DOI:
10.1039/C9SC01002E
(Edge Article)
Chem. Sci., 2019,
10, 5319-5325
1-Titanacyclobuta-2,3-diene – an elusive four-membered cyclic allene†
Received
27th February 2019
, Accepted 20th April 2019
First published on 23rd April 2019
Abstract
The synthesis of an unusual 1-metalla-2,3-cyclobutadiene complex [rac-(ebthi)Ti(Me3SiC3SiMe3)] (rac-ebthi = rac-1,2-ethylene-1,1′-bis(η5-tetrahydroindenyl)), a formal metallacyclic analogue of a non-existent four-membered 1,2-cyclobutadiene, is described. By variation of the cyclopentadienyl ligand of the titanocene precursor it was possible to stabilise this highly exotic compound which selectively reacts with ketones and aldehydes to yield enynes by oxygen transfer to titanium. Analysis of the bonding and electronic structure of the metallacycle shows that the complex is best described as an unusual antiferromagnetically coupled biradicaloid system, possessing a formal Ti(III) centre coordinated with a monoanionic radical ligand.
Introduction
Driven by the “desire to make the molecule that violates the norm”1 chemists have always looked into possibilities to stabilise exotic molecules and explore the reasons for their existence and reactivities. In this respect, a commonly used approach is the incorporation of unsaturation into cyclic structures that increases the ring strain and therefore in principle decreases the likeliness of its existence.2 For a long time, organometallic chemists have investigated metallacycles, both, with respect to their unusual coordination environments as well as potential applications in stoichiometric3,4 and catalytic transformations.4,5 In the past, a number of five-membered metallacycles based on early and late transition metals have been reported that possess rather unusual structural motifs, including Rosenthal's 1-metallacyclopenta-2,3,4-trienes,6 Suzuki's 1-metallacyclopent-3-ynes,7 Erker's 1-metallacyclopenta-3,4-dienes8 (all based on group 4 metals) or Xi's metalloles (Rh, Pd, Pt).9 Intuitively, the synthesis of four-membered and highly unsaturated metallacycles should be even more challenging and to date a common approach to stabilise such structures is the incorporation of heteroatoms into the ring system.10 For example, the parent 1,2-cyclobutadiene has a calculated strain energy of 74.9 kcal mol−1,11 thus reflecting the instability and the difficulty in isolating a molecule of this type. The chemistry of 1-metallacyclobuta-2,3-dienes has been investigated in the past as such structures play a role for catalyst deactivation in alkyne metathesis. Schrock reported on two structurally characterised examples of “all-C-deprotiometallacyclobutadienes” [Mo{C3-(tBu)2}{OCH(CF3)2}2(py)2] (A)12 and [CpW{C3(tBu)2}-Cl] (B)13 (Cp = η5-cyclopentadienyl) that were formed during metathesis of terminal alkynes (Fig. 1). Later, Fürstner (C) and – very recently Tamm (D) – observed the formation of 1-molybdacyclobuta-2,3-dienes as a product of reaction of an alkylidene catalyst with a terminal alkyne.14
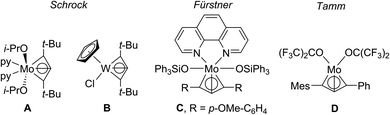 |
| Fig. 1 Structurally characterised 1-metallacyclobuta-2,3-diene complexes. Bonding situations in the metallacycle are depicted as shown in the literature. | |
To further explore the frontiers of the chemistry of group 4 metallacycles Jemmis, Schulz and Rosenthal have computationally investigated the possibilities to stabilise planar 1-metalla-2,3-cyclobutadienes and found that the incorporation of electron-donating substituents at α-carbon atoms could be beneficial.11 Later, our group has attempted to synthesise such structures by coupling of alkynyl and isonitrile ligands at titanocene, unfortunately this approach only resulted in redox-disproportionation of the Ti centre.15 Also, deprotonation of α-CH2SiMe3 and α-CH2N(SiMe3)2 substituted titanocene alkyne complexes was found to be unsuccessful.16 A more promising approach appeared to be the initial construction of a C3 framework prior to coordination to the metal. We have thus revisited and optimised the synthesis of a previously reported 1,3-dilithioallene precursor [Li2(Me3SiC3SiMe3)]17 and reacted this with [Cp2ZrCl2]. To our surprise, we obtained two unusual allenediide bridged dizirconocene complexes [Cp2Zr(RC3R)2ZrCp2] and [Cp2Zr(Cl)RC3R(Cl)ZrCp2] (R = SiMe3),18 the latter being a promising precatalyst for amine borane dehydropolymerisation.19 Interestingly, we were able to show that the dizirconacyclooctatetraene [Cp2Zr(RC3R)2ZrCp2] degrades under mass spectrometry conditions into the desired mononuclear 1-zirconacyclobuta-2,3-diene compound. In this contribution, we report on the synthesis and characterisation of the first 1-metalla-2,3-cyclobutadiene complex of a group 4 metal as well as the reactivity of this unusual complex.
Results and discussion
Reaction of [Cp2TiCl2] and
with the 1,3-dilithioallene precursor
To evaluate the influence of the metal centre we first adapted our previously reported procedure18 and reacted [Cp2TiCl2] with [Li2(Me3SiC3SiMe3)]. 1H NMR analysis of an aliquot taken after a reaction time of 16 hours at room temperature shows the formation of several Cp containing products along with a large number of resonances in the SiMe3 region of the spectrum, out of which we identified the coupling product 1 (cf. p. S4, Fig. S1†). Even at low temperatures (−40 °C), only similar product mixtures could be isolated. We next employed more electron-donating, sterically more demanding
(Cp* = η5-pentamethylcyclopentadienyl) and observed a slow colour change from red to brown. NMR analysis of the reaction mixture shows the formation of unidentified titanocene species along with the coupling product 1 as the main species, which can be isolated after column chromatographic workup in 90% yield (Scheme 1). The formation of 1 can be formally seen as the dimerisation of two carbenes in the coordination sphere of Ti. A similar type of carbene coupling to form alkynyl functionalised symmetrical olefins was reported before by Casey and co-workers for a Re system.20 Also, the formation of Ph4C6 by thermal/radical degradation of 1,3-bis(alkylseleno)allenes supports this proposal.21
 |
| Scheme 1 Reaction of with [Li2(Me3SiC3SiMe3)]. | |
In a previous study we had computed the isodesmic exchange reaction of
and the allene precursor Me(Me3Si)C3(SiMe3)Me to form the desired four-membered metallacycle and ethane. This reaction is slightly exergonic and thus in principle feasible (ΔG = −1.75 kcal mol−1,22). This approach was chosen as a theoretical model for salt metathesis using a 1,3-dilithioallene to avoid solubility effects and cluster formation that would complicate computational analysis. For the reaction of [Cp2TiCl2] with [Li2(Me3SiC3SiMe3)] at low temperature we proposed formation of a 1-titana-2,3-cyclobutadiene (vide supra), which is in accordance with the computed exergonic reaction of Cp2TiMe2 and Me(Me3Si)C3(SiMe3)Me (ΔG = −11.77 kcal mol−1). The fact that a coupling reaction leading to 1 was observed in reactions with Cp and Cp* complexes indicates that at some stage of the reaction the interaction of an intermediately formed C3 framework with the Ti centre occurred. ansa-Cp complexes in many cases show reactivities that are comparable to Cp analogues and furthermore exhibit additional stabilisation of the metallocene through the bridging unit. Subtle differences in reactivity (e.g. formation of different products or faster conversion) are often referred to as the ansa effect.23 In line with this, the reaction of [rac-(ebthi)TiMe2] and Me(Me3Si)C3(SiMe3)Me is even more thermodynamically favourable (ΔG = −16.60 kcal mol−1).
Synthesis and characterisation of 1-titanacyclobuta-2,3-diene (2)
We have thus next reacted [rac-(ebthi)TiCl2] and [Li2(Me3SiC3SiMe3)] at room temperature in pentane (Scheme 2) and observed formation of a deep red solution, from which compound 2 could be isolated as red crystals after removal of LiCl. Moderate yields of 2 (58%) can be explained by the formation of 1 as a byproduct which was identified by NMR spectroscopy and this nicely demonstrates the subtle differences in reactivity along the series Cp–rac-(ebthi)–Cp*. Single crystals suitable for X-ray analysis were obtained from a saturated pentane solution at room temperature.
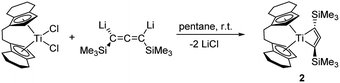 |
| Scheme 2 Reaction of [rac-(ebthi)TiCl2] with [Li2(Me3SiC3SiMe3)] and formation of 2. | |
Compound 2 shows the expected characteristic 1H NMR signals at 0.35, 5.27, and 7.22 ppm, corresponding to the SiMe3 groups and the Cp protons of the rac-(ebthi) ligand. The 13C NMR spectra show two signals at low field that we assign to the internal (δ 134.2 ppm) and metal-bound carbon atoms (δ 213.8 ppm) of the metallacycle. Notably, these values strongly differ from those found for the previously reported Mo and W complexes, pointing towards structural differences such as the absence of a M–C2 interaction (Δδ > 56 ppm, Table 1).
Table 1 Comparison of 13C NMR values for metallacyclic units in complexes 2, A, B, and C
|
A
12
|
B
13
|
C
14
|
2
|
[Li2(Me3SiC3SiMe3)]18 |
Tetrameric compound in the solid state, dynamic behaviour in solution leads to two inequivalent 13C shifts.
|
M–C |
220.4 |
257 |
205.3 |
213.8 |
37.8, 44.6a |
C–C–C |
197.1 |
196 |
190.6 |
134.2 |
174.1 |
The molecular structure of compound 2 (Fig. 2) shows the bent metallocene fragment as part of the four-membered ring system. Ti–C bond lengths are significantly longer than typical single bonds (Ti1–C1 2.229(1), Ti1–C3 2.235(2); Σrcov = 2.11 Å
24). That the Ti1–C2 distance (2.178(1) Å) is shorter than Ti1–C1/C3 indicates that the C3 unit is only slightly bent, which is corroborated by the angle C1–C2–C3 of 150.1(2)°. Compared to the Mo and W complexes this C3 unit in 2 is less bent (cf. 130°–135°). The C–C bond distances are in the range of double bonds (C1–C2 1.303(2), C2–C3 1.308(2) Å; Σrcov = 1.34 Å
24) and shorter compared to values found for Mo and W complexes, most likely due to less electron donation to the metal centre. While the Ti1–C1–C2–C3 unit is planar (1.1(3)°) the SiMe3 groups are located above and below this plane, which is in contrast to the structural features reported for group 6 metallacyclobutadienes (cf. p. S15 Table S4,†). This description of a C
C
C unit in 2 is supported by the observed in-phase (1344 cm−1) and out-of-phase (1729 cm−1) vibrations which are in good agreement with calculated values (1343 cm−1/1787 cm−1).22
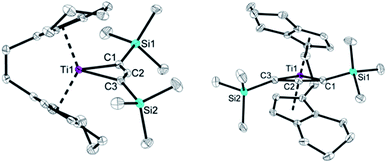 |
| Fig. 2 Two views of the molecular structure of complex 2. Thermal ellipsoids correspond to 30% probability. Hydrogen atoms are omitted for clarity. | |
Analysis of the structure and bonding of 2
To obtain a better understanding of the bonding situation in 1-titanacyclobuta-2,3-diene 2, a series of density functional theory (DFT) and wave function theory (WFT) calculations were performed. Firstly, the structure of 2 was optimised at the BP86
25/LANL2DZ26/TZVP27 level of theory, showing good agreement with structural data from single-crystal X-ray diffraction (cf. p. S48, Table S12†). At this point we noticed a small energy gap between the HOMO and LUMO (1.0 eV), which can be indicative of substantial biradical character. To further evaluate this, the optimised structure was used for several single point calculations employing the pure and hybrid density functionals (DF) BP86 and B3LYP24,28 to calculate the Kohn–Sham (KS) orbitals, as well as HF to compute the canonical MOs. All (KS) wave functions were tested with respect to RHF/UHF or RKS/UKS instabilities, in order to analyse the biradical character of Ti complex 2. While the KS wave function based on the pure DF (BP86) showed no instabilities, the hybrid DF (B3LYP) and HF solution exhibited a low-lying, “broken-symmetry” open-shell singlet state. This kind of behaviour is often observed if the biradical character is not too large,29 since part of the non-dynamic correlation is treated by the exchange–correlation functional of the (pure) density functional. Mixing in exact exchange reduces the amount of correlation treated by the DF and thus the “broken-symmetry” solution becomes more stable. While the structures that were optimised using the BP86 functional show good agreement with experimental structures (Table S12†), the electronic energy should only be considered as a rough approximation due to incorrect treatment of the non-dynamic correlation. Therefore we employed the Complete Active Space (CAS(8,9)) SCF method30 to obtain a multi-determinant open-shell singlet wave function and describe the bonding situation in 2 appropriately. This calculation determined the biradical character of 2 with β = 28%,31,32 and identified the largest contributions to the overall wave function as the two determinants placing two electrons either in the formal HOMO (ϕ4) or LUMO (ϕ5, Fig. 3).
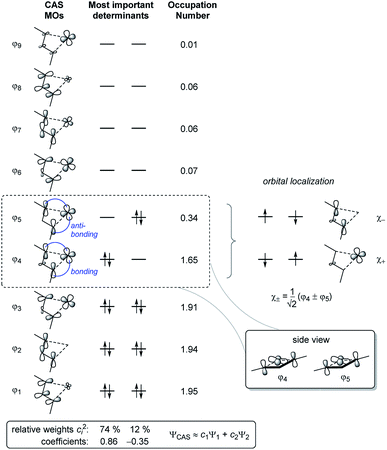 |
| Fig. 3 Schematic depiction of the active orbitals of a CAS(8,9) calculation. Only contributions to the wave function with relative weights > 1% are shown. The orbital localisation scheme indicates that one of the radical centres is localised at Ti, while the other is delocalised across the C3 backbone. | |
The singlet state is calculated to be the ground state (ΔES–T = −9.3 kcal mol−1); i.e. the radical centres are antiferromagnetically coupled and the calculated exchange coupling constant33 is 2J = ES − ET = −3260 cm−1. The radical centres are localised at Ti and on the C3 backbone of the ligand (Fig. 3, right). Therefore, the electronic structure can be understood as a complex possessing a formal Ti(III) fragment and an organic radical, where the “free” electrons are antiferromagnetically coupled. As a consequence, no classical Ti(III) chemistry would be expected as the complex is a singlet. In conjunction with results from Natural Bond Orbital (NBO) analysis, the electronic situation is best described by the main Lewis-type resonance structures depicted on the right side of Scheme 3. Note that the electrons in both the formal πx and πz bonding systems of the formal propadienylide ligand are delocalized across the C3 unit and that each of these π-bonding systems can be interpreted independently of the other, resulting in a variety of different Lewis resonance structures (see also Fig. S50†). The calculated natural charge of the C3(SiMe3)2 ligand amounts to −0.39e (CAS) or −0.64e (BP86), which is in the expected range of a formally anionic ligand. For a more detailed discussion of the CAS wave function and bonding situation, please refer to the ESI, pp. S49.†
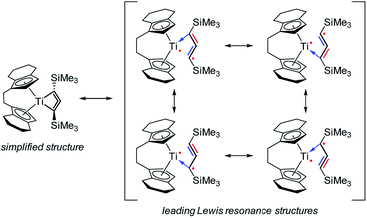 |
| Scheme 3 Main resonance structures which best describe the bonding situation in 2 as singlet biradical. The antiferromagnetically coupled electron at the ligand is delocalised within the out-of-plane πx bonding system (red), while the in-plane πz orbitals (blue) contribute to the C–Ti bonding interactions. | |
In order to investigate the topology of the electron density, we performed a QT-AIM analysis.34,35 This revealed two Ti–C bond paths (Ti1–C1 and Ti1–C3), in agreement with the Lewis resonance scheme (Scheme 3). Despite the short interatomic distance between Ti1 and C2, there is no strong bonding interaction between those atoms; on the contrary, a ring critical point is found near the centre of the TiC3 ring system. Moreover, the Laplacian of the electron density ∇2r indicates that the Ti–C bonds are strongly polarised towards the C atoms (Fig. 4).
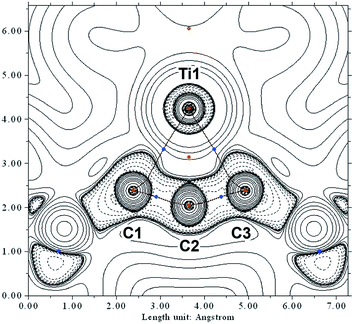 |
| Fig. 4 Contour plot of the Laplacian of the electron density ∇2r of Ti complex 2 in the TiC3 ring plane. Dashed lines indicate negative (local charge concentration), solid lines indicate positive values (local charge depletion). The Laplacian plot is overlaid with the molecular graph from QT-AIM analysis. Brown lines indicate bonding paths, blue dots correspond to bond critical points, orange points indicate ring critical points. Density from CAS(8,9)/def2-TZVP calculation. | |
The densities obtained from CAS(8,9) and BP86 calculations are similar, indicating that the pure DFT method is suitable to approximately describe the electron density despite its single-determinant character (cf. p. S53, Fig. S52†). The results from QT-AIM analysis are corroborated by ELF analysis (cf. p. S53, Fig. S53†). There is no localised electron density in the valence region of C2 directed towards Ti1, whereas the bonding electrons between C1/C3 and Ti are localised in the same area as indicated by the Laplacian of the electron density. Notably, there is no localised electron density around C2 pointing away from Ti1 either, i.e. there is no lone pair of electrons at the central carbon atom. Consequently, the electronic structure of the C3 scaffold is different from that of structurally related bent allenes, such as so-called “carbodicarbenes” (cf. p. S54, Fig. S54†).36
With these results in mind we calculated the biradical character of the hypothetical analogous Cp (β = 30%) and Cp* (β = 74%) substituted complexes and found that the singlet–triplet gap and therefore the biradical character greatly depend on the pyramidalisation (hybridisation) of the carbon atoms C1 and C3 of the TiC3 ring. Since the coordination environment around C1/C3 is nearly planar in
, it shows the highest biradical character. This trend is in agreement with previous computations11 and provides a possible explanation for the selective formation of 1 over a highly reactive planar biradical complex.
Reactions of 2 with carbonyl compounds
To gain first insights into the reactivity of the isolable and unusual biradical [rac-(ebthi)Ti(Me3SiC3SiMe3)] complex we have performed stability tests. Therefore, we exposed solutions of 2 to air and moisture and found that, in both cases, slow formation of the propyne Me3SiC2CH2SiMe3 takes place (see ESI† for details). To evaluate whether our compound shows radical-type reactivity despite its biradical character, we have added 9,10-dihydroanthracene as a potential radical trap, however, we observed no conversion at room temperature. In reactions with TEMPO ((2,2,6,6-tetramethylpiperidin-1-yl)oxyl) formation of ill-defined reaction mixtures that contain Ti(III) species takes place.
We next focused on classical biradical-type reactions that are also known for inorganic cyclic four-membered 1,3-biradical systems.37 In the reaction with CO2, a colour change from red to teal and finally brownish was observed. The NMR spectra of this sample indicate the formation of three so far unidentified organometallic products and thus suggest a high reactivity towards carbonyl substrates. However, these species could not be separated and characterised yet. Based on this observation we have next evaluated the reactivity of 2 towards simple carbonyl compounds benzophenone, acetone, acetophenone, and benzaldehyde (Scheme 4).
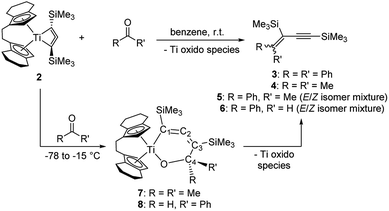 |
| Scheme 4 Reaction of 2 with ketones to yield enynes 3, 4, 5 and 6. | |
NMR analysis of reaction solutions showed that in all cases formation of enynes occurred by formal oxygen transfer to Ti and coupling of the remaining C1 unit with the C3 moiety of the metallacyle. The molecular structure of compound 3 (cf. p. S14, Fig. S7†) confirms the assignment as an enyne.
The nature of the Ti species formed by oxygen transfer could not be unequivocally clarified; however, formation of a Ti oxido species is likely. It should however be noted that such species require either kinetic stabilisation by bulky Cp ligands,38 or additional Lewis bases such as pyridines39 to persist as monomeric well-defined complexes. Monitoring of the reaction of 2 and acetone at low temperature, i.e. slow warming from −78 °C, showed that the red colour of 2 is retained up to a temperature of −15 °C, at which point the solution turned teal. After workup at low temperature, the dark-teal residue was analysed by low-temperature NMR spectroscopy. 13C NMR spectra showed four new resonances due to a new metallacyclic species 7 at 179.7 (C2), 151.9 (C1), 109.4 (C3), and 90.3 ppm (C4), thus suggesting that the well-known insertion of the ketone40 is the first step of C–C bond formation that ultimately yields the enyne (Scheme 4). It should however be noted that this reaction of carbonyl compounds with 2 can also be regarded as an addition reaction to a 1,2-biradical with addition of the C
O bond to both radical centres. Looking at the nature of the reaction products and possible intermediates this cannot be distinguished from classical Ti(IV) diyl insertion reactivity.
Remarkably, in a similar low temperature experiment with 2 and benzaldehyde we detected only the intermediate species 8 which is consistent with a structure of the type 7 (13C NMR of 8: 179.5 (C2), 154.2 (C1), 109.1 (C3), 91.8 ppm (C4)). Thermodynamic calculations reveal intermediate 8 as the sterically less stressed isomer which is preferred by −5.2 kcal mol−1 (cf. p. S42, Fig. S48†).22 This [3 + 1] coupling mode is rather unusual for a 1,3-enyne as these compounds are typically prepared by dimerisation of terminal alkynes,41 or by Pd catalysed Sonogashira coupling reactions.42 Similar carbene transfer reagents include Tebbe's and Petasis' Cp2Ti
CH2 reagent,43 however, in the herein described case transfer of a C3 unit is possible. Moreover, the present reaction pattern is reminiscent of earlier work on retro-cycloaddition using group 4 metallocene oxido or imido complexes.44 It should however be noted that transformation of the herein described reaction into a catalytic protocol might be hampered by the oxophilicity of the Ti centre.
Conclusions
For the first time, we have shown that through combination of suitable metal centre, cyclopentadienyl ligand and substituents at the C3 unit the synthesis of an elusive 1-metallacyclobuta-2,3-diene based on a group 4 metal is possible. Whereas for Cp and Cp* substituted complexes only formation of an unsaturated six-membered chain product was observed, possibly due to coupling of two C3 carbene moieties, in case of rac-(ebthi) formation of the desired 1-titanacyclobuta-2,3-diene was possible. This compound represents a formal metallacyclic analogue of non-existent 1,2-cyclobutadiene. Analysis of the structure and bonding reveals a highly unusual interaction of a formal Ti(III) fragment and an organic monoanionic radical with antiferromagnetic coupling between both radical centres, resulting in a singlet species. Reaction of the metallacycle with carbonyl compounds yields enynes via an unusual [3 + 1] coupling with formal insertion of the C
O bond into the M–C bond being the first step of the reaction. Further studies to understand the structural principles as well as further investigations regarding the reactivity of 2 and related complexes will be done in our lab in the future.
Conflicts of interest
There are no conflicts to declare.
Acknowledgements
We thank our technical and analytical staff for assistance and Prof. Axel Schulz (University of Rostock) for helpful discussions. General support by LIKAT is gratefully acknowledged. We thank the University of Rostock for access to the cluster computer and especially Malte Willert for technical support.
Notes and references
- R. Hoffmann and H. Hopf, Angew. Chem., Int. Ed., 2008, 47, 4474–4481 CrossRef CAS PubMed.
-
(a) R. P. Johnson, Chem. Rev., 1989, 89, 1111–1124 CrossRef CAS;
(b) K. J. Daoust, S. M. Hernandez, K. M. Konrad, I. D. Mackie, J. Winstanley and R. P. Johnson, J. Org. Chem., 2006, 71, 5708–5714 CrossRef CAS PubMed;
(c) M. Melaimi, P. Parameswaran, B. Donnadieu, G. Frenking and G. Bertrand, Angew. Chem., Int. Ed., 2009, 48, 4792–4795 CrossRef CAS PubMed.
- U. Rosenthal, V. V. Burlakov, M. A. Bach and T. Beweries, Chem. Soc. Rev., 2007, 36, 719–728 RSC.
- E. P. Beaumier, A. J. Pearce, X. Y. See and I. A. Tonks, Nat. Rev. Chem., 2019, 3, 15–34 CrossRef PubMed.
-
(a) W. Herrmann, V. P. W. Böhm and C.-P. Reisinger, J. Organomet. Chem., 1999, 576, 23–41 CrossRef CAS;
(b) R. H. Grubbs, Angew. Chem., Int. Ed., 2006, 45, 3760–3765 CrossRef CAS PubMed;
(c) A. K. Tomov, J. J. Chirinos, D. J. Jones, R. J. Long and V. C. Gibson, J. Am. Chem. Soc., 2005, 127, 10166–10167 CrossRef CAS PubMed.
- U. Rosenthal, A. Ohff, W. Baumann, R. Kempe, A. Tillack and V. V. Burlakov, Angew. Chem., Int. Ed. Engl., 1994, 33, 1605–1607 CrossRef.
- N. Suzuki, M. Nishiura and Y. Wakatsuki, Science, 2002, 295, 660–663 CrossRef CAS PubMed.
- J. Ugolotti, G. Dierker, G. Kehr, R. Fröhlich, S. Grimme and G. Erker, Angew. Chem., Int. Ed., 2008, 47, 2622–2625 CrossRef CAS PubMed.
- J. Wei, W.-X. Zhang and Z. Xi, Chem. Sci., 2018, 9, 560–568 RSC.
- T. Beweries, M. Haehnel and U. Rosenthal, Catal. Sci. Technol., 2013, 3, 18–28 RSC.
-
(a) S. Roy, E. D. Jemmis, A. Schulz, T. Beweries and U. Rosenthal, Angew. Chem., Int. Ed., 2012, 51, 5347–5350 CrossRef CAS PubMed;
(b) S. Roy, U. Rosenthal and E. D. Jemmis, Acc. Chem. Res., 2014, 47, 2917–2930 CrossRef CAS PubMed.
- L. G. McCullough, R. R. Schrock, J. C. Dewan and J. C. Murdzek, J. Am. Chem. Soc., 1985, 107, 5987–5998 CrossRef CAS.
-
(a) L. G. McCullough, M. L. Listemann, R. R. Schrock, M. R. Churchill and J. W. Ziller, J. Am. Chem. Soc., 1983, 105, 6729–6730 CrossRef CAS;
(b) M. R. Churchill and J. W. Ziller, J. Organomet. Chem., 1985, 281, 237–248 CrossRef CAS.
-
(a) J. Heppekausen, R. Stade, A. Kondoh, G. Seidel, R. Goddard and A. Fürstner, Chem.–Eur. J., 2012, 18, 10281–10299 CrossRef CAS PubMed;
(b) H. Ehrhorn, D. Bockfeld, M. Freytag, T. Bannenberg, C. E. Kefalidis, L. Maron and M. Tamm, Organometallics, 2019, 38, 1627–1639 CrossRef CAS.
- F. Reiß, K. Altenburger, D. Hollmann, A. Spannenberg, H. Jiao, P. Arndt, U. Rosenthal and T. Beweries, Chem.–Eur. J., 2017, 23, 7891–7895 CrossRef PubMed.
- F. Reiß, M. Reiß, A. Spannenberg, H. Jiao, D. Hollmann, P. Arndt, U. Rosenthal and T. Beweries, Chem.–Eur. J., 2017, 23, 14158–14162 CrossRef PubMed.
- Y. Pang, S. A. Petrich, V. G. Young Jr, M. S. Gordon and T. J. Barton, J. Am. Chem. Soc., 1993, 115, 2534–2536 CrossRef CAS.
- F. Reiß, M. Reiß, A. Spannenberg, H. Jiao, W. Baumann, P. Arndt, U. Rosenthal and T. Beweries, Chem.–Eur. J., 2018, 24, 5667–5674 CrossRef PubMed.
- M. Trose, M. Reiß, F. Reiß, F. Anke, A. Spannenberg, S. Boye, A. Lederer, P. Arndt and T. Beweries, Dalton Trans., 2018, 47, 12858–12862 RSC.
- C. P. Casey, S. Kraft and D. R. Powell, J. Am. Chem. Soc., 2000, 122, 3771–3772 CrossRef CAS.
- T. Shimizu, D. Miyasaka and N. Kamigata, Org. Lett., 2000, 2, 1923–1925 CrossRef CAS PubMed.
- For basic thermochemistry, molecular structures were optimized using the pure density functional (DF) BP86 in combination with the LANL2DZ basis set and corresponding ECP at Ti and the TZVP basis set on all other atoms (notation BP86/LANL2DZ/TZVP) (see ESI† for details).
- Selected examples:
(a) L. Labella, A. Chernega and M. L. H. Green, J. Chem. Soc., Dalton Trans., 1995, 395–402 RSC;
(b) A. Chernega, J. Cook, M. L. H. Green, L. Labella, S. J. Simpson, J. Souter and A. H. H. Stephens, J. Chem. Soc., Dalton Trans., 1997, 3225–3243 RSC;
(c) H. Lee, P. J. Desrosiers, I. Guzei, A. L. Rheingold and G. Parkin, J. Am. Chem. Soc., 1998, 120, 3255–3256 CrossRef CAS;
(d) J. H. Shin and G. Parkin, Chem. Commun., 1999, 887–888 RSC.
- P. Pyykkö and M. Atsumi, Chem.–Eur. J., 2009, 15, 12770–12779 CrossRef PubMed.
-
(a) A. D. Becke, Phys. Rev. A, 1988, 38, 3098–3100 CrossRef CAS;
(b) J. P. Perdew, Phys. Rev. B, 1986, 33, 8822–8824 CrossRef.
-
(a) P. J. Hay and W. R. Wadt, J. Chem. Phys., 1985, 82, 270–283 CrossRef CAS;
(b) P. J. Hay and W. R. Wadt, J. Chem. Phys., 1985, 82, 299–310 CrossRef CAS.
- A. Schäfer, C. Huber and R. Ahlrichs, J. Chem. Phys., 1994, 100, 5829–5835 CrossRef.
-
(a) S. H. Vosko, L. Wilk and M. Nusair, Can. J. Phys., 1980, 58, 1200–1211 CrossRef CAS;
(b) C. Lee, W. Yang and R. G. Parr, Phys. Rev. B: Condens. Matter Mater. Phys., 1988, 37, 785–789 CrossRef CAS;
(c) B. Miehlich, A. Savin, H. Stoll and H. Preuss, Chem. Phys. Lett., 1989, 157, 200–206 CrossRef CAS;
(d) A. D. Becke, J. Chem. Phys., 1993, 98, 5648–5652 CrossRef CAS.
-
(a) C. J. Cramer and B. A. Smith, J. Phys. Chem., 1996, 100, 9664–9670 CrossRef CAS;
(b)
C. J. Cramer, Essentials of Computational Chemistry: Theories and Models, John Wiley & Sons, Ltd, Chichester, UK, 2004 Search PubMed.
-
(a) M. Klene, M. A. Robb, M. J. Frisch and P. Celani, J. Chem. Phys., 2000, 113, 5653–5665 CrossRef CAS;
(b) N. Yamamoto, T. Vreven, M. A. Robb, M. J. Frisch and H. B. Schlegel, Chem. Phys. Lett., 1996, 250, 373–378 CrossRef CAS.
-
(a) L. Salem and C. Rowland, Angew. Chem., Int. Ed. Engl., 1972, 11, 92–111 CrossRef CAS;
(b) E. Miliordos, K. Ruedenberg and S. S. Xantheas, Angew. Chem., Int. Ed., 2013, 52, 5736–5739 CrossRef CAS PubMed.
- Where β = 1 indicates a “perfect” biradical with two electrons in two degenerate orbitals and smaller values indicate an increasing energy gap between HOMO and LUMO, and β → 0 indicates a closed-shell species.
-
(a) L. Noodleman, J. Chem. Phys., 1981, 74, 5737–5743 CrossRef CAS;
(b) D. Herebian, K. E. Wieghardt and F. Neese, J. Am. Chem. Soc., 2003, 125, 10997–11005 CrossRef CAS PubMed.
-
(a) R. F. W. Bader, Acc. Chem. Res., 1985, 18, 9–15 CrossRef CAS;
(b) R. F. W. Bader, Chem. Rev., 1991, 91, 893–928 CrossRef CAS;
(c)
R. F. W. Bader, Atoms in Molecules: A Quantum Theory, Oxford University Press, 1994 Search PubMed;
(d) R. F. W. Bader, Monatsh. Chem., 2005, 136, 819–854 CrossRef CAS.
- T. Lu and F. Chen, Performed and plotted with MultiWfn 3.5, J. Comput. Chem., 2012, 33, 580–592 CrossRef CAS PubMed.
-
(a) R. Tonner and G. Frenking, Angew. Chem., Int. Ed., 2007, 46, 8695–8698 CrossRef CAS PubMed;
(b) C. A. Dyker, V. Lavallo, B. Donnadieu and G. Bertrand, Angew. Chem., Int. Ed., 2008, 47, 3206–3209 CrossRef CAS PubMed.
- A. Hinz, R. Kuzora, A. Schulz and A. Villinger, Chem.–Eur. J., 2014, 20, 14659–16673 CrossRef CAS PubMed.
- T. E. Hanna, E. Lobkovsky and P. J. Chirik, Inorg. Chem., 2007, 46, 2359–2361 CrossRef CAS PubMed.
-
(a) M. R. Smith, P. T. Matsunaga and R. A. Andersen, J. Am. Chem. Soc., 1993, 115, 7049–7050 CrossRef CAS;
(b) C. Godemann, E. Barsch, A. Spannenberg, R. Ludwig and T. Beweries, Eur. J. Inorg. Chem., 2014, 14, 4068–4072 CrossRef.
- Selected examples:
(a) N. Suzuki, R. Kurita, A. Kawamura, T. Ono and Y. Masuyama, J. Organomet. Chem., 2018, 874, 40–48 CrossRef CAS;
(b) K. Altenburger, W. Baumann, A. Spannenberg, P. Arndt and U. Rosenthal, Eur. J. Inorg. Chem., 2014, 14, 5948–5957 CrossRef;
(c) G. Erker, K. Engel, J. L. Atwood and W. E. Hunter, Angew. Chem., Int. Ed., 1983, 22, 494–495 CrossRef.
- R. Chinchilla and C. Nájera, Chem. Rev., 2007, 107, 874–922 CrossRef CAS PubMed.
- H. Doucet and J. C. Hierso, Angew. Chem., Int. Ed., 2007, 46, 834–871 CrossRef CAS PubMed.
-
Modern Carbonyl Olefination – Methods and Applications, ed. T. Takeda, Wiley-VCH, Weinheim, 2004 Search PubMed.
- Selected examples:
(a) T. A. Hanna, A. M. Baranger, P. J. Walsh and R. G. Bergman, J. Am. Chem. Soc., 1995, 117, 3292–3293 CrossRef CAS;
(b) T. A. Hanna, A. M. Baranger and R. G. Bergman, J. Org. Chem., 1996, 61, 4532–4541 CrossRef CAS PubMed;
(c) F. Basuli, H. Aneetha, J. C. Huffman and D. J. Mindiola, J. Am. Chem. Soc., 2005, 127, 17992–17993 CrossRef CAS PubMed;
(d) U. J. Kilgore, F. Basuli, J. C. Huffman and D. J. Mindiola, Inorg. Chem., 2006, 45, 487–489 CrossRef CAS PubMed;
(e) G. D. Kortman, M. J. Orr and K. L. Hull, Organometallics, 2015, 34, 1013–1016 CrossRef CAS;
(f) T. T. Nguyen, G. D. Kortman and K. L. Hull, Organometallics, 2016, 35, 1713–1725 CrossRef CAS.
Footnote |
† Electronic supplementary information (ESI) available: Experimental, spectroscopic, crystallographic and computational details (PDF); xyz coordinates. CCDC 1897219 and 1897220. For ESI and crystallographic data in CIF or other electronic format see DOI: 10.1039/c9sc01002e |
|
This journal is © The Royal Society of Chemistry 2019 |
Click here to see how this site uses Cookies. View our privacy policy here.