DOI:
10.1039/C9SC02970B
(Edge Article)
Chem. Sci., 2019,
10, 8577-8582
Hyperpolarization of 15N-pyridinium and 15N-aniline derivatives by using parahydrogen: new opportunities to store nuclear spin polarization in aqueous media†
Received
17th June 2019
, Accepted 30th July 2019
First published on 6th August 2019
Abstract
Hyperpolarization techniques hold the promise to improve the sensitivity of magnetic resonance imaging (MRI) contrast agents by over 10
000-fold. Among these techniques, para-hydrogen induced polarization (PHIP) allows for generating contrast agents within seconds. Typical hyperpolarized contrast agents are traceable for 2–3 minutes only, thus prolonging tracking-times holds great importance for the development of new ways to diagnose and monitor diseases. Here, we report on the design of perdeuterated 15N-containing molecules with longitudinal relaxation times (T1) of several minutes. T1 is a measure for how long hyperpolarization can be stored. In particular, we introduce two new hyperpolarizable families of compounds that we signal enhanced with para-hydrogen: tert-amine aniline derivatives and a quaternary pyridinium compound with 15N-T1 of about 8 minutes. Especially the latter compound has great potential for applicability since we achieved 15N-polarization up to 8% and the pyridinium motif is contained in a variety of drug molecules and is also used in drug delivery systems.
Introduction
Nuclear magnetic resonance (NMR) spectroscopy and magnetic resonance imaging (MRI) are powerful techniques, which have widely been used for studying molecular structures associated to diseases and to visualize illnesses even in vivo.1–4 Both techniques are greatly hampered, due to their low sensitivity. This limitation can be overcome by using hyperpolarization methods, which increase signals of molecules by more than four orders of magnitude.5–13 Several hyperpolarization techniques have evolved to gain new insights e.g. in the fields of structural biology, material science, chemical analysis, biochemistry and biomedical science. With a view on the latter, hyperpolarization allows for creating new contrast agents to study and diagnose diseases in vivo.14 The technique mainly used for producing hyperpolarized contrast agents is dissolution dynamic nuclear polarization (d-DNP).5 It enables the hyperpolarization of metabolically active compounds that can be followed during in vivo studies.8,9,11,14–16 Other methods with biomedical relevance are spin exchange optical pumping (SEOP)17–20 of noble gases and para-hydrogen induced polarization (PHIP).21–32 PHIP methods transfer nuclear spin order from para-hydrogen (para-H2) enriched hydrogen over to target molecules for their hyperpolarization. Hydrogenative PHIP adds para-H2 to unsaturated precursors over suitable hydrogenation catalysts, to create large spin-order in the target compounds, which can be converted into observable magnetization afterwards. Due to the design of suitable precursor molecules, this technique can now be utilized to hyperpolarize metabolically active compounds and to analyze their chemical conversion in vivo.28,31,32
Within the past ten years, a non-hydrogenative para-H2-based hyperpolarization methods has evolved: signal amplification by reversible exchange (SABRE).32–37 For this method, para-H2 and a substrate of interest coordinate to a temporarily stable transition metal complex. In this complex, the para-H2 spin order is converted into observable magnetization at the molecule of interest. Dissociation of the complex leads to free hyperpolarized substrates that have not been altered as in the classical PHIP approach.38 However, this method has not been shown to be applicable for in vivo applications yet since, SABRE experiments typically need to be performed in organic solvents. However, the field rapidly progressing and work is on the way to make this technique more biologically applicable in the future.32,39,40
What all techniques have in common is the desire to store hyperpolarization in contrast agents for long periods of time. To this end, hyperpolarization is typically stored on hetero-nuclei such as in 13C and 15N, which possess longitudinal relaxation times (T1) ranging from seconds to minutes. The T1 of 13C-pyruvate, the metabolite most commonly hyperpolarized, for example is in the range of 40–60 s.41 For in vivo applications, this results in a time window of 2–3 minutes, during which pyruvate can be monitored.11,14,41 To increase tracing times, 15N nuclei are more favorable than 13C nuclei since T1 can be one order of magnitude longer and T1 > 1200 s (20 minutes) in water have been reported in quaternary nitrogen compounds.30 Due to its longer T1 values, 15N-derived chemical probes have been explored: with respect to PHIP N-ethyl trimethyl ammonium (NETMA) and an allyl choline derivative have been polarized in biocompatible solvents.30,42,43 Dissolution DNP has demonstrated first in vivo experiments utilizing 15N polarized choline and several other applications in vitro such as pH-sensing, Ca2+ monitoring and enzyme activity.44–46 Degrees of 15N-polarization have long been rather low until the advancements in cross-polarization (CP) d-DNP have overcome this challenge.13
SABRE has made great progress in polarizing 15N spins in the past years.34,35,47 Demonstrations of over 40% polarization in 15N pyridine and more than 30% for imidazole have been accomplished in methanol.48,49 Prospective applications may include pH-sensing50 or probing of hypoxia.47,49 The later may in particular become feasible via storage of polarization in a 15N-nitro group of metronidazole which has a T1 of about 10 minutes in methanol.51
Currently the main challenge is to discover molecules that are biological relevant, have long T1 and can be hyperpolarized to a large degree. Here, we are tackling this challenge and introduce classes of compounds that meet these requirements. Our particular focus is thereby on pyridinium, a compound already relevant in drug applications.52–56
Experimental
The synthesis of the labelled compounds was conducted as follows: to yield 1, we prepared 15N-pyridine-d5 starting from protonated 15N-pyridine, oxidation with meta-chloroperoxybenzoic acid (m-CPBA) followed by H–D exchange reaction under microwave condition in D2O (Scheme 1A). Further reduction with PCl3 in CH2Cl2 yielded 15N-pyridine-d5.57 Finally, quaternization of 5 was accomplished by the treatment with allyl bromide-d5 (6) in EtOAc to yield 1 as colourless solid.58 In order to synthesize the aniline derivatives 2 and 3, we first synthesized 15N-aniline-d5 (7) in a two-step procedure from benzene-d5.59 Mono-allylation of 7 with allyl bromide-d5 (6) in the presence of K2CO3 and further treatment with CD3I in presence of DIPEA, yielded 2 (Scheme 1B). Stirring of 2 in neat CD3I leads to the quaternary aniline derivative 3. Further experimental details can be found in the ESI.†
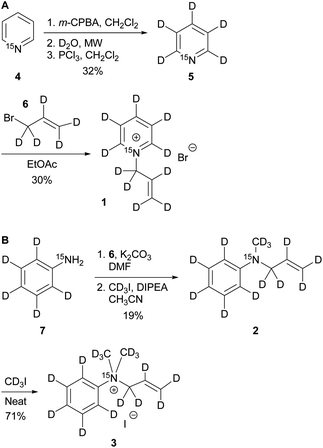 |
| Scheme 1 Syntheses of 15N-pyridinium derivative (A) and -aniline based (B) derivatives; MW: microwave. | |
Result and discussion
We have synthesized and investigated a library of 15N-enriched compounds and report on two novelties: firstly, we have discovered an aniline derivative containing a tertiary amine with a long T1 of about 10 minutes in methanol-d4 (MeOD). This is of particular interest since it demonstrates that uncharged nitrogen species, in addition to quaternary compounds, have potential to store polarization for long periods and opens up new possibilities to design contrast agents with lipophilic moieties. Secondly, we are introducing a new class of compounds that can be hyperpolarized and possesses a T1 of about 8 minutes in water: quaternary pyridinium derivatives. Quaternary pyridinium is a core structure found in many molecules which has been used for investigations of neurodegenerative diseases60 as well as in drug design and drug-delivery approaches.52–56 We furthermore present the hyperpolarization of the library of compounds via PHIP and a pulsed transfer method to enhance the 15N signals. Generating contrast agents in aqueous media becomes possible by utilizing rhodium nano-catalysts (NAC@Rh) that promote the hydrogenation reaction with para-H2 in water.30
Table 1 presents the investigated compounds and at the top the general scheme of how the investigated compounds are hyperpolarized with para-H2. The precursor compounds prior to hydrogenation are a pyridinium derivative (1), a tert-amine derivative of aniline (2) and a quaternary nitrogen derivative of aniline (3). We have perdeuterated all of the precursors to prolong 15N-T1 by weakening dipolar couplings, as compared to the protonated counterparts. As an unsaturated moiety to which para-H2 will be added during the hydrogenation step, we have chosen deuterated allyl groups. The rationale behind this choice is twofold: first, the added protons from para-H2 after the hydrogenation will be one extra bond away as compared to the vinyl derivatives, thus reducing dipolar interactions that potentially shorten T1. Second, the scalar coupling network in the hydrogenation products 1a–3a are thought to be an ideal spin system to apply the recently developed ESOTHERIC (efficient spin order transfer to heteronuclei via relayed INEPT chains) spin order transfer sequence to hyperpolarize the 15N spins.61,62 This is because the 3JH,N coupling is larger than 4JH,N (see ESI†) and the protons are weakly coupled.
Table 1
15N-T1 values for pyridinium and phenylammonium compounds along with their reduced products using para-H2a
Prior to performing hyperpolarization experiments, we determined 15N-T1 for the unsaturated precursor molecules 1–3 in D2O, MeOD or mixtures thereof to increase the molecule's solubility. The 15N-T1 values obtained in different solvents and at various magnetic fields are summarized in Table 1. For the precursor molecules it is noteworthy to mention that 15N-T1 of the tert-amine 2 has a 15N-T1 of 570 ± 40 s in MeOD (this compound was not soluble in water) at high field and the quaternary ammonium compound 3 displays a 15N-T1 of 420 ± 100 s in D2O. For the unsaturated pyridinium derivative 1, we discovered a 15N-T1 of 220 ± 30 s at high field in D2O.
Since we found 15N-T1 values of several minutes for all precursor compounds, we performed hydrogenation reactions and investigated 15N-T1 of the hydrogenation products. This was done by hyperpolarizing the 15N nuclei and measuring the polarization decay with low flip angle pulses as described in the next paragraph and in the ESI.† Our first observation was that the anilinium derivative 3a decomposes upon hydrogenation. This may reflect that trimethylanilinium is typically used as a methylation agent63 and not stable enough for hyperpolarization studies with para-H2. Moreover, a similar kind of degradation was reported on 15N-propargylcholine while performing PHIP.42 In addition to this, Shchepin et. al. reported lack of the successful 15N hyperpolarization on other choline derivatives using 15N-enriched PHIP precursors.64 The 15N-T1 of the tert-amine 2a is strongly reduced after hydrogenation to 150 ± 20 s. Lastly, the pyridinium derivative 1a has a T1 of 120 ± 10 s at high field in D2O, but reaches 500 ± 30 s (about 8 minutes) when the field is lowered to 0.1 T (see also Fig. S1†). With respect to T1, the main relaxation source at high field appears to be chemical shift anisotropy (CSA). This offers possibilities to make the compound applicable for studies in clinical scanners. Given its long 15N-T1 at low field in water and being an important structure in a variety of biomolecules or drugs, the pyridinium derivative is the most promising compound discovered among the investigated compounds here for future applications.
To obtain the hyperpolarized products, compounds 1–3 were hydrogenated with para-H2 under two experimental conditions: for preparation in MeOD, we used the homogeneous Rh-catalyst [Rh(dppb)(COD)][BF4] (dppb: diphenylphosphino butane, COD: cyclooctadiene). For hyperpolarization in D2O, we used an N-acetylcysteine-capped Rh-nano-catalysts (NAC@Rh).30 The enrichment of H2 in its para-state was 80%, as determined experimentally. At first, we have investigated the 1H polarization and subsequently the 15N polarization following the ESOTHERIC sequence.61,62 The results are summarized in Table 2.
Table 2 PHIP enhancements by using the homogenous and heterogeneous catalysts
|
Compd. |
1H P% |
15N P% |
Multiple products or decomposition.
Measured in MeOD : D2O (1 : 1) at 320 K. Compd.: compound.
|
PHIP (homogeneous) MeOD at 320 K |
1a
|
11 ± 1.3 |
7.4 ± 0.6 |
2a
|
—a |
—a |
3a
|
—a |
—a |
PHIP (heterogeneous) D2O at 353 K |
1a
|
2.1 ± 1.2 |
2.3 ± 1.1 |
2a
|
1.3 ± 0.2b |
0.8 ± 0.1b |
3a
|
—a |
—a |
As compound 3a did not form during hydrogenation, no hyperpolarization data is reported here for either the homogeneous or heterogeneous catalyst. Compound 2 turned out to be insoluble in D2O; therefore, we chose an equimolar mixture of MeOD and D2O for dissolving the heterogeneous catalyst for PHIP experiments. We have found 1% polarization of 1H and 15N nuclei respectively in the hydrogenated compound 2a, whereas multiple polarized products were observed in MeOD with the homogeneous catalyst. This result demonstrates that heterogeneous catalysts provide new opportunities for polarizing nitrogen containing compounds that may not be accessible with the standard homogeneous catalyst.
With respect to the pyridinium derivative, we observed significant 1H polarization of 11% ± 1.3% in 1a using the homogeneous catalyst in MeOD. We succeeded in transferring this polarization to the 15N-spin with a signal enhancement (ε) of 32
000 (P = 7.4% ± 0.6%) compared to thermal polarization at B0 = 7 T at 320 K in MeOD. For improved biocompatibility, we performed polarization experiments with the heterogeneous catalyst in water and achieved a highest polarization of 3.1% (ε = 15
000-fold compared to the thermal signal at 353 K, Fig. 1) and an average 2.3% polarization. The spectrum of the hyperpolarized compound in water as well as the T1-experiment (inset) with small tip angle pulses at 0.1 T is depicted in Fig. 1.
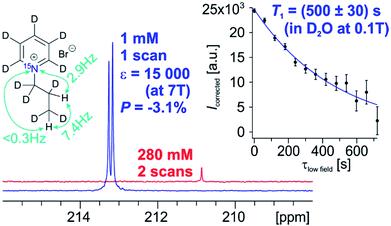 |
| Fig. 1 Hyperpolarized 15N spectrum of 1a (blue) by using PHIP in D2O and thermally polarized 15N spectrum of the unsaturated precursor 1 (red) at 7 T. The inset shows the 15N-T1 relaxation data measured at 0.1 T, using a hyperpolarized sample of 1a which was collected using sample shuttling and small flip angle pulses (see ESI† for further details). | |
Conclusions
In conclusion, we have introduced and synthesized perdeuterated 15N-allyl-pyridinium (1) and -aniline derivatives (2 & 3). We succeeded in forming hyperpolarized addition products of 1 and 2 utilizing para-H2. Most notably, a 15N-pyridinium derivative (1a) provided strong 15N-polarization of P = 7.4% in methanol and P = 2.3% in water compared to thermal polarization. Polarization in water was achieved via rhodium nanocatalysts that although heterogeneous PHIP catalysts are still in an early development stage show here the possibility to signal enhance molecules that are not polarizable with standard homogeneous metal complexes. In water at 0.1 T field, we discovered a long 15N-T1 of about 8 min. We also found that the tert-amine 2 features notably a slow relaxation time of 10 min for 15N-nuclei in methanol. This is despite the fact that it is not a quaternary nitrogen compound, and thus could be used as a hydrophobic 15N-labelled tracer. Overall, our presented studies introduce new possibilities for the molecular design of contrast agents and storage capabilities of hyperpolarized spin states. It is noteworthy to mention that out of all compounds studied here, the highest levels of hyperpolarization (1H and 15N) were found in pyridinium derivatives, a molecular species present in many bio-relevant molecules. Longer relaxation times of 15N nuclei of these compounds in combination with targeting moieties will potentially in the future ensure long traceability and opportunity to deliver the hyperpolarization in organisms for biomedical imaging applications.
Conflicts of interest
There are no conflicts to declare.
Acknowledgements
We gratefully thank the Max-Planck-Society and the Max-Planck-Institute for Biophysical Chemistry for generous funding and Prof. Christian Griesinger for access to his equipment and facilities. The authors furthermore thank Dr Sergey Korchak and Dr Salvatore Mamone for support. We also thank Dr V. Belov and the chemical synthesis facility for performing the microwave reaction.
Notes and references
-
E. M. Haacke, R. W. Brown, M. R. Thompson and R. Venkatesan, Magnetic Resonance Imaging: Physical Principles and Sequence Design, Wiley-Liss, New York, 1999 Search PubMed.
-
R. R. Edelman, Clinical Magnetic Resonance Imaging, Saunders Elsevier, 2006 Search PubMed.
- Y. D. Xiao, R. Paudel, J. Liu, C. Ma, Z. S. Zhang and S. K. Zhou, Int. J. Mol. Med., 2016, 38, 1319–1326 CrossRef CAS PubMed.
- E. Mormina, M. Petracca, G. Bommarito, N. Piaggio, S. Cocozza and M. Inglese, World J. Radiol., 2017, 9, 371–388 CrossRef PubMed.
- J. H. Ardenkjær-Larsen, B. Fridlund, A. Gram, G. Hansson, L. Hansson, M. H. Lerche, R. Servin, M. Thaning and K. Golman, Proc. Natl. Acad. Sci. U. S. A., 2003, 100, 10158–10163 CrossRef PubMed.
- K. Golman and M. Thaning, Proc. Natl. Acad. Sci. U. S. A., 2006, 103, 11270–11275 CrossRef CAS PubMed.
- S. E. Day, M. I. Kettunen, F. A. Gallagher, D.-E. Hu, M. Lerche, J. Wolber, K. Golman, J. H. Ardenkjaer-Larsen and K. M. Brindle, Nat. Med., 2007, 13, 1382–1387 CrossRef CAS PubMed.
- F. A. Gallagher, M. I. Kettunen, S. E. Day, D.-E. Hu, J. H. Ardenkjær-Larsen, P. R. Jensen, M. Karlsson, K. Golman, M. H. Lerche and K. M. Brindle, Nature, 2008, 453, 940–943 CrossRef CAS PubMed.
- J. Kurhanewicz, D. B. Vigneron, K. Brindle, E. Y. Chekmenev, A. Comment, C. H. Cunningham, R. J. DeBerardinis, G. G. Green, M. O. Leach and S. S. Rajan, Neoplasia, 2011, 13, 81–97 CrossRef CAS PubMed.
- S. Jannin, A. Bornet, R. Melzi and G. Bodenhausen, Chem. Phys. Lett., 2012, 549, 99–102 CrossRef CAS.
- S. J. Nelson, J. Kurhanewicz, D. B. Vigneron, P. E. Larson, A. L. Harzstark, M. Ferrone, M. van Criekinge, J. W. Chang, R. Bok and I. Park, Sci. Transl. Med., 2013, 5, 198ra108 Search PubMed.
- X. Ji, A. Bornet, B. Vuichoud, J. Milani, D. Gajan, A. J. Rossini, L. Emsley, G. Bodenhausen and S. Jannin, Nat. Commun., 2017, 8, 13975 CrossRef CAS PubMed.
- J. Milani, B. Vuichoud, A. Bornet, R. Melzi, S. Jannin and G. Bodenhausen, Rev. Sci. Instrum., 2017, 88, 015109 CrossRef PubMed.
- K. Golman, M. Lerche, R. Pehrson and J. H. Ardenkjaer-Larsen, Cancer Res., 2006, 66, 10855–10860 CrossRef CAS PubMed.
- C. Gabellieri, S. Reynolds, A. Lavie, G. S. Payne, M. O. Leach and T. R. Eykyn, J. Am. Chem. Soc., 2008, 130, 4598–4599 CrossRef CAS PubMed.
- J. J. Miller, J. T. Grist, S. Serres, J. R. Larkin, A. Z. Lau, K. Ray, K. R. Fisher, E. Hansen, R. S. Tougaard, P. M. Nielsen, J. Lindhardt, C. Laustsen, F. A. Gallagher, D. J. Tyler and N. Sibson, Sci. Rep., 2018, 8, 15082 CrossRef PubMed.
- T. G. Walker and W. Happer, Rev. Mod. Phys., 1997, 69, 629–642 CrossRef CAS.
- S. Appelt, A. B.-A. Baranga, C. Erickson, M. Romalis, A. Young and W. Happer, Phys. Rev. A: At., Mol., Opt. Phys., 1998, 58, 1412–1439 CrossRef CAS.
- H. E. Möller, X. J. Chen, B. Saam, K. D. Hagspiel, G. A. Johnson, T. A. Altes, E. E. De Lange and H. U. Kauczor, Magn. Reson. Med., 2002, 47, 1029–1051 CrossRef PubMed.
- L. Schröder, T. J. Lowery, C. Hilty, D. E. Wemmer and A. Pines, Science, 2006, 314, 446–449 CrossRef PubMed.
- C. R. Bowers and D. P. Weitekamp, Phys. Rev. Lett., 1986, 57, 2645–2648 CrossRef CAS PubMed.
- C. R. Bowers and D. P. Weitekamp, J. Am. Chem. Soc., 1987, 109, 5541–5542 CrossRef CAS.
- T. C. Eisenschmid, R. U. Kirss, P. P. Deutsch, S. I. Hommeltoft, R. Eisenberg, J. Bargon, R. G. Lawler and A. L. Balch, J. Am. Chem. Soc., 1987, 109, 8089–8091 CrossRef CAS.
- K. Golman, O. Axelsson, H. Jóhannesson, S. Månsson, C. Olofsson and J. S. Petersson, Magn. Reson. Med., 2001, 46, 1–5 CrossRef CAS PubMed.
- E. Y. Chekmenev, J. Hövener, V. A. Norton, K. Harris, L. S. Batchelder, P. Bhattacharya, B. D. Ross and D. P. Weitekamp, J. Am. Chem. Soc., 2008, 130, 4212–4213 CrossRef CAS PubMed.
- S. Glöggler, A. M. Grunfeld, Y. N. Ertas, J. McCormick, S. Wagner, P. P. M. Schleker and L. S. Bouchard, Angew. Chem., Int. Ed., 2015, 54, 2452–2456 CrossRef PubMed.
- O. G. Salnikov, K. V. Kovtunov and I. V. Koptyug, Sci. Rep., 2015, 5, 13930 CrossRef PubMed.
- F. Reineri, T. Boi and S. Aime, Nat. Commun., 2015, 6, 5858 CrossRef CAS PubMed.
- A. Schmidt, S. Berner, W. Schimpf, C. Müller, T. Lickert, N. Schwaderlapp, S. Knecht, J. Skinner, A. Dost and P. Rovedo, Nat. Commun., 2017, 8, 14535 CrossRef CAS PubMed.
- J. McCormick, S. Korchak, S. Mamone, Y. N. Ertas, Z. Liu, L. Verlinsky, S. Wagner, S. Glöggler and L.-S. Bouchard, Angew. Chem., Int. Ed., 2018, 57, 10692–10696 CrossRef CAS PubMed.
- E. Cavallari, C. Carrera, M. Sorge, G. Bonne, A. Muchir, S. Aime and F. Reineri, Sci. Rep., 2018, 8, 8366 CrossRef PubMed.
- J.-B. Hövener, A. N. Pravdivtsev, B. Kidd, C. R. Bowers, S. Glöggler, K. V. Kovtunov, M. Plaumann, R. Katz-Brull, K. Buckenmaier, A. Jerschow, F. Reineri, T. Theis, R. V. Shchepin, S. Wagner, P. Bhattacharya, N. M. Zacharias and E. Y. Chekmenev, Angew. Chem., Int. Ed., 2018, 57, 11140–11162 CrossRef PubMed.
- R. W. Adams, J. A. Aguilar, K. D. Atkinson, M. J. Cowley, P. I. Elliott, S. B. Duckett, G. G. Green, I. G. Khazal, J. López-Serrano and D. C. Williamson, Science, 2009, 323, 1708–1711 CrossRef CAS PubMed.
- T. Theis, M. L. Truong, A. M. Coffey, R. V. Shchepin, K. W. Waddell, F. Shi, B. M. Goodson, W. S. Warren and E. Y. Chekmenev, J. Am. Chem. Soc., 2015, 137, 1404–1407 CrossRef CAS PubMed.
- T. Theis, G. X. Ortiz, A. W. Logan, K. E. Claytor, Y. Feng, W. P. Huhn, V. Blum, S. J. Malcolmson, E. Y. Chekmenev and Q. Wang, Sci. Adv., 2016, 2, e1501438 CrossRef PubMed.
- P. J. Rayner, M. J. Burns, A. M. Olaru, P. Norcott, M. Fekete, G. G. R. Green, L. A. R. Highton, R. E. Mewis and S. B. Duckett, Proc. Natl. Acad. Sci. U. S. A., 2017, 114, E3188–E3194 CrossRef CAS PubMed.
- M. Suefke, S. Lehmkuhl, A. Liebisch, B. Blümich and S. Appelt, Nat. Phys., 2017, 13, 568–572 Search PubMed.
- R. A. Green, R. W. Adams, S. B. Duckett, R. E. Mewis, D. C. Williamson and G. G. R. Green, Prog. Nucl. Magn. Reson. Spectrosc., 2012, 67, 1–48 CrossRef CAS PubMed.
- J. F. P. Colell, M. Emondts, A. W. J. Logan, K. Shen, J. Bae, R. V. Shchepin, G. X. Ortiz Jr, P. Spannring, Q. Wang, S. J. Malcolmson, E. Y. Chekmenev, M. C. Feiters, F. P. J. T. Rutjes, B. Blümich, T. Theis and W. S. Warren, J. Am. Chem. Soc., 2017, 139, 7761–7767 CrossRef CAS PubMed.
- P. J. Rayner and S. B. Duckett, Angew. Chem., Int. Ed., 2018, 57, 6742–6753 CrossRef CAS PubMed.
- J. Kurhanewicz, D. B. Vigneron, J. H. Ardenkjaer-Larsen, J. A. Bankson, K. Brindle, C. H. Cunningham, F. A. Gallagher, K. R. Keshari, A. Kjaer, C. Laustsen, D. A. Mankoff, M. E. Merritt, S. J. Nelson, J. M. Pauly, P. Lee, S. Ronen, D. J. Tyler, S. S. Rajan, D. M. Spielman, L. Wald, X. Zhang, C. R. Malloy and R. Rizi, Neoplasia, 2019, 21, 1–16 CrossRef PubMed.
- F. Reineri, A. Viale, S. Ellena, D. Alberti, T. Boi, G. B. Giovenzana, R. Gobetto, S. S. Premkumar and S. Aime, J. Am. Chem. Soc., 2012, 134, 11146–11152 CrossRef CAS PubMed.
- L. B. Bales, K. V. Kovtunov, D. A. Barskiy, R. V. Shchepin, A. M. Coffey, L. M. Kovtunova, A. V. Bukhtiyarov, M. A. Feldman, V. I. Bukhtiyarov and E. Y. Chekmenev, J. Phys. Chem. C, 2017, 121, 15304–15309 CrossRef CAS PubMed.
- H. Nonaka, R. Hata, T. Doura, T. Nishihara, K. Kumagai, M. Akakabe, M. Tsuda, K. Ichikawa and S. Sando, Nat. Commun., 2013, 4, 2411 CrossRef PubMed.
- W. Jiang, L. Lumata, W. Chen, S. Zhang, Z. Kovacs, A. D. Sherry and C. Khemtong, Sci. Rep., 2015, 5, 9104 CrossRef PubMed.
- C. Cudalbu, A. Comment, F. Kurdzesau, R. B. van Heeswijk, K. Uffmann, S. Jannin, V. Denisov, D. Kirik and R. Gruetter, Phys. Chem. Chem. Phys., 2010, 12, 5818–5823 RSC.
- D. A. Barskiy, R. V. Shchepin, A. M. Coffey, T. Theis, W. S. Warren, B. M. Goodson and E. Y. Chekmenev, J. Am. Chem. Soc., 2016, 138, 8080–8083 CrossRef CAS PubMed.
- P. J. Rayner, P. Norcott, K. M. Appleby, W. Iali, R. O. John, S. J. Hart, A. C. Whitwood and S. B. Duckett, Nat. Commun., 2018, 9, 4251 CrossRef PubMed.
- B. E. Kidd, J. L. Gesiorski, M. E. Gemeinhardt, R. V. Shchepin, K. V. Kovtunov, I. V. Koptyug, E. Y. Chekmenev and B. M. Goodson, J. Phys. Chem. C, 2018, 122, 16848–16852 CrossRef CAS PubMed.
- R. V. Shchepin, D. A. Barskiy, A. M. Coffey, T. Theis, F. Shi, W. S. Warren, B. M. Goodson and E. Y. Chekmenev, ACS Sensors, 2016, 1, 640–644 CrossRef CAS PubMed.
- R. V. Shchepin, J. R. Birchall, N. V. Chukanov, K. V. Kovtunov, I. V. Koptyug, T. Theis, W. S. Warren, J. G. Gelovani, B. M. Goodson, S. Shokouhi, M. S. Rosen, Y.-F. Yen, W. Pham and E. Y. Chekmenev, Chem.–Eur. J., 2019, 25, 8829–8836 CAS.
- J. W. Simpkins and N. Bodor, Adv. Drug Delivery Rev., 1994, 14, 243–249 CrossRef CAS.
- A. Friedman, D. Kaufer, J. Shemer, I. Hendler, H. Soreq and I. Tur-Kaspa, Nat. Med., 1996, 2, 1382–1385 CrossRef CAS PubMed.
- B. Pavan, A. Dalpiaz, N. Ciliberti, C. Biondi, S. Manfredini and S. Vertuani, Molecules, 2008, 13, 1035–1065 CrossRef CAS PubMed.
- J. Rautio, K. Laine, M. Gynther and J. Savolainen, AAPS J., 2008, 10, 92–102 CrossRef CAS PubMed.
- C. Fromm-Dornieden, J.-D. Rembe, N. Schäfer, J. Böhm and E. K. Stuermer, J. Med. Microbiol., 2015, 64, 407–414 CrossRef CAS PubMed.
- P. J. Rayner, M. J. Burns, A. M. Olaru, P. Norcott, M. Fekete, G. G. Green, L. A. Highton, R. E. Mewis and S. B. Duckett, Proc. Natl. Acad. Sci. U. S. A., 2017, 114, E3188–E3194 CrossRef CAS PubMed.
- A. C. Tibbits, Y. S. Yan and C. J. Kloxin, Macromol. Rapid Commun., 2017, 38, 1700113 CrossRef PubMed.
- H. Nonaka, M. Hirano, Y. Imakura, Y. Takakusagi, K. Ichikawa and S. Sando, Sci. Rep., 2017, 7, 40104 CrossRef CAS PubMed.
- P. N. Jethva, J. R. Kardani and I. Roy, FEBS J., 2011, 278, 1688–1698 CrossRef CAS PubMed.
- S. Korchak, S. J. Yang, S. Mamone and S. Glöggler, ChemistryOpen, 2018, 7, 344–348 CrossRef CAS PubMed.
- S. Korchak, S. Mamone and S. Glöggler, ChemistryOpen, 2018, 7, 672–676 CrossRef CAS PubMed.
- S. W. Ragsdale, Vitam. Horm., 2008, 79, 293–324 CAS.
- R. V. Shchepin and E. Y. Chekmenev, J. Labelled Compd. Radiopharm., 2013, 56, 655–662 CrossRef CAS PubMed.
Footnote |
† Electronic supplementary information (ESI) available: Synthetic procedures & characterization, NMR experimental description, computation of 1H and 15N enhancements. See DOI: 10.1039/c9sc02970b |
|
This journal is © The Royal Society of Chemistry 2019 |