DOI:
10.1039/C9SC00028C
(Edge Article)
Chem. Sci., 2019,
10, 3963-3968
A2SrMIVS4 (A = Li, Na; MIV = Ge, Sn) concurrently exhibiting wide bandgaps and good nonlinear optical responses as new potential infrared nonlinear optical materials†
Received
3rd January 2019
, Accepted 23rd February 2019
First published on 25th February 2019
Abstract
Exploration of new nonlinear optical (NLO) materials is of importance for infrared (IR) applications. However, it is an extremely tough challenge to design and synthesize excellent IR NLO materials with optimal performances (e.g., concurrently a large NLO response and wide bandgap). Herein, four new mixed alkali/alkaline earth metal sulfides, A2SrMIVS4 (A = Li, Na; MIV = Ge, Sn), were successfully synthesized by a motif-optimization approach using the classical AgGaS2 as a template. Note that all of them concurrently exhibit wide bandgaps (3.1–3.8 eV) and good NLO responses (0.5–0.8 × AgGaS2) with phase-matching behavior, which satisfy the balance conditions (Eg ≥ 3.0 eV and dij ≥ 0.5 × benchmark AgGaS2) of optical performances and hence are outstanding IR NLO materials. Remarkably, both of Na2SrMIVS4 have the same structure without the structural transformation (Ge to Sn) in the reported related analogues and an interesting cation-dependent structural change is also found in Na2MIISnS4 (MII: Sr, R3c vs. Ba, I
2d). These results verify that the above design strategy of motif-optimization provides a feasible guide for the discovery of new IR NLO candidates and the A–AE–M–S (A = alkali metal; AE = alkaline-earth metal; M = Ga, In, Ge, Sn) system was identified as the preferred system for IR NLO materials.
Introduction
Nonlinear optical (NLO) materials are of great importance in achieving the output of tunable lasers ranging from ultraviolet (UV)/deep-UV to the infrared (IR) region.1 In the deep-UV to visible regions, many kinds of oxides including borates,2 phosphates,3 carbonates,4 and iodates5 have been developed as worth exploring systems for the discovery of NLO candidates. Unfortunately, most oxides are limited in the IR region because of their low IR absorption edges induced by the strong absorption of metal/metalloid-oxygen bands. So far, commercially available NLO materials have been rarely discovered in the IR region and all of them still exhibit some inherent performance drawbacks (low laser damage threshold (LDT) and two-photon absorption (TPA)) that seriously hinder their wide applications.6 Therefore, a critical prerequisite for the future exploration of IR NLO materials is to achieve a suitable balance between the large second harmonic generation (SHG) response (dij) and wide bandgap (Eg) in view of their inversely proportional relationship; thus, the two important parameters (Eg and dij) must satisfy the following balance conditions (Eg ≥ 3.0 eV and dij ≥ 0.5 × benchmark AgGaS2) for one outstanding IR NLO material.7 After decades of exploration and development, hundreds of IR NLO materials have been synthesized and note that chalcogenides are the main research source for the exploration of new IR NLO materials.8 The previously reported results show that by incorporation of the second-order Jahn–Teller (SOJT) effect d0 transition-metals or/and lone-pair cations into crystal structures it is hard to obtain suitable balanced performances for IR NLO materials.9 Thus, an efficient design strategy that could enhance the Eg and simultaneously maintain good dij appears to be particularly important. After a detailed literature survey, to the best of our knowledge, about dozens of chalcogenides satisfy the demand of performance balance so far and most of them are sulfides.10 Besides, this investigation also shows that almost all their cations are alkali or/and alkaline earth metals without d–d or f–f electronic transitions and several anionic groups, e.g., typical MS4 (M = Ga, In, Ge, Sn) tetrahedra, act as critical “NLO active units” and provide the main contribution to the origin of the SHG response.11 Recently, considering the structural and performance features of commercial IR NLO materials, a targeted element-cosubstitution strategy has been used to design new potential IR NLO materials by the replacement of cations or/and anionic groups with classical materials as templates.12 For example, many diamond-like (DL) and chalcopyrite-like (CL) sulfides can be structurally designed from the prototype compound AgGaS2.13 With this in mind, the classical AgGaS2 is chosen as a template, through substituting the Ag cation with alkali (Li, Na) and alkaline earth (Sr) metals and the GaS4 anionic group with MIVS4 (MIV = Ge, Sn) NLO active units to afford the successful discovery of four new noncentrosymmetric (NCS) sulfides in this work, A2SrMIVS4 (A = Li, Na; MIV = Ge, Sn). The experimental results show that all of them exhibit excellent performances (Eg: 3.1–3.8 eV; dij: 0.5–0.8 × AgGaS2) and achieve the optimal balance of wide Eg and good dij, which indicates that they satisfy the performance demands as outstanding IR NLO candidates and can further remove the low LDTs and TPA of commercial materials. Moreover, the above results also confirm the feasibility of the motif-optimization strategy for exploring new IR NLO materials.
Results and discussion
Single crystal X-ray analysis shows that Li2SrMIVS4 crystallize in the I
2m space group whereas Na2SrMIVS4 crystallize in the R3c space group (Table S1†). Note that an interesting structural change is found in Na2SrSnS4 (R3c) and Na2BaSnS4 (I
2d), but Na2SrMIVS4 do not undergo the structural transformation with different MIV (Ge to Sn) atoms in comparison with other reported analogues, such as Ag2BaGeS4 (I
2m) vs. Ag2BaSnS4 (I222)14 and Na2BaGeS4 (R3c) vs. Na2BaSnS4 (I
2d)12a (Table S2†). Bond valences and the global instability index (GII) are also calculated and the results verify their structural reasonability (GII = 0.10–0.13) (Tables S3–S6†). Herein, we have chosen Li2SrGeS4 and Na2SrSnS4 as the representatives to depict their structural differences. As for Li2SrGeS4, its three-dimensional (3D) network structure is composed of 2D corner-sharing (LiS4)n layers (Fig. 1a) and isolated GeS4 ligands, where the Sr atoms are located in the tunnels (Fig. 1b); in other words, its structure can be also depicted as a 3D framework composed by the interconnection of edge-sharing SrS8 dodecahedra with Li and Ge atoms located at the channels (Fig. 1c). More importantly, it appears that the interesting compression of the unit cell along the c-axis and Li2SrGeS4 can be considered as the “compressed CL material”, which is also similar to the reported CL structures. According to the following equation of the structural distortion degree (Δd = 2 − (c/a), a and c are the cell parameters),15 the calculated results show that Li2SrMIVS4 exhibit a large Δd (Ge: 0.815 vs. Sn: 0.811), thus leading to severe compression along the c axis because of the larger coordination number (8) of Sr atoms than only 4 in classical AgGaS2 (Table S7†). Moreover, based on the similar structures of known Li-based compressed CL sulfides,13b the interlayer spacing between two adjacent (LiS4)n layers (1 and 2) was also calculated and the interlayer spacing increases gradually from 2.5160 to 2.5497 Å with the increase of the cation radius of Sr2+ (1.40 Å) to Ba2+ (1.56 Å),16 which indicates that a large cation radius corresponds to a wide interlayer spacing but is inversely proportional to the large Δd in compressed CL materials (Fig. 1d and Table S7†). This result may give us a feasible way to predict the interlayer spacing and Δd for new compressed CL materials.
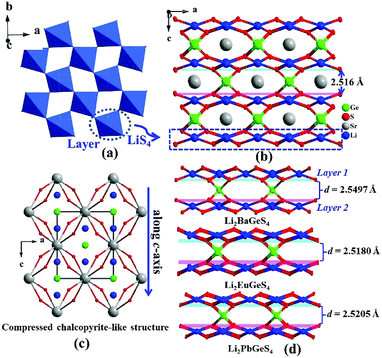 |
| Fig. 1 (a) Corner-sharing (LiS4)n layer; (b) whole crystal structure of Li2SrGeS4 (Sr–S bonds omitted for clarity); (c) 3D network structure composed of edge-sharing SrS8 dodecahedra (Ge–S and Li–S bonds omitted for clarity); (d) comparison of the interlayer spacing between adjacent (LiS4)n layers in Li-based materials. | |
Na2MIISnS4 (MII = Sr, Ba) undergo the interesting structural transformation (Sr, R3c vs. Ba, I
2d) with the different alkaline-earth metals (Fig. 2). Based on the previous analysis,12a the whole structure of Na2SrSnS4 can be depicted as the combination of numerous three-types of rings including the outermost 24-NaS6-MR ring, inner fake ring (composed of six isolated SnS4 tetrahedra) and innermost 6-(SrS7)-MR circle (Fig. 2b). Interestingly, both of the similar isolated SnS4 tetrahedron and NaS6 octahedron exist in the structures of Na2MIISnS4 although they still have several obviously different structural features. The prominent structural difference is the connection modes of NaS6 and BaS8/SrS7 polyhedra, for instance, the BaS8 polyhedra connect with each other by sharing edges to form a 3D network in Na2BaSnS4 (Fig. 2a), whereas the SrS7 ligands link together by sharing corners and edges to make up the tunnel structure in Na2SrSnS4; besides, note that the NaS6 octahedra are connected together to form 24-MRs in Na2SrSnS4, which is obviously different from that (all Na atoms exist in the tunnels linking together to form a 3D framework structure) in Na2BaSnS4. Moreover, Na2SrMS4 show discriminated structures compared with those of Li2SrMS4 (R3c vs. I
2m) because of the different coordination numbers for monovalent cations (Na: 6 vs. Li: 4). We have also investigated the effect of distortion degrees (Δd = (d(longest) − d(shortest)) and Δθ = (θ(largest) − θ(smallest))) of IS4 tetrahedra (I =Ag, Cu, Na, Li) on the structural changes of the I2–AE–M–S4 system (Table S2†). The result shows that the distortion degrees (Δd and Δθ) of IS4 units exhibit significant changes while the series of compounds undergo the space group transformations. After analysis of their structural features, it is clear that their structural transformations have a close relationship with the sizes and coordination numbers of cations. The above results also verify that the variable structural dimensionality and symmetry can be attributed to the cationic size effect, and similar phenomena were discovered in many relative systems, such as BaGa4S7 (Pmn21)11avs. PbGa4S7 (Pc),17a Ba2GeSe4 (P21/m)17bvs. Mg2GeSe4 (Pnma),17cetc. More importantly, our previous work has also reported the rising structural symmetries (Cs, P
; Rb, P21/c; α-K, Aba2; and Na, P
c2) in the A2Hg3Ge2S8 (A = alkali metal) system with the element substitutions (Cs to Na).18 Thus, a slight change of the cation size can lead to structural changes and future structure prediction should pay close attention to the effect of cation sizes on crystal structures.
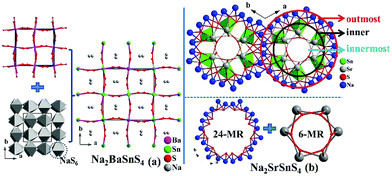 |
| Fig. 2 (a) Crystal structure of Na2BaSnS4 along the c-axis: a 3D network composed of edge-sharing BaS8 dodecahedra, and an edge and corner-sharing (NaS6)n 3D network; (b) the whole structure of Na2SrSnS4: three-types of rings (outermost, inner, innermost), 24-NaS6-MR, and 6-SrS7-MR. | |
Air-stable high quality single crystals of millimeter dimension for title compounds were successfully prepared by spontaneous crystallization in vacuum-sealed silica tubes (Fig. 4a and b). Their experimental XRD patterns are in agreement with the simulated ones derived from the crystal data, which further verify the purity of polycrystalline samples (Fig. 3). We have also compared the powder XRD patterns before and after melting for the title compounds. Comparison results show that all of them exhibit identical patterns before and after melting, which demonstrates that they are congruently melting compounds (Fig. S1†). Besides, as seen from Fig. S2,† there are no obvious absorption peaks in the range from 4000 to 500 cm−1, which indicates that they have a wide IR transmission region (∼20 μm). In addition, critical optical parameters including Eg, dij and LDTs of the title compounds were systematically measured. All of them exhibit a wider Eg than the benchmark AgGaS2 (2.73 eV), such as Li2SrGeS4 (3.75 eV), Li2SrSnS4 (3.10 eV), Na2SrGeS4 (3.80 eV), and Na2SrSnS4 (3.12 eV), which is similar to those of other reported A2BaMS4 compounds (Fig. 4a and b). The above results also indicate that the cosubstitution of alkali or alkaline earth metals in the same main group has little influence on the material Eg, which can also be confirmed in relative systems including AZn4Ga5S12 (A = K, Rb, Cs; ∼3.65 eV)19 and A3Ga3PS8Cl (A = K, Rb; 3.60 vs. 3.65 eV).10d Besides, their shortwave absorption edges are lower than 400 nm and such a low absorption edge can remove the effect of harmful TPA at 1 μm for another classical ZnGeP2; thus, recent mature laser sources (e.g. 1.06 μm) may be utilized to achieve the frequency-conversion in the title compounds. Besides, based on density functional theory, their band structures have been calculated and the results indicate that their optical absorptions (2.20–2.84 eV) are mainly derived from the MIVS4 units for the title compounds (Fig. 5 and S3†). Moreover, a wide Eg is helpful to improve the LDT and further ensures the normal use without damage or breakdown under the high-power laser system for one of the materials. Recently, estimating the LDTs of new NLO compounds on powder samples has become a feasible method according to the previously reported research results.20 Herein, powder LDTs of the title compounds were investigated under the excitation of a 1.06 μm pulse laser with AgGaS2 as the reference (Table S8†). The experimental result shows that the title compounds have higher LDTs than AgGaS2, for example, Li2SrGeS4 and Na2SrGeS4 (10 × AgGaS2), Li2SrSnS4 (5.0 × AgGaS2), and Na2SrSnS4 (6.0 × AgGaS2), which are comparable to those of other famous IR NLO crystals, such as LiGaS2 (11 × AgGaS2),21 LiInS2 (2.5 × AgGaS2),21 HgGa2S4 (3.0 × AgGaS2),22 BaGa4S7 (3.0 × AgGaS2),11a and BaGa4Se7 (3.7 × AgGaS2).11b Based on the high LDTs and low shortwave absorption edges, the title compounds have good potential to achieve the output of the high-power IR laser with mature lasers (Ti:Al2O3 or Nd:YAG) excitation sources in comparison with commercial IR NLO materials.
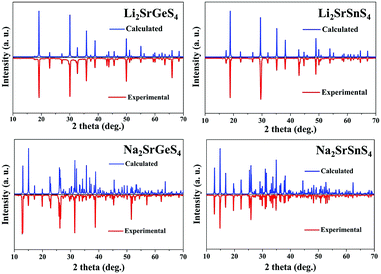 |
| Fig. 3 Powder XRD patterns of title compounds. | |
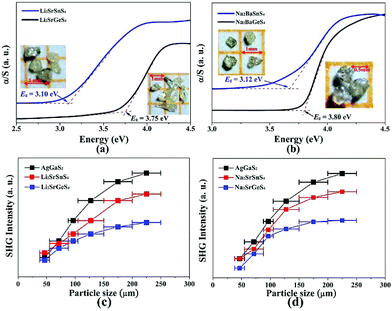 |
| Fig. 4 (a) Experimental bandgaps of Li2SrGeS4 and Li2SrSnS4 (inserted are the photographs of picked crystals); (b) experimental bandgaps of Na2SrGeS4 and Na2SrSnS4 (inserted are the photographs of picked crystals); (c) powder SHG responses of Li2SrGeS4 and Li2SrSnS4versus AgGaS2; (d) powder SHG responses of Na2SrGeS4 and Na2SrSnS4versus AgGaS2. | |
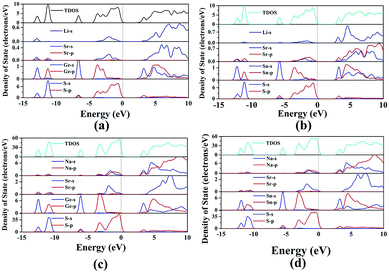 |
| Fig. 5 Total and partial density of states (TDOS and PDOS) of title compounds: (a) Li2SrGeS4, (b) Li2SrSnS4, (c) Na2SrGeS4, and (d) Na2SrSnS4. | |
In view of the NCS structures of the title compounds, the common Kurtz and Perry method23 was adopted to investigate their powder SHG responses with AgGaS2 as the reference. As seen from Fig. 4, all of their SHG intensities show a gradually increasing trend with the increase of their particle sizes, respectively, indicating the type I phase-matching behavior that is of importance for the high-efficient laser output, which is also similar to those of relative Ba-based analogues. Note that they exhibit good SHG responses about 0.5 and 0.8 times that of AgGaS2 (d36 = 13.9 pm V−1) for the title thiogermanates (7.0 pm V−1) and thiostannates (11.1 pm V−1) at the maximum particle size (200–250 μm), respectively. Meanwhile, their SHG coefficients have also been calculated and the maximum values are 4.75 for Li2SrGeS4, 6.64 for Li2SrSnS4, 6.44 for Na2SrGeS4 and 7.07 pm V−1 for Na2SrSnS4, respectively, which are basically in agreement with the experimental results. Besides, their dij is comparable to those of famous Li-based IR NLO materials, such as LiGaS2 (d31 = 5.8 pm V−1)21 or LiInS2 (d31 = 7.25 pm V−1).21 According to the above analysis, the title compounds exhibit excellent performances with a wide Eg (>3.0 eV) and good dij (>0.5 × AgGaS2) that satisfy the proposed balance conditions and hence are outstanding IR NLO materials. Thus, all of them can be expected to act as promising NLO candidates in the IR region.
Recently, an increasing number of IR NLO materials has been discovered by adopting the motif-optimization strategy with classical materials as templates based on the optimized atoms or/and anionic groups, such as DL or CL materials. Thus, this strategy has been proved to be an effective way to reorganize crystal structures and adjust physicochemical performances.13 In this work, incorporation of electropositive alkali or/and alkaline earth metals (A+/AE2+) as cations and MIVS4 as “NLO active units” into the crystal structure of AgGaS2 to replace the Ag+ cation and GaS4 units affords the successful discovery of a series of promising IR NLO materials and the optimization of alkali or alkaline earth metals in the same main group has little influence on the material Eg, which can be also confirmed in the successful discovery of a series of promising IR NLO materials and the research results also prove that the above design strategy is feasible (Fig. 6). Up to now, only 8 related IR NLO materials with excellent performances belonging to the A2AEMIVS4 (A = Li, Na; AE = Ba, Sr; MIV = Ge, Sn) formula have been discovered (Table S9†). Thus, based on the above analysis, it can be concluded that the selection of cations or anionic groups should be extended to other A (K, Rb, Cs) and AE (Mg, Ca) elements or MIIIS4 ligands and the A–AE–M–S system should be identified as the preferred system for the future exploration of new IR NLO materials.
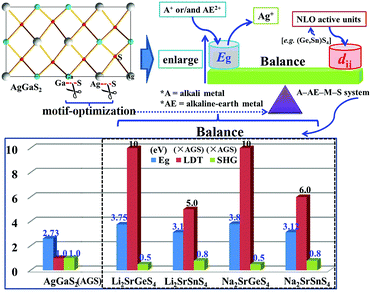 |
| Fig. 6 Schematic diagram of designing new IR NLO materials by the motif-optimization with AgGaS2 as the template and title compounds achieve the suitable balance of wide Eg and good dij. | |
Conclusions
In summary, four potential IR NLO sulfides, A2SrMIVS4, have been successfully discovered by the motif-optimization design strategy with classical AgGaS2 as the template. Li2SrMIVS4 exhibit compressed CL structures, whereas Na2SrMIVS4 possess multiple rings and tunnel structures. Besides, analysis of the interlayer spacing (d) among CL materials shows that d has a close relationship with the cation radius and structural distortion degrees. Remarkably, all of them exhibit excellent performances, especially for the optimal balance of wide Eg and good dij. The discovery of the above materials also confirms that motif-optimization is an effective strategy to tune crystal structures and performances and then further design promising IR NLO candidates. Furthermore, considering the characteristics of optimized cations or anionic groups, the A–AE–M–S system was identified as the preferred system for the future exploration of new IR NLO materials.
Conflicts of interest
There are no conflicts to declare.
Acknowledgements
This work was supported by the National Natural Science Foundation of China (Grant No. 51872324, 51425206, 61835014), Advanced Talents Incubation Program of Hebei University (801260201293), National Key Research Project (Grant No. 2016YFB1102302, 2016YFB0402104), Xinjiang Key Research and Development Program (Grant No. 2016B02021), Major Program of Xinjiang Uygur Autonomous Region of China during the 13th Five-Year Plan Period (Grant No. 2016A02003) and Foundation of Director of XTIPC, CAS (Grant No. 2016PY004).
Notes and references
-
(a) S. F. Li, X. M. Jiang, Y. H. Fan, B. W. Liu, H. Y. Zeng and G. C. Guo, Chem. Sci., 2018, 9, 5700 RSC;
(b) T. T. Tran, H. W. Yu, J. M. Rondinelli, K. R. Poeppelmeier and P. S. Halasyamani, Chem. Mater., 2016, 28, 5238 CrossRef CAS;
(c) Y. Wang, B. B. Zhang, Z. H. Yang and S. L. Pan, Angew. Chem., Int. Ed., 2018, 57, 2150 CrossRef CAS PubMed;
(d) Z. G. Xia and K. R. Poeppelmeier, Acc. Chem. Res., 2017, 50, 1222 CrossRef CAS PubMed;
(e) P. Becker, Adv. Mater., 1998, 10, 979 CrossRef CAS;
(f) K. M. Ok, Acc. Chem. Res., 2016, 49, 2774 CrossRef CAS PubMed;
(g) Y. Pan, S. P. Guo, B. W. Liu, H. G. Xue and G. C. Guo, Coord. Chem. Rev., 2018, 374, 464 CrossRef CAS;
(h) X. F. Wang, Y. Wang, B. B. Zhang, F. F. Zhang, Z. H. Yang and S. L. Pan, Angew. Chem., Int. Ed., 2017, 56, 14119 CrossRef CAS PubMed.
-
(a) R. L. Tang, C. L. Hu, F. F. Mao, J. H. Feng and J. G. Mao, Chem. Sci., 2019, 10, 837 RSC;
(b) G. Q. Shi, Y. Wang, F. F. Zhang, B. B. Zhang, Z. H. Yang, X. L. Hou, S. L. Pan and K. R. Poeppelmeier, J. Am. Chem. Soc., 2017, 139, 10645 CrossRef CAS PubMed;
(c) F. Kong, S. P. Huang, Z. M. Sun, J. G. Mao and W. D. Cheng, J. Am. Chem. Soc., 2006, 128, 7750 CrossRef CAS PubMed;
(d) S. G. Zhao, P. F. Gong, S. Y. Luo, S. J. Liu, L. N. Li, M. A. Asghar, T. Khan, M. C. Hong, Z. S. Lin and J. H. Luo, J. Am. Chem. Soc., 2015, 137, 2207 CrossRef CAS PubMed;
(e) H. P. Wu, S. L. Pan, K. R. Poeppelmeier, H. Y. Li, D. Z. Jia, Z. H. Chen, X. Y. Fan and Y. Yang, J. Am. Chem. Soc., 2011, 133, 7786 CrossRef CAS PubMed.
-
(a) X. S. Xing, R. J. Sa, P. X. Li, N. N. Zhang, Z. Y. Zhou, B. W. Liu, J. Liu, M. S. Wang and G. C. Guo, Chem. Sci., 2017, 8, 7751 RSC;
(b) H. W. Yu, W. G. Zhang, J. Young, J. M. Rondinelli and P. S. Halasyamani, J. Am. Chem. Soc., 2016, 138, 88 CrossRef CAS PubMed;
(c) B. B. Zhang, G. P. Han, Y. Wang, X. L. Chen, Z. H. Yang and S. L. Pan, Chem. Mater., 2018, 30, 5397 CrossRef CAS;
(d) L. Xiong, J. Chen, J. Lu, C. Y. Pan and L. M. Wu, Chem. Mater., 2018, 30, 7823 CrossRef CAS.
-
(a) G. H. Zou, Z. E. Lin, H. M. Zeng, H. Jo, S. J. Lim, T. S. You and K. M. Ok, Chem. Sci., 2018, 9, 8957 RSC;
(b) G. H. Zou, N. Ye, L. Huang and X. S. Lin, J. Am. Chem. Soc., 2011, 133, 20001 CrossRef CAS PubMed;
(c) G. H. Zou, H. Jo, S. J. Lim, T. S. You and K. M. Ok, Angew. Chem., Int. Ed., 2018, 57, 8619 CrossRef CAS PubMed;
(d) J. Chen, L. Xiong, L. Chen and L. M. Wu, J. Am. Chem. Soc., 2018, 140, 14082 CrossRef CAS PubMed.
-
(a) C. L. Hu and J. G. Mao, Coord. Chem. Rev., 2015, 288, 1 CrossRef CAS;
(b) M. Mutailipu, M. Zhang, H. P. Wu, Z. H. Yang, Y. H. Shen, J. L. Sun and S. L. Pan, Nat. Commun., 2018, 9, 3089 CrossRef PubMed.
-
(a) A. O. Okorogu, S. B. Mirov, W. Lee, D. I. Crouthamel, N. Jenkins, A. Y. Dergachev, K. L. Vodopyanov and V. V. Badikov, Opt. Commun., 1998, 155, 307 CrossRef CAS;
(b) G. D. Boyd, F. G. Storz, J. H. McFee and H. M. Kasper, IEEE J. Quantum Electron., 1972, 8, 900 CrossRef CAS;
(c) G. D. Boyd, E. Buehler and F. G. Storz, Appl. Phys. Lett., 1971, 18, 301 CrossRef CAS.
-
(a) L. Kang, M. L. Zhou, J. Y. Yao, Z. S. Lin, Y. C. Wu and C. T. Chen, J. Am. Chem. Soc., 2015, 137, 13049 CrossRef CAS PubMed;
(b) F. Liang, L. Kang, Z. S. Lin and Y. C. Wu, Cryst. Growth Des., 2017, 17, 2254 CrossRef CAS.
-
(a) I. Chung and M. G. Kanatzidis, Chem. Mater., 2014, 26, 849 CrossRef CAS;
(b) S. P. Guo, Y. Chi and G. C. Guo, Coord. Chem. Rev., 2017, 335, 44 CrossRef CAS;
(c) M. C. Chen, L. M. Wu, H. Lin, L. J. Zhou and L. Chen, J. Am. Chem. Soc., 2012, 134, 6058 CrossRef CAS PubMed;
(d) Z. Z. Luo, C. S. Lin, H. H. Cui, W. L. Zhang, H. Zhang, H. Chen, Z. Z. He and W. D. Cheng, Chem. Mater., 2015, 27, 914 CrossRef CAS;
(e) X. M. Jiang, S. P. Guo, H. Y. Zeng, M. J. Zhang and G. C. Guo, Struct. Bonding, 2012, 145, 1 CrossRef CAS.
-
(a) T. K. Bera, J. I. Jang, J. B. Ketterson and M. G. Kanatzidis, J. Am. Chem. Soc., 2009, 131, 75 CrossRef CAS PubMed;
(b) J. C. Syrigos, D. J. Clark, F. O. Saouma, S. M. Clarke, L. Fang, J. I. Jang and M. G. Kanatzidis, Chem. Mater., 2015, 27, 255 CrossRef CAS.
-
(a) B. W. Liu, H. Y. Zeng, X. M. Jiang, G. E. Wang, S. F. Li, L. Xu and G. C. Guo, Chem. Sci., 2016, 7, 6273 RSC;
(b) Y. Y. Li, P. F. Liu and L. M. Wu, Chem. Mater., 2017, 29, 5259 CrossRef CAS;
(c) J. A. Brant, D. J. Clark, Y. S. Kim, J. I. Jang, J. H. Zhang and J. A. Aitken, Chem. Mater., 2014, 26, 3045 CrossRef CAS;
(d) J. A. Brant, D. J. Clark, Y. S. Kim, J. I. Jang, A. Weiland and J. A. Aitken, Inorg. Chem., 2015, 4, 2809 CrossRef PubMed;
(e) K. Wu and S. L. Pan, Coord. Chem. Rev., 2018, 377, 191 CrossRef CAS.
-
(a) X. S. Lin, G. Zhang and N. Ye, Cryst. Growth Des., 2009, 9, 1186 CrossRef CAS;
(b) J. Y. Yao, D. J. Mei, L. Bai, Z. S. Lin, W. L. Yin, P. Z. Fu and Y. C. Wu, Inorg. Chem., 2010, 49, 9212 CrossRef CAS;
(c) Y. Kim, I. S. Seo, S. W. Martin, J. Baek, P. S. Halasyamani, N. Arumugam and H. Steinfink, Chem. Mater., 2008, 20, 6048 CrossRef CAS;
(d) B. W. Liu, H. Y. Zeng, M. J. Zhang, Y. H. Fan, G. C. Guo, J. S. Huang and Z. C. Dong, Inorg. Chem., 2015, 54, 976 CrossRef CAS PubMed.
-
(a) K. Wu, Z. H. Yang and S. L. Pan, Angew. Chem., Int. Ed., 2016, 55, 6713 CrossRef CAS PubMed;
(b) D. J. Mei, S. Y. Zhang, F. Liang, S. G. Zhao, J. Q. Jiang, J. B. Zhong, Z. S. Lin and Y. C. Wu, Inorg. Chem., 2017, 56, 13267 CrossRef CAS PubMed.
-
(a) F. Liang, L. Kang, Z. S. Lin, Y. C. Wu and C. T. Chen, Coord. Chem. Rev., 2017, 333, 57 CrossRef CAS;
(b) K. Wu, B. B. Zhang, Z. H. Yang and S. L. Pan, J. Am. Chem. Soc., 2017, 139, 14885 CrossRef CAS PubMed;
(c) H. Chen, P. F. Liu, B. X. Li, H. Lin, L. M. Wu and X. T. Wu, Dalton Trans., 2018, 47, 429 RSC.
-
(a) C. L. Teske, Z. Naturforsch., 1979, 34b, 544 CAS;
(b) L. Y. Nian, K. Wu, G. J. He, Z. H. Yang and S. L. Pan, Inorg. Chem., 2018, 57, 3434 CrossRef CAS PubMed.
- J. A. Aitken, P. Larson, S. D. Mahanti and M. G. Kanatzidis, Chem. Mater., 2001, 13, 4714 CrossRef CAS.
- F. C. Hawthorne, Acta Crystallogr., 1994, B50, 481 CAS.
-
(a) X. S. Li, L. Kang, C. Li, Z. S. Lin, J. Y. Yao and Y. C. Wu, J. Mater. Chem. C, 2015, 3, 3060 RSC;
(b) A. Assoud, N. Soheilnia and H. Kleinke, Z. Naturforsch., 2004, 59, 975 CAS;
(c) K. Wu, S. L. Pan and Z. H. Yang, RSC Adv., 2015, 5, 33646 RSC.
-
(a) J. H. Liao, G. M. Marking, K. F. Hsu, Y. Matsushita, M. D. Ewbank, R. Borwick, P. Cunningham, M. J. Rosker and M. G. Kanatzidis, J. Am. Chem. Soc., 2003, 125, 9484 CrossRef CAS PubMed;
(b) G. A. Marking, J. A. Hanko and M. G. Kanatzidis, Chem. Mater., 1998, 10, 1191 CrossRef CAS;
(c) K. Wu, Z. H. Yang and S. L. Pan, Chem. Mater., 2016, 28, 2795 CrossRef CAS.
- H. Lin, H. Chen, Y. J. Zheng, J. S. Yu, X. T. Wu and L. M. Wu, Chem.–Eur. J., 2017, 23, 10407 CrossRef CAS PubMed.
-
(a) M. J. Zhang, X. M. Jiang, L. J. Zhou and G. C. Guo, J. Mater. Chem. C, 2013, 1, 4754 RSC;
(b) M. J. Zhang, B. X. Li, B. W. Liu, Y. H. Fan, X. G. Li, H. Y. Zeng and G. C. Guo, Dalton Trans., 2013, 42, 14223 RSC.
-
(a) L. Isaenko, A. Yelisseyev, S. Lobanov, A. Titov, V. Petrov, J. J. Zondy, P. Krinitsin, A. Merkulov, V. Vedenyapin and J. Smirnova, Cryst. Res. Technol., 2003, 38, 379 CrossRef CAS;
(b) L. Isaenko and I. Vasilyeva, J. Cryst. Growth, 2008, 310, 1954 CrossRef CAS.
- V. Petrov, G. Marchev, A. Tyazhev, M. Beutler, V. Panyutin, M. Starikova, A. Esteban-Martin, V. Badikov, G. Shevyrdyaeva, D. Badikov, M. Reza, S. Sheina and A. Fintisova, Opt. Eng., 2013, 52, 117102 CrossRef.
- S. K. Kurtz and T. T. Perry, J. Appl. Phys., 1968, 39, 3798 CrossRef CAS.
Footnotes |
† Electronic supplementary information (ESI) available: Experimental details, structural distortion, BVS and GII, performance comparison, LDTs, powder XRD, BS and DOS. CCDC 1888059, 1888058, 1888057, and 1888060 for Li2SrGeS4, Li2SrSnS4, Na2SrGeS4, and Na2SrSnS4, respectively. For ESI and crystallographic data in CIF or other electronic format see DOI: 10.1039/c9sc00028c |
‡ Kui Wu and Yu Chu contributed equally. |
|
This journal is © The Royal Society of Chemistry 2019 |
Click here to see how this site uses Cookies. View our privacy policy here.