DOI:
10.1039/C8SC05714A
(Edge Article)
Chem. Sci., 2019,
10, 4220-4226
Dinuclear HgII tetracarbene complex-triggered aggregation-induced emission for rapid and selective sensing of Hg2+ and organomercury species†‡
Received
21st December 2018
, Accepted 5th March 2019
First published on 6th March 2019
Abstract
Rapid, reliable and highly selective detection of mercury species, including Hg2+ ions and organomercury, is of significant importance for environmental protection and human health. Herein, a new fluorescent dye 1,1,2,2-tetrakis[4-(3-methyl-1H-benzimidazol-1-yl)phenyl ethylene tetraiodide (Tmbipe) with aggregation-induced emission (AIE) potential was prepared and characterized. The presence of four positively charged methylated benzimidazole groups endows Tmbipe with excellent water solubility and almost undetectable background fluorescence. However, it can coordinate with two Hg2+ ions or two organomercury molecules (e.g. methylmercury and phenylmercury) to form a planar dinuclear HgII tetracarbene complex, which can then self-aggregate to turn on AIE fluorescence. Such a fluorescence turn-on process can be completed in 3 min. In addition, synergic rigidification of the tetraphenylethylene-bridged Tmbipe molecule by mercury-mediated chelate ring formation and subsequent aggregation results in obviously higher fluorescence enhancement than that given by the single aggregation-induced one. Low background, high fluorescence enhancement and rapid response time make Tmbipe a good fluorescent probe for reliable, sensitive and highly selective quantitation of both inorganic and organic mercury species. This probe was also demonstrated to work well for identification of mercury species accumulation in living cells.
Introduction
Mercury is generally acknowledged as one of the most toxic and dangerous metal ions because of its well-characterized toxicological features.1 Even at low concentrations, mercury is highly toxic. Mercury can spread through air, water and oil. And what's worse, it can accumulate through the food chain and atmospheric circulation.2 Exposure to mercury can cause serious environmental and health threats. It has been found that mercury damages the kidneys, especially renal proximal tubular epithelial cells. Mercury can also cause immune dysfunction, nephrotic syndrome and glomerulonephritis. In addition, it can harm the brain, nerves and endocrine system.3
Great efforts have been made in the sensitive and selective detection of mercury. High performance liquid chromatography (HPLC),4 gas chromatography (GC),5 atomic absorption spectroscopy (AAS),6 capillary electrophoresis,7 inductively coupled plasma mass spectrometry (ICP-MS),8 neutron activation analysis,9 anodic stripping voltammetry,10 and X-ray fluorescence spectrophotometry11 are the commonly used techniques for mercury detection. The main limitations of these methods lie in valuable, complex instruments, long data-acquisition time, and the requirement of professionally trained personnel. In the past decades, a great number of fluorescent probes and elegantly designed DNA-based biosensors have been reported for mercury detection.12 Though highly sensitive and selective, most of these methods only give response to Hg2+ ions and cannot work for other mercury species.12a,b
In fact, when mercury is released into the environment during various natural events or due to human activities, inorganic mercury can be converted into organic forms (e.g. methylmercury, MeHg+) by anaerobic microorganisms.13 In addition, organomercury is much more poisonous than its inorganic form.14 The reason is that the fat-soluble property enables organomercury compounds to easily pass through biofilms and act as deadly neurotoxins for many eukaryotes including fish, animals, and humans,15 leading to prenatal brain injury, cognitive and motor impairment, visual acuity, hearing loss, etc.16 It has been reported that more than 80% mercury in human blood exists in the methyl form.17 Therefore, the development of a cost- and labour-effective sensing platform showing highly sensitive and selective response to both Hg2+ and organomercury is still urgently required.
As a newly discovered photophysical phenomenon, aggregation-induced emission (AIE) has attracted more and more attention in recent years, and has great promising potential for sensing and bioimaging applications.18 In this work, we prepared a new water soluble AIE dye (1,1,2,2-tetrakis[4-(3-methyl-1H-benzimidazol-1-yl)phenyl]ethylene tetraiodide, Tmbipe, Scheme 1) that could rapidly coordinate with Hg(II) species to form a dinuclear HgII tetracarbene complex, and thus reported a novel sensing strategy for the sensitive and selective detection of both Hg2+ ions and organic mercury (including methylmercury and phenylmercury (PhHg+)). Different from traditional AIE-active mechanisms, the fluorescence response of Tmbipe to Hg(II) species relies on two successive processes: mercury-mediated chelate ring formation and subsequent aggregation. Synergic rigidification of Tmbipe molecules by these two processes could give significant fluorescence enhancement and thus improved Hg2+ detection sensitivity. This probe was also demonstrated to work well for imaging detection of mercury species in living cells.
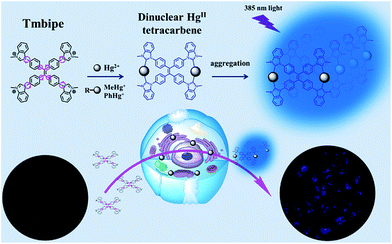 |
| Scheme 1 Structure of Tmbipe and its use in the detection of inorganic and organic mercury. | |
Results and discussion
Tmbipe preparation and its interaction with Hg(II) species
To achieve the sensitive detection of both Hg2+ ions and organomercury, a water-soluble AIE dye Tmbipe was designed and prepared following the synthetic route shown in Scheme S1.‡ Briefly, the precursor 1,1,2,2-tetrakis[4-(1H-benzimidazol-1-yl)phenyl]ethylene (Tbipe) was synthesized according to the reported method (Fig. S1‡).19 That is, the symmetric McMurry coupling reaction was used to prepare Tbipe in high yield. Then simple Hofmann alkylation between Tbipe and methyl iodide was carried out in dichloromethane to obtain the target probe Tmbipe. The details of the synthesis and characterization of Tmbipe are available in the ESI (Fig. S2 and S3‡). The results of both 1H-nuclear magnetic resonance (1H-NMR) and high resolution mass spectrometry (HRMS) characterization confirm the successful preparation of high purity Tbipe and Tmbipe with a chemical structure as shown in Scheme 1. Due to the presence of four positively charged methylated benzimidazole groups, Tmbipe shows excellent water solubility.
The tetraphenylethylene skeleton endows Tmbipe with typical AIE-based fluorescence features.20 Thus, the fluorescence property of Tmbipe was investigated. Both the C–C bonds between phenyl rings and C
C bonds and the C–N bonds between phenyl rings and benzimidazoles show high rotation tendencies (Scheme 1), which not only reduces the molecular planarity but also increases the possibility of radiationless relaxation, thus conferring Tmbipe with negligible fluorescence emission in aqueous solution. However, we were pleased to find that addition of Hg2+ and organomercury (e.g. MeHg+ and PhHg+) could result in significant enhancement of the fluorescence emission (Fig. 1), thus suggesting that Tmbipe might be used as a fluorescent probe for convenient detection of both Hg2+ and organomercury.
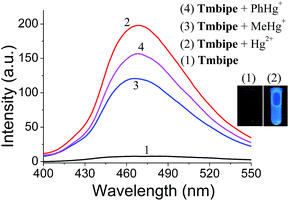 |
| Fig. 1 Fluorescence spectra of Tmbipe in the absence and presence of Hg2+, MeHg+ or PhHg+. [Tmbipe] = 10 μM; [Hg2+] = [MeHg+] = [PhHg+] = 20 μM. The inset shows the aqueous solution photograph of Tmbipe in the absence and presence of Hg2+ under UV light irradiation. | |
To understand why Hg2+ and organomercury can turn on the fluorescence of Tmbipe, the interactions between Tmbipe and Hg2+ (and organomercury) were investigated. First of all, Job's plot analysis was used to determine the binding stoichiometry between them. The results showed that Tmbipe bound to Hg2+, MeHg+ and PhHg+ with the same stoichiometry of 1
:
2 (Fig. S4‡), indicating that one Tmbipe molecule can bind with two molecules of Hg(II) species. The above results suggested that Tmbipe might bind to Hg2+ ions and organomercury in a similar manner.
Then, UV-vis and infrared (IR) absorption spectroscopies were used to further elucidate the binding interactions between Tmbipe and mercury species. Since the Hg2+ ion itself gives much simpler UV-vis and IR absorption signals than organomercury species, the IR and UV-vis spectra of the Tmbipe/Hg2+ mixture were recorded and compared with that of Tmbipe (Fig. 2). The UV-vis spectrum of Tmbipe showed an absorption peak at 265 nm. With the addition of increasing concentrations of Hg2+, the peak intensity continuously increased, which might be attributed to the coordination of mercury ions with Tmbipe.21 Interestingly, when the concentration ratio of Hg2+ to Tmbipe exceeded 1.0, an isosbestic point was observed at 340 nm, indicating that two Tmbipe/Hg2+ complexes existed in the mixture: one might be the complex with a stoichiometric ratio of 1
:
1, and the other might be the one with a stoichiometric ratio of 1
:
2. That is to say, in the low Hg2+ concentration range, one Tmbipe molecule preferentially binds with one Hg2+ ion to form a 1
:
1 complex. With the further addition of Hg2+ ions, the 1
:
1 complex will bind with a second Hg2+ ion to form the 1
:
2 complex. The IR spectrum of the Tmbipe/Hg2+ mixture clearly shows a new band centered at 1445 cm−1 compared to that of free Tmbipe, suggesting the existence of C–Hg bonds in the complex formed by Hg2+ and Tmbipe.18b Therefore, it can be speculated that one mercury atom simultaneously coordinates with two benzimidazole groups to form a dinuclear HgII tetracarbene complex Hg2Tmbipe, in which two 15-membered macrocycles are contained (Scheme 1).
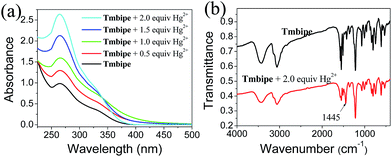 |
| Fig. 2 UV-vis (a) and IR (b) spectra of Tmbipe in the absence or presence of Hg2+. | |
Formation of the closed macrocycles could “lock” the free rotation of both C–N and C–C bonds in Tmbipe, resulting in a great increase of molecular planarity. Such a rigidification of the tetraphenylethylene-bridged Tmbipe molecule suppresses the radiationless deactivation pathways. As a result, the fluorescence of Tmbipe is turned on. A similar complexation reaction has recently been used by Sinha et al. to prepare dinuclear AgI and AuI tetracarbene complexes using two tetraphenylethylene-bridged tetraimidazolium salts as precursors.21a Different from the fact that a relatively long reaction time (2 days) and high temperature (60 °C) are required for AgI and AuI tetracarbene complex formation, our Hg2Tmbipe can be readily formed at ambient temperature, which is reflected by the fact that a stable and strong fluorescence signal output can be obtained within 3 min after Hg2+ addition (Fig. S5‡). Such a rapid signal response makes Tmbipe an excellent candidate for fluorescence sensing of mercury species.
Since Tmbipe shares common structural characteristics of AIE dyes, we want to determine if the mercury species-induced fluorescence enhancement is related with aggregation of Tmbipe or not. To answer this question, several techniques, including transmission electron microscopy (TEM), dynamic light scattering (DLS) and resonance light scattering (RLS), were used to characterize the morphology and size changes of Tmbipe before and after Hg2+ addition. DLS results (Fig. 3a) showed that free Tmbipe had an average size about 1.3 nm, indicating that it was monodisperse in solution. Addition of 2.0 equiv. of Hg2+ resulted in a sharp increase of the hydrodynamic size to around 1000 nm, suggesting the formation of large aggregates. The results of TEM characterization also demonstrated the Hg2+-induced aggregation of Tmbipe. As shown in Fig. 3b and c, almost no observable nanoparticles are shown in the TEM image of free Tmbipe. After addition of 2.0 equiv. of Hg2+, however, big clumps are clearly observed, thus giving direct evidence for Tmbipe aggregation in the presence of Hg2+. RLS is also an effective technique for studying the aggregation behavior of dyes. As shown in Fig. 3d, the RLS intensities of Tmbipe continuously increased with Hg2+ concentration, further demonstrating the size increase caused by Hg2+-induced aggregation. Collectively, when Tmbipe is mixed with Hg2+ ions, it will rapidly coordinate with two Hg2+ ions to form a highly planar dinuclear HgII tetracarbene complex, Hg2Tmbipe. The increase in molecular planarity can then promote the self-aggregation of Hg2Tmbipevia hydrophobic and π–π interactions. The formation of aggregates might further restrict the skeletal vibration of Tmbipe molecules, thus giving strong AIE fluorescence.
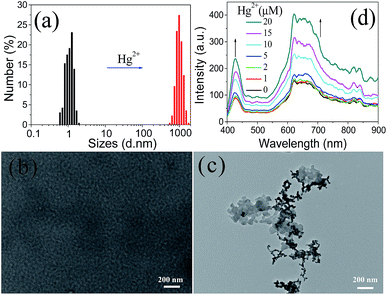 |
| Fig. 3 (a) DLS, (b and c) TEM and (d) RLS characterization of Tmbipe in the absence and presence of Hg2+. In (a) and (c), 2.0 equiv. of Hg2+ was added. | |
Selection of solvent for sensitive mercury species quantitation
Tmbipe is water soluble. This makes mercury species detection in pure aqueous media possible. However, we noticed that a very weak background fluorescence could also be observed without mercury species addition in pure aqueous solution (Fig. 4). To give a high signal-to-noise ratio in mercury species detection, it is best to decrease the background to a negligible level. Therefore, a suitable solvent system was screened. Fig. 4 shows the fluorescence change of Tmbipe in water/tetrahydrofuran (THF)-mixed solvents. In 100% aqueous solution, Tmbipe showed a very weak fluorescence, which might be attributed to the slight self-aggregation due to the hydrophobic and π–π interactions of the tetraphenylethene skeleton of Tmbipe. This self-aggregation was easily destroyed when a small amount of THF (e.g. 5%) was added, accompanied by the decrease of the fluorescence to a negligible level. The low background was maintained when the fraction of THF was increased to 80%. After that, the fluorescence significantly increased with the THF volume fraction, which can be rationally interpreted by the emergence of the AIE signal due to the aggregation of water-soluble Tmbipe in organic solvent. Therefore, a mixed solvent (5% THF–water) was used as the working solvent system for subsequent ultralow background detection of mercury species.
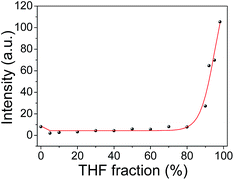 |
| Fig. 4 Fluorescence intensity of Tmbipe at 468 nm in different solvents. | |
It should be noted that Hg2+-induced fluorescence signal enhancement (Fig. 1 and 5) was much higher than that induced by solvent. This can be reasonably interpreted by their different working mechanisms. That is, in our Hg2+-induced aggregation system, the radiationless deactivation of Tmbipe can be overcome in two ways: formation of a planar dinuclear HgII tetracarbene complex with two chelate rings and subsequent aggregation of the tetracarbene complex. Such a synergic rigidification of tetraphenylethylene-bridged Tmbipe molecules can give significantly enhanced fluorescence. Such a highly efficient fluorescence enhancement mechanism is beneficial for the sensitive detection of Hg2+ ions. In a solvent-induced aggregation system, however, only aggregation but no chelate ring formation-induced probe molecule rigidification is used to turn on fluorescence. The effects of pH on the fluorescence response of Tmbipe to Hg2+ and organomercury species were also investigated. The results (Fig. S6‡) showed that pH had little effect on the fluorescence signals of the sensing systems, indicating that Tmbipe can be used for the detection of Hg2+ and organomercury species in a broad pH range.
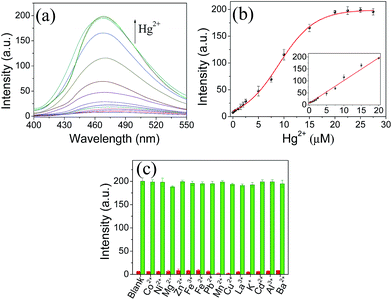 |
| Fig. 5 Quantitation of Hg2+. (a) Fluorescence spectra of the sensing systems containing different concentrations of Hg2+. (b) Hg2+ concentration-dependent changes in fluorescence intensity at 468 nm. The inset shows the linear relationship between the fluorescence intensity and Hg2+ concentration in the range of 200 nM–20 μM. (c) Selectivity of the Hg2+-sensing system. The red bars represent the fluorescence signals of the sensing systems in the presence of 100 μM other metal ions. The green bars represent the fluorescence signals of the sensing systems containing 20 μM Hg2+ and 100 μM other metal ions. | |
Sensitivity and selectivity of Hg2+ quantitation
Having demonstrated the feasibility of Hg2+ detection using Tmbipe as the AIE probe, the detection sensitivity was evaluated by recording the fluorescence response of the proposed sensing platform towards different concentrations of Hg2+. As shown in Fig. 5, Hg2+ concentration-dependent fluorescence signal increase was given by the sensing system, and a linear relationship (I = 6.44 + 9.50C (μM), R2 = 0.9962) between the fluorescence intensity and Hg2+ concentration was observed in the range of 200 nM–20 μM. Based on the rule of 3σ/S, the detection limit was calculated to be 63 nM.
The prepared Hg2+ probe exhibits good detection selectivity, which was demonstrated by testing the response of Tmbipe to other metal ions with 5 times the concentration of Hg2+ (Fig. 5c). None of these competitive metal ions, including Co2+, Ni2+, Mg2+, Zn2+, Fe3+, Fe2+, Pb2+, Mn2+, Cu2+, La3+, K+, Cd2+, Al3+ and Ba2+, could give an observable increase of fluorescence intensity, demonstrating the high selectivity of the probe for Hg2+ quantitation. More interestingly, the selectivity of Tmbipe was so high that it enabled selective Hg2+ detection even in the mixture of Hg2+ and other challenging metal ions. That is, when Hg2+ and another metal ion (5 times the Hg2+ concentration) were both added to the sensing system, the fluorescence signal increased to a level similar to that when Hg2+ alone was added, thus indicating that Hg2+ detection would not be interfered with by the presence of other metal ions. Such a high selectivity might be attributed to the formation of dinuclear HgII tetracarbene complexes, which requires the coordination of Hg2+ with carbon atoms in benzimidazole groups. Compared to other metal ions, Hg2+ has a much higher tendency to coordinate with carbon atoms, which can be demonstrated by the high conversion rate of Hg2+ to MeHg+ in the natural environment and extraordinary stability of MeHg+ in a hot sulfuric acid solution.22
Quantitation of organomercury compounds
Considering that organomercury is much more poisonous than Hg2+ due to its good lipid solubility, the sensitive and reliable detection of organomercury is more striking. Therefore, the feasibility of organomercury quantitation using the proposed sensing system was investigated by recording the changes in fluorescence intensity of Tmbipe (10 μM) as a function of organomercury concentration (Fig. 6). First, the applicability for MeHg+ detection was tested. Encouragingly, Tmbipe could also give a rapid fluorescence response to MeHg+, and about 16-fold fluorescence intensity increase was observed after addition of 2.0 equiv. of MeHg+. A good relationship (I = 8.57 + 6.31C (μM), R2 = 0.9902) was observed between fluorescence intensity and MeHg+ concentration in the range of 200 nM–20 μM. The detection limit was calculated to be 94 nM. To date, only a few fluorescent probes that can work for both Hg2+ and MeHg+ have been reported.18b,23 Compared to these probes, our Tmbipe shows comparable or better detection sensitivity (Table S1‡). Besides MeHg+, PhHg+, another organomercury species could also be easily detected by Tmbipe in the same way. The linear detection range was 200 nM–20 μM (I = 7.68 + 7.62C (μM), R2 = 0.9961) and the detection limit was 78 nM. These results, combined with those of Job plot analysis, suggested that both Hg2+ ions and organomercury can coordinate with Tmbipe to form dinuclear HgII tetracarbene complexes and AIE-active aggregates, thus making the simultaneous detection of inorganic and organic mercury species possible.
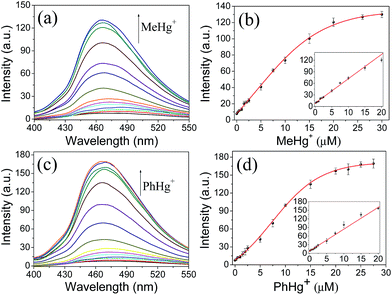 |
| Fig. 6 Quantitation of (a and b) MeHg+ or (c and d) PhHg+ using the Tmbipe probe. (a and c) Fluorescence spectra of the sensing systems containing different concentrations of (a) MeHg+ or (c) PhHg+. (b and d) MeHg+ or PhHg+ concentration-dependent changes in fluorescence intensity at 468 nm. The insets show the linear relationship between the fluorescence intensity and MeHg+ or PhHg+ concentration in the range of 200 nM–20 μM. | |
Fluorescence imaging in living cells
Having demonstrated the ability of Tmbipe to quantitatively detect Hg2+ ions and organomercury, its bioimaging application in detecting Hg(II) species in living cells was then explored. To achieve this, human cervical carcinoma HeLa cells were divided into three groups: one group was incubated with 10 μM Tmbipe probe for 30 min and the other two groups were treated with 10 μM or 20 μM Hg2+ for 1 h, respectively, followed by incubation with 10 μM Tmbipe for 30 min after removing non-accumulated Hg2+. As shown in Fig. 7, no evidence of fluorescence appeared in the HeLa cells without Hg2+ treatment, indicating that Tmbipe remained in a dispersed state in living cells containing no Hg(II) species. In contrast, blue fluorescence was observed from the cells contaminated with 1.0 equiv. of Hg2+, suggesting that probe molecules can efficiently pass through cell membranes and coordinate with intracellular Hg(II) species, giving an active fluorescence signal. When the Hg2+ concentration was increased to 2.0 equivalents, the fluorescence intensity was significantly enhanced, demonstrating that the observed fluorescence intensity is concentration-dependent. Similar results were observed for Hg(II)-imaging in HL7704 human adult hepatocyte cells and MCF-7 human breast cancer cells (Fig. S7‡). These results suggest that the prepared Tmbipe probe is able to detect the accumulation of mercury species in living cells.
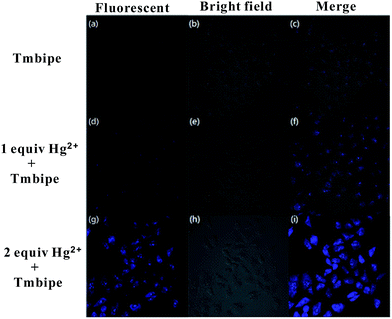 |
| Fig. 7 Detection of Hg(II) species in HeLa cells. | |
Conclusions
In summary, a new water-soluble AIE-based fluorescent probe, Tmbipe, which contains a tetraphenylethylene-bridged skeleton and four positively charged benzimidazole groups, was designed, synthesized and well characterized. This probe could coordinate with both Hg2+ and organomercury to form planar dinuclear HgII tetracarbene complexes, which would self-aggregate to give active AIE fluorescence. Synergic rigidification of the tetraphenylethylene-bridged Tmbipe molecule by mercury-mediated chelate ring formation and subsequent aggregation could result in higher fluorescence enhancement than that given by single aggregation-induced AIE activation. The fluorescence response of Tmbipe to Hg(II) species was so rapid that it could reach a maximum in 3 min. The unique dinuclear HgII tetracarbene formation-based working mechanism endows Tmbipe with highly specific response towards Hg2+ and organomercury species. These attractive characteristics endow Tmbipe with the ability to sensitively and selectively detect both Hg2+ ion and organomercury species. This probe was also demonstrated to work well for the detection of mercury species accumulation in living cells.
Experimental
Materials and reagents
Methylmercury, phenylmercury, iodomethane, 1H-benzimidazole and 4,4′-difluorobenzophenone were obtained from Sigma. TiCl4, tetrahydrofuran (THF), copper power, dimethyl sulfoxide (DMSO), zinc powder, ethyl acetate, diethyl ether and other metal salts (Hg(Ac)2, Co(Ac)2, Ni(NO3)2, Mg(NO3)2, Zn(Ac)2, Fe(NO3)3, Fe(NO3)2, Pb(NO3)2, Mn(Ac)2, Cu(NO3)2, La(NO3)3, KAc, Cd(NO3)2, Al(NO3)3 and Ba(NO3)2) were obtained from Jiangtian Co. Ltd. (Tianjin, China). Deionized and sterilized water (resistance > 18 MΩ cm−1) was used in all the experiments. All chemical reagents were of analytical grade and used without further purification.
Instruments and measurements
The 1H-nuclear magnetic resonance (1H-NMR) spectra were recorded in DMSO-d6 on a Bruker Spectrospin Avance 400 MHz NMR spectrophotometer. Chemical shifts are given in ppm downfield from tetramethylsilane. Infrared (IR) spectra were measured on a Perkin Elmer Spectrum One spectrophotometer. UV-vis absorption spectra were recorded on a Cary 60 UV-vis spectrophotometer (Agilent Technologies). High resolution mass spectrometry (HRMS) was carried out using an Agilent 6520 Q-TOF LC/MS mass spectrometer. Elemental analysis was performed on a Perkin-Elmer 240 elemental analyzer. Fluorescence spectra and resonance light scattering (RLS) spectra were measured at room temperature using a SHIMADZU RF-5301PC spectrofluorimeter. The transmission electron micrograph (TEM) images were recorded on a German Leica TCS-SP8 transmission electron microscope. A Malvern Zetasizer Nano ZS90 particle size analyzer was used for dynamic light scattering (DLS) studies.
Synthesis and characterization of Tmbipe
The synthetic route to Tmbipe is shown in Scheme S1.‡ The precursor Tbipe was synthesized according to the reported method.19 Its 1H-NMR spectrum is given in Fig. S1.‡ Then, the compound Tbipe (0.500 g, 0.630 mmol) and an excess of iodomethane (0.716 g, 5.040 mmol) were dissolved in 10 mL of dry dichloromethane. The obtained reaction mixture was heated to reflux for 48 h. During this period, a pale yellow precipitate was generated. After cooling of the reaction mixture to ambient temperature, the precipitate was isolated by filtration, washed with diethyl ether and then dried in vacuo to give a pale yellow solid. 1H-NMR (400 MHz, DMSO-d6), δ = 10.18 (s, 4H), 8.16 (d, 4H), 7.79–7.83 (m, 16H), 7.70–7.72 (d, 4H), 7.61–7.63 (d, 8H), 4.18 ppm (s, 12H); HRMS (ESI, positive ions): m/z = 214.0998 (calcd for Tmbipe4+ = 214.2682). Elemental analysis calcd (%) for C58H48N8I4: C, 51.05; H, 3.55; N, 8.21; found: C, 51.15; H, 3.42; N, 8.09.
Detection of Hg2+ and organomercury
A stock solution of Tmbipe (1 mM) was prepared in deionized water. The mixture of 10 μM Tmbipe and different concentrations of Hg2+, methylmercury or phenylmercury was prepared in a mixed solvent (THF
:
H2O = 5
:
95). After incubation at ambient temperature for 3 min, the fluorescence spectrum in the range of 400–550 nm was recorded under the excitation of 385 nm (excitation slit = emission slit = 5 nm), and the fluorescence intensity at 468 nm was used for mercury species quantitation.
Detection of Hg(II) species in living cells
HeLa cells and MCF-7 cells were cultured in Dulbecco's modified Eagle's medium (DMEM) supplemented with 10% fetal bovine serum (FBS). HL 7702 cells were cultured in RPMI 1640 medium supplemented with 10% FBS. Cells in the exponential phase of growth were seeded into 24 well plates and then incubated with 10 μM or 20 μM Hg2+ for 1 h at 37 °C. After being washed three times with PBS, the cells were incubated with 10 μM Tmbipe for another 30 min. Then, the cells were washed three times with PBS buffer. Fluorescence imaging of living cells was performed on an Olympus IX-81 microscope.
Conflicts of interest
There are no conflicts to declare.
Acknowledgements
This work was supported by the National Natural Science Foundation of China (no. 21371130 and 21728801), the Natural Science Foundation of Tianjin (no. 15JCYBJC48300 and 16JCYBJC19900), and the Innovation Fund of Tianjin University.
Notes and references
- K. H. Kim, E. Kabir and S. A. Jahan, J. Hazard. Mater., 2016, 306, 376 CrossRef CAS PubMed.
- C. O. R. Okpala, G. Sardo, S. Vitale, G. Bono and A. Arukwe, Crit. Rev. Food Sci. Nutr., 2018, 58, 1986 CrossRef CAS PubMed.
- J. D. Park and W. Zheng, J. Prev. Med. Public Health, 2012, 45, 344 CrossRef PubMed.
- J. Delafiori, G. Ring and A. Furey, Talanta, 2016, 153, 306 CrossRef CAS PubMed.
- G. A. Zachariadis, J. Chromatogr. A, 2013, 1296, 47 CrossRef CAS PubMed.
- H. Bagheri and M. Naderi, J. Hazard. Mater., 2009, 165, 353 CrossRef CAS PubMed.
- X. P. Yan, X. B. Yin, D. Q. Jiang and X. W. He, Anal. Chem., 2003, 75, 1726 CrossRef CAS PubMed.
- C. Y. Peng, M. He, B. B. Chen, L. J. Huang and B. Hu, Analyst, 2017, 142, 4570 RSC.
- P. Movalli, P. Bode, R. Dekker, L. Fornasari, S. Van der Mije and R. Yosef, Environ. Sci. Pollut. Res., 2017, 24, 25986 CrossRef CAS PubMed.
- E. S. Almeida, E. M. Richter and R. A. A. Munoz, Anal. Chim. Acta, 2014, 837, 38 CrossRef CAS PubMed.
- G. Elias, E. Margui, S. Diez and C. Fontas, Anal. Chem., 2018, 90, 4756 CrossRef CAS PubMed.
-
(a) H. N. Kim, W. X. Ren, J. S. Kim and J. Yoon, Chem. Soc. Rev., 2012, 41, 3210 RSC;
(b) E. M. Nolan and S. J. Lippard, Chem. Rev., 2008, 108, 3443 CrossRef CAS PubMed;
(c) J. Chan, S. C. Dodani and C. J. Chang, Nat. Chem., 2012, 4, 973 CrossRef CAS PubMed;
(d) W. Cai, S. B. Xie, J. Zhang, D. Y. Tang and Y. Tang, Biosens. Bioelectron., 2017, 98, 466 CrossRef CAS PubMed;
(e) H. Xie, Q. Wang, Y. Q. Chai, Y. L. Yuan and R. Yuan, Biosens. Bioelectron., 2016, 86, 630 CrossRef CAS PubMed;
(f) X. L. Liang, L. Wang, D. Wang, L. W. Zeng and Z. Y. Fang, Chem. Commun., 2016, 52, 2192 RSC;
(g) P. Zheng, M. Li, R. Jurevic, S. K. Cushing, Y. X. Liu and N. Q. Wu, Nanoscale, 2015, 7, 11005 RSC;
(h) B. Tang, B. Y. Ding, K. H. Xu and L. L. Tong, Chem.–Eur. J., 2009, 15, 3147 CrossRef CAS PubMed;
(i) Y. C. Chen, W. J. Zhang, Y. J. Cai, R. T. K. Kwok, Y. B. Hu, J. W. Y. Lam, X. G. Gu, Z. K. He, Z. Zhao, X. Y. Zheng, B. Chen, C. Gui and B. Z. Tang, Chem. Sci., 2017, 8, 2047 RSC;
(j) N. Zhao, J. W. Y. Lam, H. H. Y. Sung, H. M. Su, I. D. Williams, K. S. Wong and B. Z. Tang, Chem.–Eur. J., 2014, 20, 133 CrossRef CAS PubMed;
(k) X. J. Liu, C. Qi, T. Bing, X. H. Cheng and D. H. Shangguan, Anal. Chem., 2009, 81, 3699 CrossRef CAS PubMed;
(l) S. J. Wang, X. Li, J. Q. Xie, B. Y. Jiang, R. Yuan and Y. Xiang, Sens. Actuators, B, 2018, 259, 730 CrossRef CAS;
(m) W. H. Wang, T. S. Kang, P. W. H. Chan, J. J. Lu, X. P. Chen, C. H. Leung and D. L. Ma, Sci. Technol. Adv. Mater., 2015, 16, 065004 CrossRef PubMed;
(n) D. S. H. Chan, H. M. Lee, C. M. Che, C. H. Leung and D. L. Ma, Chem. Commun., 2009, 48, 7479 RSC;
(o) X. J. Xue, F. Wang and X. G. Liu, J. Am. Chem. Soc., 2008, 130, 3244 CrossRef CAS PubMed;
(p) S.-M. Jia, X.-F. Liu, P. Li, D.-M. Kong and H.-X. Shen, Biosens. Bioelectron., 2011, 27, 148 CrossRef CAS PubMed;
(q) D.-M. Kong, N. Wang, X.-X. Guo and H.-X. Shen, Analyst, 2010, 135, 545 RSC;
(r) Q. Zhang, Y. Cai, H. Li, D.-M. Kong and H.-X. Shen, Biosens. Bioelectron., 2012, 38, 331 CrossRef CAS PubMed;
(s) Q. Duan, X. Lv, C. Liu, Z. Geng, F. Zhang, W. Sheng, Z. Wang, P. Jia, Z. Li, H. Zhu and B. Zhu, Ind. Eng. Chem. Res., 2019, 58, 11 CrossRef CAS;
(t) Q. Duan, H. Zhu, C. Liu, R. Yuan, Z. Fang, Z. Wang, P. Jia, Z. Li, W. Sheng and B. Zhu, Analyst, 2019, 144, 1426 RSC.
-
(a) K. S. Hoy, W. Feng and X. C. Le, J. Environ. Sci., 2018, 68, 218 CrossRef PubMed;
(b) T. Zhang, B. Kim, C. Levard, B. C. Reinsch, G. V. Lowry, M. A. Deshusses and H. Hsu-Kim, Environ. Sci. Technol., 2012, 46, 6950 CrossRef CAS PubMed;
(c) S. M. Ullrich, T. W. Tanton and S. A. Abdrashitova, Crit. Rev. Environ. Sci. Technol., 2001, 31, 241 CrossRef CAS.
- S. Díez, Rev. Environ. Contam. Toxicol., 2009, 198, 111 CrossRef PubMed.
-
(a) F. A. Duarte, B. M. Soares, A. A. Vieira, E. R. Pereira, J. V. Maciel, S. S. Caldas and E. G. Primel, Anal. Chem., 2013, 85, 5015 CrossRef CAS PubMed;
(b) L. Yang, V. Colombini, P. Maxwell, Z. Mester and R. E. Sturgeon, J. Chromatogr. A, 2003, 1011, 135 CrossRef CAS PubMed.
- K. Eto, H. Tokunaga and K. Nagashima, Toxicol. Pathol., 2002, 30, 714 CrossRef CAS PubMed.
-
(a) J. S. Park, J. S. Lee, G. B. Kim, J. S. Cha, S. K. Shin, H. G. Kang, E. J. Hong, G. T. Chung and Y. H. Kim, Water, Air, Soil Pollut., 2010, 207, 391 CrossRef CAS;
(b) H. Akagi and A. Naganuma, J. Health Sci., 2000, 46, 323 Search PubMed.
-
(a) H.-T. Feng, Y.-X. Yuan, J.-B. Xiong, Y.-S. Zheng and B.-Z. Tang, Chem. Soc. Rev., 2018, 47, 7452 RSC;
(b) A. Chatterjee, M. Banerjee, D. G. Khandare, R. U. Gawas, S. C. Mascarenhas, A. Ganguly, R. Gupta and H. Joshi, Anal. Chem., 2018, 234, 12698 Search PubMed;
(c) X. Feng, C. X. Qi, H. T. Feng, Z. Zhao, H. H. Y. Sung, I. D. Williams, R. T. K. Kwok, J. W. Y. Lam, A. J. Qin and B. Z. Tang, Chem. Sci., 2018, 9, 5679 RSC;
(d) X. Z. Yan, H. Z. Wang, C. E. Hauke, T. R. Cook, M. Wang, M. L. Saha, Z. X. Zhou, M. M. Zhang, X. P. Li, F. H. Huang and P. J. Stang, J. Am. Chem. Soc., 2015, 137, 15276 CrossRef CAS PubMed.
- S. Odabas, E. Tekin, F. Turksoy and C. Tanyeli, J. Lumin., 2016, 176, 240 CrossRef CAS.
-
(a) J. Shi, Q. Deng, Y. Li, M. Zheng, Z. Chai, C. Wan, Z. Zheng, L. Li, F. Huang and B. Tang, Anal. Chem., 2018, 90, 13775 CrossRef CAS PubMed;
(b) S. J. Liu, Y. H. Cheng, H. K. Zhang, Z. J. Qiu, R. T. K. Kwok, J. W. Y. Lam and B. Z. Tang, Angew. Chem., Int. Ed., 2018, 57, 6274 CrossRef CAS PubMed;
(c) Q. Zhang, Y.-C. Liu, D.-M. Kong and D.-S. Guo, Chem.–Eur. J., 2015, 21, 13253 CrossRef CAS PubMed;
(d) H.-X. Jiang, M.-Y. Zhao, C.-D. Niu and D.-M. Kong, Chem. Commun., 2015, 51, 16518 RSC.
-
(a) N. Sinha, L. Stegemann, T. T. Y. Tan, N. L. Doltsinis, C. A. Strassert and F. E. Hahn, Angew. Chem., Int. Ed., 2017, 56, 2785 CrossRef CAS PubMed;
(b) M.-H. Yu, H.-H. Yang, A. R. Naziruddin, S. Kanne, B. H. Wang, F. C. Liu, I. J. B. Lin and G. H. Lee, Eur. J. Inorg. Chem., 2016, 2016, 4829 CrossRef CAS.
-
M. Schlosser, Organometallics in Synthesis, 3rd edn, Wiley, New York, 2012 Search PubMed.
-
(a) S.-L. Pan, K. Li, L.-L. Li, M.-Y. Li, L. Shi, Y.-H. Liu and X.-Q. Yu, Chem. Commun., 2018, 54, 4955 RSC;
(b) M. Deng, D. Gong, S.-C. Han, X. Zhu, A. Iqbal, W. Liu, W. Qin and H. Guo, Sens. Actuators, B, 2017, 243, 195 CrossRef CAS;
(c) J. Garcia-Calvo, S. Vallejos, F. C. Garcia, J. Rojo, J. M. Garcia and T. Torroba, Chem. Commun., 2016, 52, 11915 RSC;
(d) B. Díaz de Greñu, J. García-Calvo, J. V. Cuevas, G. García-Herbosa, B. García, N. Busto, S. Ibeas, T. Torroba, B. Torroba, A. Herrera and S. Pons, Chem. Sci., 2015, 6, 3757 RSC;
(e) L. Ding, Q. Zou and J. Su, Sens. Actuators, B, 2012, 168, 185 CrossRef CAS;
(f) Q. Zou and H. Tian, Sens. Actuators, B, 2010, 149, 20 CrossRef CAS;
(g) X. Chen, K.-H. Baek, Y. Kim, S.-J. Kim, I. Shin and J. Yoon, Tetrahedron, 2010, 66, 4016 CrossRef CAS;
(h) Y.-K. Yang, S.-K. Ko, I. Shin and J. Tae, Org. Biomol. Chem., 2009, 7, 4590 RSC.
Footnotes |
† This work is dedicated to Professor Dai-Zheng Liao on the occasion of his 80th birthday. |
‡ Electronic supplementary information (ESI) available. See DOI: 10.1039/c8sc05714a |
|
This journal is © The Royal Society of Chemistry 2019 |