF-1@CNF composite aerogels, CNF-based aerogels with other typical porous materials, such as PAF-45, MIL-101, MIL-53, PIM-1, activated carbon, and zeolite-X, were also produced following the preparation process described above for comparison. The colors of the as-prepared composite aerogels are dependent on the nature of the porous materials trapped inside (Fig. 2d–k). For example, the pristine double-cross-linked CNF aerogel is slightly blueish while the PAF-1@CNF composite aerogel is fairly white and the PAF-45@CNF one is dark brown. SEM images of composite aerogels coated with other porous materials are shown in Fig. S3a–f.†
The porous materials in composite aerogels are all incorporated with a ratio of 50 wt%. According to N2 sorption isotherms (Fig. 3a and b), their BET surface areas were calculated and are listed in Table S1.† It can be clearly found that the BET surface area is greatly reduced when the powder material is produced into an aerogel, which is common in the post-modification process. BPA adsorption experiments of the corresponding porous materials in their powder form were tested as a control factor.
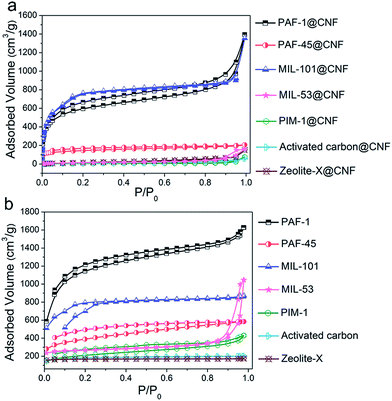 |
| Fig. 3 (a) N2 adsorption isotherm of the as-prepared CNFs-based composite aerogels loaded with PAF-1, PAF-45, MIL-101, MIL-53, PIM-1, activated carbon, and zeolite-X. (b) N2 adsorption isotherm of powders of PAF-1, PAF-45, MIL-101, MIL-53, PIM-1, activated carbon, and zeolite-X. | |
The adsorption isotherms of BPA by composite aerogels and powders were determined by equilibrium adsorption experiments. The equilibrium uptakes on the basis of the equilibrium BPA concentration left over after 48 hours of adsorption were calculated according to the Langmuir adsorption model. From Fig. S4 and Table S1,† we can obviously see that the maximum sorption capacities on the aerogels were all lower than that on their corresponding powders, which results from the reduction of BET surface areas of the powdery porous materials. In addition, there is no doubt that the adsorption capacity of the PAF-1@CNF composite aerogel is the highest of all the tested materials, except for powder PAF-1. And the maximum adsorption capacity of the PAF-1@CNF composite aerogels at equilibrium (qmax,e) is about 1000 mg g−1 (Fig. 4a and b), which, to our best knowledge, is also the highest value reported so far among all the powder adsorbents (Fig. 5).38 According to a previous report, the concentration of BPA in industrial waste water produced by a paper production facility is 72 μg L−1.39 As the maximum adsorption capacity of the PAF-1@CNF composite aerogel is 1000 mg g−1, only 0.072 mg sample are needed to process 1 L of such waste water. In comparison with PAF-1@CNF, 2.66 mg and 10.43 mg of adsorbents are required for commercial chitosan and zeolite, respectively, which is about 37 times more. The above data suggest that the PAF-1@CNF composite aerogel can be used as a potential adsorbent for practical waste water treatment. Additionally, the removal efficiencies and adsorption kinetics were executed by the incubation of 1 mg of composite aerogels or powders in 5 mL of an aqueous solution of BPA at a concentration of 0.1 mmol L−1. The BPA removal efficiencies (Fig. 6a and b) of PAF-1 and the PAF-1@CNF aerogel reached 89.2% and 77.7% within 10 seconds, respectively, and then up to 97.0% in 1 hour. It is worth noting that, although the BET surface area decreased nearly 50% after PAF-1 transformed into the PAF-1@NFC aerogel, the removal efficiency of PAF-1@CNF aerogel is similar to that of PAF-1 which is probably owing to the enrichment of benzene rings in the materials thus providing stronger π–π interactions in the PAF-1@CNF aerogel. Based on this principle, the PAF-45 aerogel also presents a relatively high removal efficiency of 75%, indicating PAF materials are good candidates in BPA removal from water. It is notable that MIL-53 has a high BPA uptake of 93.7% in 1 hour, but the removal efficiency of its bulky aerogel dramatically dropped to 12.4%. Attributed to its flexible framework, pore blocking occurred in the CNF chemically cross-linking procedure. On the contrary, other CNF-based composite aerogels showed much lower BPA removal efficiencies and so could hardly be employed in BPA removal. Furthermore, by using Ho and McKay's pseudo-second-order adsorption model, the apparent pseudo-second-order rate constant (kobs) of adsorption in the PAF-1@CNF composite aerogel towards BPA was fitted and calculated as 0.282 mg g−1 min−1 (Fig. S5 and S6, Table S1†). Recently, W. R. Dichtel and co-workers reported the fabrication of a porous β-cyclodextrin polymer with an ultrahigh adsorption efficiency of about 95% of its equilibrium uptake in 10 seconds; however, because of its limited surface area (263 m2 g−1), its adsorption capacity only reached 88 mg g−1.40 In our work, BPA adsorption benefited from the high surface area of the PAF-1@CNF composite aerogel.
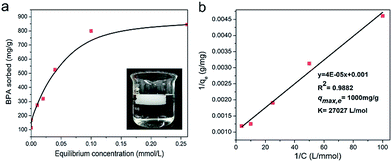 |
| Fig. 4 (a) Plot of the adsorption equilibrium uptake of PAF-1@CNF composite aerogels versus the initial concentration of BPA in water. (b) Adsorption isotherm of PAF-1@CNF composite aerogels towards BPA in water, fitted according to a Langmuir model. | |
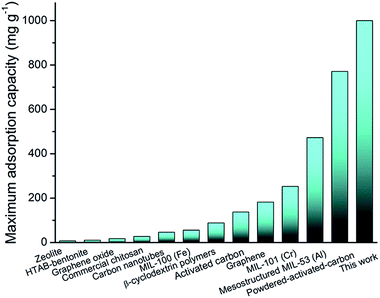 |
| Fig. 5 Histogram of the BPA maximum adsorption capacity (mg g−1) of zeolite,14 bentonite,15 graphene oxide,13 commercial chitosan,16 carbon nanotubes,12 MIL-100 (Fe), MIL-101 (Cr),15 activated carbon,10 graphene,17 β-cyclodextrin polymers,40 mesostructured MIL-53 (Al),33 powdered-activated-carbon,11 and the PAF-1@CNF aerogel. | |
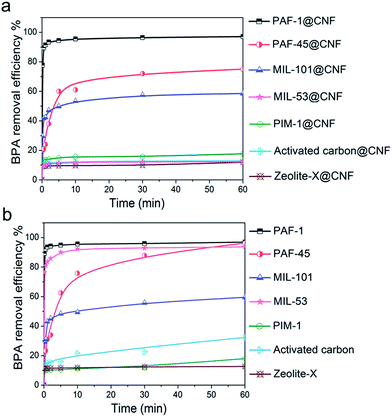 |
| Fig. 6 (a) BPA removal efficiency of the as-prepared CNF-based composite aerogels loaded with PAF-1, PAF-45, MIL-101, MIL-53, PIM-1, activated carbon, and zeolite-X. (b) BPA removal efficiency of powders of PAF-1, PAF-45, MIL-101, MIL-53, PIM-1, activated carbon, and zeolite-X. 1 mg of the composite aerogels or powder in 5 mL of aqueous solution of BPA with a concentration of 0.1 mmol L−1. | |
The recyclability of adsorbents is also crucial for a cost-effective practical usage. Therefore, the release of BPA molecules out of the PAF-1@CNF composite aerogels was conducted by washing with methanol. After performing 12 adsorption/desorption cycles, the removal efficiency of PAF-1@CNF composite aerogels remained as high as 95.7% towards BPA, indicative of an excellent reusability (Fig. 7a). SEM images confirmed that PAF-1 particles are stably attached to the CNF aerogel networks after twelve cycles (Fig. 7b).
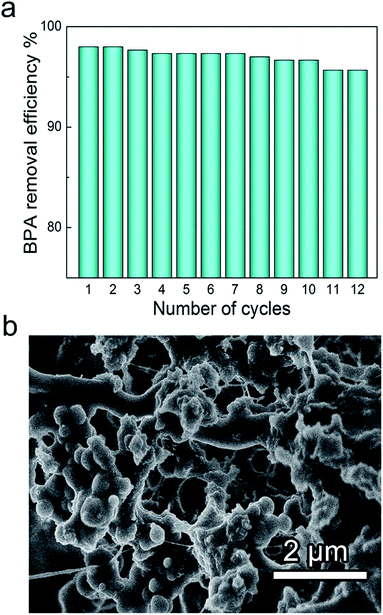 |
| Fig. 7 (a) Histogram of the BPA removal efficiency of PAF-1@CNF composite aerogels after 12 adsorption/desorption cycles. The removal efficiency for each cycle is the average of three experimental values. (b) SEM image of PAF-1@CNF composite aerogels obtained after 12 adsorption/desorption cycles. | |
To better describe the molecule sorption behavior in the synthesized PAF-1@CNF composite aerogel, four dye molecules with different size/shape/charge were selected (Table S2†) and dye adsorption experiments were carried out.41 As shown in Fig. S7a,† the neat CNF aerogel adsorbed few dye molecules because of its low surface area. The adsorption of BB-G in CNF can be attributed to the surface adsorption through hydrogen bonds as a large amount of hydroxyl groups are exposed on the CNF surface. Aside from the surface adsorption effect of CNF (Fig. S7b†), R-B molecules (dye-3) with a similar size to the micropore size of PAF-1 were adsorbed nearly 100%. Dye molecules larger than the micropore size (AR-94 and BB-G) were adsorbed in the mesopores in the composite aerogel. BB-G molecules were adsorbed more than AR-94 because of its flexible structure which allows part of BB-G molecules to diffuse inside the micropores of the monolith. The adsorption amount of MB molecules is the least as the linear chain structure of MB allows the easy dye release in the solution. The results above suggest a size and shape dependent adsorption in PAF-1@CNF composite aerogel.
Conclusions
In this work, PAF-1@CNF composite aerogels were prepared with the maximum PAF-1 loading up to 50 wt%. PAF-1 particles were incorporated uniformly in the aerogel network. The resulting PAF-1@CNF composite aerogels show BET surface areas as high as 2203 m2 g−1 and a relatively low density of 0.015 g cm−3. Due to the π–π interaction associated with the benzene rings of PAF-1 components and hydrogen bonding associated with the hydroxyl groups of the CNF components, the PAF-1@CNF composite aerogel with a 50 wt% PAF-1 loading showed an excellent adsorption performance towards BPA in aqueous solution, and can remove 77.7% of the BPA molecules initially present in water within 10 seconds at an adsorption rate of 532.2 mg g−1 min−1, and its removal efficiency reached 97.0% after 1 hour of adsorption with a maximum adsorption capacity of 1000 mg g−1, which is the highest value reported thus far. According to the dye adsorption experiments, size and shape dependent molecule adsorption are suggested in PAF-1@CNF composite aerogels. Besides, the remarkable reusability of PAF-1@CNF composite aerogels should provide a novel type of super-adsorbent for practical water treatment.
Conflicts of interest
There are no conflicts to declare.
Acknowledgements
This work was supported by National Natural Science Foundation of China (21503038, 21531003, 91622106 and 31870535).
References
- K. V. Ragavan, L. S. Selvakumar and M. S. Thakur, Chem. Commun., 2013, 49, 5960–5962 RSC.
- L. Zhao, Y. Ji, D. Kong, J. Lu, Q. Zhou and X. Yin, Chem. Eng. J., 2016, 303, 458–466 CrossRef CAS.
- L. Lin, A. Uzunoglu and L. A. Stanciu, Small, 2017, 14, 1702828 CrossRef PubMed.
- P. W. Seo, N. A. Khan, Z. Hasan and S. H. Jhung, ACS Appl. Mater. Interfaces, 2016, 8, 29799–29807 CrossRef CAS PubMed.
- Thanhmingliana and D. Tiwari, Chem. Eng. J., 2015, 263, 364–373 CrossRef CAS.
- D. Guignard, V. Gayrard, M. Z. Lacroix, S. Puel, N. Picard-Hagen and C. Viguié, Chemosphere, 2017, 182, 458–467 CrossRef CAS PubMed.
- M. Mon, R. Bruno, J. Ferrando-Soria, D. Armentano and E. Pardo, J. Mater. Chem. A, 2018, 6, 4912–4947 RSC.
- R. Das, C. D. Vecitis, A. Schulze, B. Cao, A. F. Ismail, X. Lu, J. Chen and S. Ramakrishna, Chem. Soc. Rev., 2017, 46, 6946–7020 RSC.
- D. Shetty, I. Jahovic, J. Raya, Z. Asfari, J.-C. Olsen and A. Trabolsi, ACS Appl. Mater. Interfaces, 2018, 10, 2976–2981 CrossRef CAS PubMed.
- Z. Li, M. A. Gondal and Z. H. Yamani, J. Saudi Chem. Soc., 2014, 18, 208–213 CrossRef CAS.
- H. Soni and P. Padmaja, J. Porous Mater., 2014, 21, 275–284 CrossRef CAS.
- S. Li, Y. Gong, Y. Yang, C. He, L. Hu, L. Zhu, L. Sun and D. Shu, Chem. Eng. J., 2015, 260, 231–239 CrossRef CAS.
- S. Bele, V. Samanidou and E. Deliyanni, Chem. Eng. Res. Des., 2016, 109, 573–585 CrossRef CAS.
- N. Genç, Ö. Kılıçoğlu and A. O. Narci, Environ. Technol., 2017, 38, 424–432 CrossRef PubMed.
- Y. Li, F. Jin, C. Wang, Y. Chen, Q. Wang, W. Zhang and D. Wang, Environ. Sci. Pollut. Res., 2015, 22, 8618–8628 CrossRef CAS PubMed.
- M. H. Dehghani, M. Ghadermazi, A. Bhatnagar, P. Sadighara, G. Jahed-Khaniki, B. Heibati and G. McKay, J. Environ. Chem. Eng., 2016, 4, 2647–2655 CrossRef CAS.
- J. Xu, L. Wang and Y. Zhu, Langmuir, 2012, 28, 8418–8425 CrossRef CAS PubMed.
- F. Qin, S. Jia, Y. Liu, H. Li and S. Wu, Desalin. Water Treat., 2015, 54, 93–102 CrossRef CAS.
-
G. Zhu and H. Ren, Porous Organic Frameworks: Design, Synthesis and Their Advanced Applications, Spinger, 2015 Search PubMed.
- X. Zou, H. Ren and G. Zhu, Chem. Commun., 2013, 49, 3925–3936 RSC.
- X. Jing and G. Zhu, Chin. Sci. Bull., 2018, 63, 2215–2228 Search PubMed.
- T. Ben, H. Ren, S. Ma, D. Cao, J. Lan, X. Jing, W. Wang, J. Xu, F. Deng, J. M. Simmons, S. Qiu and G. Zhu, Angew. Chem., Int. Ed., 2009, 48, 9457–9460 CrossRef CAS PubMed.
- H. Ren, T. Ben, F. Sun, M. Guo, X. Jing, H. Ma, K. Cai, S. Qiu and G. Zhu, J. Mater. Chem., 2011, 21, 10348–10353 RSC.
- L. Li, K. Cai, P. Wang, H. Ren and G. Zhu, ACS Appl. Mater. Interfaces, 2015, 7, 201–208 CrossRef CAS PubMed.
- D. Klemm, F. Kramer, S. Moritz, T. Lindström, M. Ankerfors, D. Gray and A. Dorris, Angew. Chem., Int. Ed., 2011, 50, 5438–5466 CrossRef CAS PubMed.
- M. Hamedi, E. Karabulut, A. Marais, A. Herland, G. Nyström and L. Wågberg, Angew. Chem., Int. Ed., 2013, 52, 12038–12042 CrossRef CAS PubMed.
- G. Ye, X. Zhu, S. Chen, D. Li, Y. Yin, Y. Lu, S. Komarneni and D. Yang, J. Mater. Chem. A, 2017, 5, 8247–8254 RSC.
- X. Yang, K. Shi, I. Zhitomirsky and E. D. Cranston, Adv. Mater., 2015, 27, 6104–6109 CrossRef CAS PubMed.
- H. Zhu, X. Yang, E. D. Cranston and S. Zhu, Adv. Mater., 2016, 28, 7652–7657 CrossRef CAS PubMed.
- D. Zhao, J. Huang, Y. Zhong, K. Li, L. Zhang and J. Cai, Adv. Funct. Mater., 2016, 26, 6279–6287 CrossRef CAS.
- Y. Lu, H. Liu, R. Gao, S. Xiao, M. Zhang, Y. Yin, S. Wang, J. Li and D. Yang, ACS Appl. Mater. Interfaces, 2016, 8, 29179–29185 CrossRef CAS PubMed.
- G. Férey, C. Mellot-Draznieks, C. Serre, F. Millange, J. Dutour, S. Surblé and I. Margiolaki, Science, 2005, 309, 2040 CrossRef PubMed.
- M. Zhou, Y. Wu, J. Qiao, J. Zhang, A. McDonald, G. Li and F. Li, J. Colloid Interface Sci., 2013, 405, 157–163 CrossRef CAS PubMed.
- Y. Kinoshita, K. Wakimoto, A. H. Gibbons, A. P. Isfahani, H. Kusuda, E. Sivaniah and B. Ghalei, J. Membr. Sci., 2017, 539, 178–186 CrossRef CAS.
- Y. S. Ho and G. McKay, Process Biochem., 1999, 34, 451–465 CrossRef CAS.
- I. X. García-Zubiri, G. González-Gaitano and J. R. Isasi, J. Colloid Interface Sci., 2009, 337, 11–18 CrossRef PubMed.
- I. A. Udoetok, R. M. Dimmick, L. D. Wilson and J. V. Headley, Carbohydr. Polym., 2016, 136, 329–340 CrossRef CAS PubMed.
- A. Bhatnagar and I. Anastopoulos, Chemosphere, 2017, 168, 885–902 CrossRef CAS PubMed.
- B. Petrie, L. Lopardo, K. Proctor, J. Youdan, R. Barden and B. Kasprzyk-Hordern, Sci. Total Environ., 2019, 650, 900–907 CrossRef CAS PubMed.
- A. Alsbaiee, B. J. Smith, L. Xiao, Y. Ling, D. E. Helbling and W. R. Dichtel, Nature, 2015, 529, 190 CrossRef PubMed.
- B. Liang, H. Wang, X. Shi, B. Shen, X. He, Z. A. Ghazi, N. A. Khan, H. Sin, A. M. Khattak, L. Li and Z. Tang, Nat. Chem., 2018, 10, 961–967 CrossRef CAS PubMed.
Footnote |
† Electronic supplementary information (ESI) available. See DOI: 10.1039/c8ta09644a |
|
This journal is © The Royal Society of Chemistry 2019 |