DOI:
10.1039/C8SC05502E
(Edge Article)
Chem. Sci., 2019,
10, 3074-3079
Transition metal catalyzed stereodivergent synthesis of syn- and anti-δ-vinyl-lactams: formal total synthesis of (−)-cermizine C and (−)-senepodine G†
Received
10th December 2018
, Accepted 24th January 2019
First published on 24th January 2019
Abstract
A stereodivergent and diastereoselective transition-metal-catalyzed intramolecular hydroamidation of allenes and alkynes furnishing δ-vinyl-lactams is reported. Employing a rhodium catalyst allowed for the selective synthesis of the syn-δ-lactam. Conversely, a palladium catalyst led to the formation of the anti-δ-lactam in high selectivity. The new method shows high functional group compatibility and assorted synthetic transformations were demonstrated as well as its utility for the enantioselective formal total syntheses of (−)-cermizine C and (−)-senepodine G.
Transition metal-catalyzed intramolecular hydroamination reactions starting from aminoalkenes,1 aminoallenes,2 aminodienes3 and aminoalkynes4 have been reported frequently for the synthesis of nitrogen-containing heterocycles. Although the δ-lactam moiety is of equally high interest, to date reports of inter- and intramolecular hydroamidations are still rare and stereoselective and stereodivergent variants are unknown.5 Thus, δ- (and γ-) lactams are found as pharmacophores in a number of drugs and bioactives, such as (+)-ebmamonin (antiarrhythmic agent), rhynchophylline (treatment of disorder of the central nervous system) and BMD 188 (induces the apoptotic death of prostate cancer cells). Furthermore δ-lactams have been used as key intermediates for the synthesis of piperidine type alkaloids such as cermizine C & D and as senepodine G (Fig. 1).6
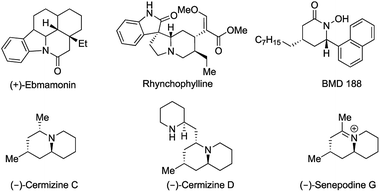 |
| Fig. 1 Bioactives and natural products. | |
Our group recently reported a series of inter- and intramolecular addition reactions of various nucleophiles and pronucleophiles to allenes and alkynes covering regio-, enantio- and diastereoselective C–O, C–S, C–N and C–C bond formations.7,8 Thus, these new methods represent atom-economic alternatives to the transition-metal-catalyzed allylic substitution9 and allylic oxidation.10 We herein disclose an unprecedented stereodivergent rhodium- and palladium-catalyzed intramolecular hydroamidation to gain selective access to either syn- or anti-vinyl-δ-lactams.
Our studies commenced by employing the phenyl-β-substituted tosyl amide model substrates 1 (Table 1).
Table 1 Optimization of the transition-metal catalyzed intramolecular hydroamidation11,12a
After a first successful reactivity test using a Rh/dppf catalytic system (d.r. 85/15), an optimization of the reaction parameters was undertaken (Table 1). Employing [Rh(COD)Cl]2/dppf enabled us to selectively obtain the syn-configurated product albeit in low conversion (entry 2). Fortunately, utilizing chloroacetic acid as a Brønsted acid additive could improve the conversion dramatically to 96% (entry 3). Furthermore, lowering the reaction temperature from 80 °C to room temperature led to optimal results (92% isolated yield) and diastereoselectivity of 91
:
9 in favor of the syn-diastereomer (entry 4). Conversely, by altering the metal source to palladium, a complete inversion of the diastereoselectivity in favor of the anti-diastereomer was observed. The combination of [Pd(dba)2], dppf and chloroacetic acid at 80 °C led to the best results in terms of diastereoselectivity (6/94) and yield (96%) (entry 8). Further modifying the reaction temperature or examining different additives and ligands showed no improvement.11
With optimized conditions in hand, the scope and limitations of this reaction were explored (Table 2). Alkyl-, vinyl- and cyclic-functionalized amides behaved well and furnished the corresponding syn- and anti-lactams 3a to 6a and 3b to 6b in good to excellent yields and d.r. Next an assorted variety of different functionalized, aromatic β-substituted amides was investigated. Substrates bearing phenyl, biaryl, naphthyl and vinyl groups provided the corresponding lactams in yields up to 95% and d.r. up to 96/4 (2a, 7a, 8a and 2b, 7b, 8b). Compounds 2a and 2b were also synthesized via large scale catalysis without any decline in yield and d.r., respectively.11 Sensitivity towards steric hindrance was tested employing meta and para substituted derivatives.13 Functional groups like thioethers and halides attached to the aromatic ring were compatible and afforded the desired products 12a & 13a and 12b & 13b in good to excellent yields. Besides β-substituted amides also α-substituted amides could be cyclized in good yields, though with lower diastereoselectivities (14a & 14b).
Table 2 Scope of the catalytic diastereoselective intramolecular amidation towards syn- and anti-configured δ-lactams11abc
All reactions were performed on a 0.3 mmol scale; dppf (1,1-bis(diphenylphosphino)ferrocene); ClCH2CO2H = chloroacetic acid; DCE = 1,2-dichloroethane.
Combined yield.
Selectivity determined by 1H-NMR analysis of the crude reaction mixture.
Yield and d.r. of large scale Rh-reaction (1.4 mmol).
Yield and d.r. of large scale Pd-reaction (3.1 mmol).
|
|
Overall the Pd-catalyzed reaction towards the anti-product showed slightly better results in terms of d.r. in case of aliphatic- and cyclic-functionalized amides compared to the Rh-catalyzed protocol. The relative configuration of syn- and anti-configured products were determined by NOE-experiments and confirmed by X-ray diffraction analysis (Fig. 2).11
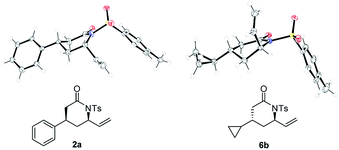 |
| Fig. 2 Crystal structure of 2a and 6b. | |
To extend the use of this reaction even further, alkyne substrates bearing isopropyl, phenyl and anisole as substituents were subjected to rhodium catalysis conditions (Table 3). The alkyne substrates needed higher reaction temperatures in order to obtain the δ-lactams in sufficiently high yields. Thus, yields up to 88% and diastereoselectivities up to 87/14 in favor of the syn-diastereomer were obtained (Table 3). Unfortunately, the palladium catalyst system did not show any reactivity for the terminal alkyne substrates.11
Table 3 Scope of the catalytic diastereoselective intramolecular amidation towards syn-configured compounds employing alkynes as substrate11abc
All reactions were performed on a 0.3 mmol scale; dppf (1,1-bis(diphenylphosphino)ferrocene); ClCH2CO2H = chloroacetic acid; DCE = 1,2-dichloroethane.
Combined yield.
Selectivity determined by 1H-NMR analysis of the crude reaction mixture.
|
|
To demonstrate that the reaction does not just provide access to highly diastereoselective lactams but also enables their stereoselective synthesis, enantiomerically enriched starting material 16 was subjected to the catalysis conditions.11,13
We were satisfied to observe that the enantiomeric purity was maintained under both the syn-3a- and anti-3b-catalysis conditions (Scheme 1).
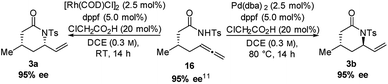 |
| Scheme 1 Enabling the stereoselective synthesis of syn- (3a) and anti-lactam (3b), by employing enantiomerically enriched starting material (16). | |
To investigate the reversibility of the reaction, both products were subjected to the contrary conditions.
The anti-lactam subjected to the “syn-conditions” showed no change of relative configuration. Conversely, the syn-lactam exposed to the “anti-conditions” resulted in an inversion of the relative configuration (Scheme 2).11 To gain insight into the respective stabilities of the syn- and the anti-product, DFT calculations (BP86/def2SVP) were performed. The calculations showed that the anti- is approximately 1.4 kcal mol−1 more stable than the syn-diastereomer.11
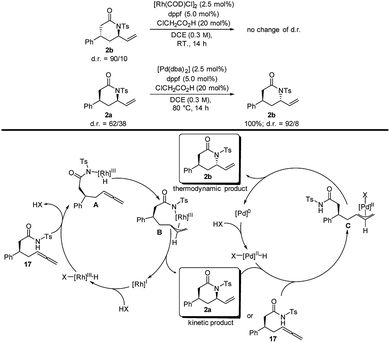 |
| Scheme 2 Reversibility investigations. | |
In conclusion, we posit that in presence of rhodium the reaction is driven by kinetic control, whereas in presence of palladium the reaction towards the syn-diastereomer is reversible and the formation of the more stable anti-diastereomer either under thermodynamic control or under product development control.11 The mechanism of the rhodium-catalyzed addition of nucleophiles to allenes and alkynes was investigated by our group recently.14 Based on those results, we propose the following mechanistic rationale for the formation of the syn-product (Scheme 2, left-hand cycle). First, the active rhodium species is generated via oxidative addition, followed by a ligand exchange to form intermediate A. Hydrometalation then gives rise to the key allylrhodium species B. In the configuration-determining step, a reductive elimination via an inner sphere mechanism takes place favoring the syn-product 7a.15 For the palladium-mediated reaction (Scheme 2, right-hand cycle) we suggested a similar mechanism to the Tsuji–Trost reaction.16 First the active palladium species undergoes hydrometalation to form the π-allyl species C. Then the C–N bond is formed by nucleophilic attack on to the π-allyl intermediate C, via an outer sphere mechanism, favoring the anti-product.
The utility of 2b as scaffold in the synthesis of more complex molecules was demonstrated by performing assorted transformations (Scheme 3). Ozonolysis of the allylic moiety delivered the C1-shortened aldehyde 18 in excellent yield (99%). The C1-chain-elongated aldehyde 19 was accessed through hydroformylation, employing the self-assembly ligand 6-diphenylphosphinopyridine (6-DPPon) in excellent yield (98%) and regioselectivity. Cleaving the tosyl group under reductive conditions yielded the unprotected lactam 20. Finally, a hydrolysis was performed as a gateway to access diastereomerically enriched δ-amino acid 21.
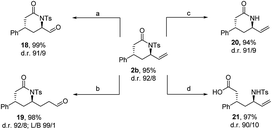 |
| Scheme 3 Follow-up chemistry; *was synthesized in a gram scale catalysis; (a) (i) O3, MeOH, −78 °C; (ii) SMe2, MeOH, −78 °C to RT, 99%; (b) [{Rh(CO)2acac}] (0.5 mol%), 6-DPPon (10 mol%), CO/H2 (1 : 1, 10 bar), toluene, 80 °C, 21 h, 98% (L/B > 95 : 5); (c) Li, naphthalene, THF, −78 °C to RT; (d) H2O/IPA, LiOH, reflux, 17 h. | |
Inspired by the variety of functionalization, the newly developed lactam synthesis was applied in the formal total synthesis of cermizine C and senepodine G (Scheme 4). Both natural products were isolated for the first time in 2004 from the club moss lycopodium carnuum and chinense by KOBAYASHI.17 This representative from the lycopodium family as well as even more complex alkaloids were often targeted in the total synthesis, due to their high variety, unusual skeletons and biological properties.18–20 Some efforts were made to find an easy access to these alkaloids and especially to form the main core unit.21 A first synthesis was reported by Snider starting from (S)-piperidine ethanol.22 Even though the synthesis was efficient and elegant, the starting material is expensive and its synthesis not trivial. Other attempts were either based on long reaction sequencesor used auxiliary chemistry to introduce the desired stereochemistry.23,24
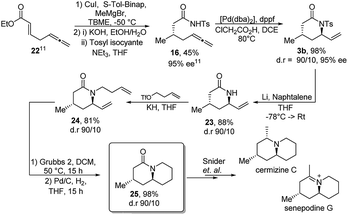 |
| Scheme 4 Formal total synthesis of cermizine C and senepodine G. | |
Our interest in cermizine C and senepodine G was initially stimulated by the quinolizidine core, which is accessible in a straightforward fashion by applying the present methodology (Scheme 4). The attempt for the enantioselective formal total synthesis of this compounds was therefore started from (S)-methyltosylamide (16) which was accessed in two steps from an α,β-unsaturated ester 22.11
The enantiomerically enriched starting material 16 was subjected to a gram-scale catalytic cyclization and delivered the tosyl-protected lactam 3b in excellent yield (98%), diastereoselectivity (d.r. = 90/10) and enantioselectivity (95% ee). Next was the deprotection of tosyl–lactam 3b followed by an alkylation reaction to obtain 24 in a good yield. With precursor 24 in hand, a Grubbs ring closing metathesis followed by catalytic hydrogenation furnished the quinolizidine core 25, which was previously converted into cermizine C and senepodine G by Snider et. al.22 Hence, we have realized a highly efficient stereoselective formal total synthesis of these alkaloids (7 steps, 31% overall yield) starting from compound 22. In comparison Snider was able to synthesis compound 25 in 5 steps and an overall yield of 41%, starting from (S)-piperidine ethanol.25 However, our method compares favorably, in terms of starting material accessibility and costs, to the procedure developed by Snider.
Conclusions
In conclusion, we have established a general and efficient stereodivergent and highly diastereoselective procedure to gain selective access to syn- and anti-vinyl-δ-lactams by using either a rhodium or palladium-based catalytic system. The reaction tolerates a wide range of functional groups which enables the synthesis of a variety of different δ-lactams. Assorted transformations allowed the functionalization of both the alkene and lactam moiety. Furthermore, we successfully utilized the new developed methodology in a highly stereospecific and atom economic formal total synthesis of cerminzin C and senepodine G.
Conflicts of interest
There are no conflicts to declare.
Acknowledgements
We thank Solvias, Umicore, BASF, and Wacker for generous gifts of chemicals. Dr Manfred Keller, Dr Daniel Kratzert and Felix Bauer are acknowledged for highly qualified NMR-, X-ray-analysis and DFT calculations.
Notes and references
-
(a) Y. K. Kim, T. Livinghouse and J. E. Bercaw, Tetrahedron Lett., 2001, 16, 2933–2935 CrossRef;
(b) G. A. Molander and E. D. Dowdy, J. Org. Chem., 1998, 63, 8983–8988 CrossRef CAS.
-
(a) V. M. Arredondo, F. E. McDonald and T. J. Marks, J. Am. Chem. Soc., 1998, 120, 4871–4872 CrossRef CAS;
(b) M. Meguro and Y. Yamamoto, Tetrahedron Lett., 1998, 39, 5421–5424 CrossRef CAS;
(c) R. L. LaLonde, B. D. Sherry, E. J. Kang and F. D. Toste, J. Am. Chem. Soc., 2007, 129, 2452–2453 CrossRef CAS PubMed.
-
(a) S. Hong, A. M. Kawaoka and T. J. Marks, J. Am. Chem. Soc., 2003, 125, 15878–15892 CrossRef CAS PubMed;
(b) S. Hong and T. J. Marks, J. Am. Chem. Soc., 2002, 124, 7886–7887 CrossRef CAS PubMed.
-
(a) L. Ackermann, R. G. Bergman and R. N. Loy, J. Am. Chem. Soc., 2003, 125, 11956–11963 CrossRef CAS PubMed;
(b) I. Bytschkov and S. Doye, Tetrahedron Lett., 2002, 43, 3715–3718 CrossRef CAS;
(c) T. E. Müller, M. Grosche, E. Herdtweck, A. K. Pleier, E. Walter and Y. K. Yan, Organometallics, 2000, 19, 170–183 CrossRef;
(d) L. M. Lutete, I. Kadota and Y. Yamamoto, J. Am. Chem. Soc., 2004, 126, 1622–1623 CrossRef CAS PubMed;
(e) G. B. Bajracharya, Z. Huo and Y. Yamamoto, J. Org. Chem., 2005, 70, 4883–4886 CrossRef CAS PubMed.
-
(a) N. T. Patil, Z. Huo, G. B. Bajracharya and Y. Yamamoto, J. Org. Chem., 2006, 71, 3612–3614 CrossRef CAS PubMed;
(b) Y. Yu, G. A. Stephenson and D. Mitchell, Tetrahedron Lett., 2006, 47, 3811–3814 CrossRef CAS;
(c) L. Zhang, D. Ye, Y. Zhou, G. Liu, E. Feng, H. Jiang and H. Liu, J. Org. Chem., 2010, 75, 3671–3677 CrossRef CAS PubMed;
(d) S. Obika, Y. Yasui, R. Yanada and Y. Takemoto, J. Org. Chem., 2008, 73, 5206–5209 CrossRef CAS PubMed.
- Y. Shimoji, K. Tomita, T. Hashimoto, F. Saito, Y. Morisawa, H. Mizuno, R. Yorikane and H. Koike, J. Med. Chem., 1992, 35, 816–822 CrossRef CAS PubMed; A. G. Schultz and L. Pettus, J. Org. Chem, 1997, 62, 6855–6861 CrossRef; D. G. Tang,
et al.
, Pathol. Oncol. Res., 1998, 179–190 CrossRef; J. S. Shi, J. X. Yu, X. P. Chen and R. X. Xu, Acta Pharmacologica Sinica, 2003, 24, 97–101 Search PubMed.
- For the addition of nucleophiles and pronucleophiles to allenes/alkynes, see:
(a) K. Xu, N. Thieme and B. Breit, Angew. Chem., Int. Ed., 2014, 53, 2162–2165 (
Angew. Chem.
, 2014
, 126
, 2194–2197
) CrossRef CAS PubMed;
(b) C. Li, M. Kähny and B. Breit, Angew. Chem., Int. Ed., 2014, 53, 13780–13784 (
Angew. Chem.
, 2014
, 126
, 14000–14004
) CrossRef CAS PubMed;
(c) P. Koschker and B. Breit, Acc. Chem. Res., 2016, 49, 1524–1536 CrossRef CAS PubMed;
(d) C. Li and B. Breit, J. Am. Chem. Soc., 2014, 136, 862–865 CrossRef CAS PubMed;
(e) A. M. Haydl, L. J. Hilpert and B. Breit, Chem.–Eur. J., 2016, 22, 6547–6551 CrossRef CAS PubMed;
(f) A. M. Haydl, D. Berthold, P. A. Spreider and B. Breit, Angew. Chem., Int. Ed., 2016, 55, 5765–5769 (
Angew. Chem.
, 2016
, 128
, 5859–5863
) CrossRef CAS PubMed;
(g) T. M. Beck and B. Breit, Org. Lett., 2016, 18, 124–127 CrossRef CAS PubMed;
(h) S. Ganss and B. Breit, Angew. Chem., Int. Ed., 2016, 55, 9738–9742 (
Angew. Chem.
, 2016
, 128
, 9890–9894
) CrossRef CAS PubMed;
(i) P. A. Spreider, A. M. Haydl, M. Heinrich and B. Breit, Angew. Chem., Int. Ed., 2016, 55, 15569–15573 (
Angew. Chem.
, 2016
, 128
, 15798–15802
) CrossRef CAS PubMed;
(j) T. M. Beck and B. Breit, Eur. J. Org. Chem., 2016, 5839–5844 CrossRef CAS;
(k) N. Thieme and B. Breit, Angew. Chem., 2017, 129, 1542–1546 (
Angew. Chem., Int. Ed.
, 2017
, 56
, 1520–1524
) CrossRef;
(l) T. M. Beck and B. Breit, Angew. Chem., Int. Ed., 2017, 56, 1903–1907 (
Angew. Chem.
, 2017
, 129
, 1929–1933
) CrossRef CAS PubMed;
(m) J. Schmidt, C. Li and B. Breit, Chem.–Eur. J., 2017, 23, 6531–6534 CrossRef CAS PubMed;
(n) D. Berthold and B. Breit, Org. Lett., 2018, 20(3), 598–601 CrossRef CAS;
(o) C. Grugel and B. Breit, Org. Lett., 2018, 20(4), 1066–1069 CrossRef CAS PubMed.
-
(a) Q. A. Chen, Z. Chen and V. M. Dong, J. Am. Chem. Soc., 2015, 137, 8392–8395 CrossRef CAS PubMed;
(b) T. Kawamoto, S. Hirabayashi, X. Guo, T. Nishimura and T. Hayashi, Chem. Commun., 2009, 24, 3528–3530 RSC;
(c) A. B. C. Simas, B. Plietker, C. Jäkel, J. Xie and B. M. Trost, Chem.–Eur. J., 2005, 11, 7075–7082 CrossRef PubMed;
(d) J. Xie, J. D. Sieber and B. M. Trost, J. Am. Chem. Soc., 2011, 133, 20611–20622 CrossRef PubMed;
(e) F. Kleinbeck and F. D. Toste, J. Am. Chem. Soc., 2009, 131, 9178–9179 CrossRef CAS PubMed;
(f) R. M. Zeldin and F. D. Toste, Chem. Sci., 2011, 2, 1706 RSC;
(g) C. Liu and R. A. Widenhoefer, Org. Lett., 2007, 9, 1935–1938 CrossRef CAS PubMed;
(h) K. L. Toups, G. T. Liu and R. A. Widenhoefer, J. Organomet. Chem., 2009, 694(4), 571–575 CrossRef CAS PubMed;
(i) W. Brieden, K. H. Baringhaus and B. M. Trost, Angew. Chem., Int. Ed., 1992, 31, 1335–1336 (
Angew. Chem.
, 1992
, 104
, 1392–1394
) CrossRef;
(j) L. M. Lutete, I. Kadota and Y. Yamamoto, J. Am. Chem. Soc., 2004, 126, 1622–1623 CrossRef CAS PubMed;
(k) F. A. Cruz, Z. Chen, S. i. Kurtoic and V. M. Dong, Chem. Commun., 2016, 52, 5836–5839 RSC;
(l) M. Narsireddy and Y. Yamamoto, J. Org. Chem., 2008, 73, 9698–9709 CrossRef CAS PubMed.
-
(a) B. M. Trost and D. L. Van Vranken, Chem. Rev., 1996, 96, 395–422 CrossRef CAS PubMed;
(b) B. M. Trost and M. L. Crawley, Chem. Rev., 2003, 103, 2921–2943 CrossRef CAS PubMed;
(c) Z. Lu and S. Ma, Angew. Chem., Int. Ed., 2008, 47, 258–297 (
Angew. Chem.
, 2008
, 120
, 264–303
) CrossRef CAS PubMed;
(d) D. C. Vrieze, G. S. Hoge, P. Z. Hoerter, J. T. Van Haitsma and B. M. Samas, Org. Lett., 2009, 11, 3140–3142 CrossRef CAS PubMed;
(e) T. Hayashi, A. Okada, T. Suzuka and M. Kawatsura, Org. Lett., 2003, 5, 1713–1715 CrossRef CAS PubMed;
(f) P. A. Evans and D. K. Leahy, J. Am. Chem. Soc., 2002, 124, 7882–7883 CrossRef CAS PubMed;
(g) G. Lipowsky, N. Miller and G. Helmchen, Angew. Chem., Int. Ed., 2004, 43, 4595–4597 (
Angew. Chem.
, 2004
, 116
, 4695–4698
) CrossRef CAS PubMed;
(h) K.-Y. Ye, H. He, W.-B. Liu, L.-X. Dai, G. Helmchen and S.-L. You, J. Am. Chem. Soc., 2011, 133, 19006–19104 CrossRef CAS PubMed;
(i) W. Chen and J. F. Hartwig, J. Am. Chem. Soc., 2013, 135, 2068–2071 CrossRef CAS PubMed;
(j) W. Chen and J. F. Hartwig, J. Am. Chem. Soc., 2014, 136, 377–3837 CrossRef CAS PubMed;
(k) S. Krautwald, D. Sarlah, M. A. Schafroth and E. M. Carreira, Science, 2013, 340, 1065–1068 CrossRef CAS PubMed;
(l) J. Y. Hamilton, D. Sarlah and E. M. Carreira, Angew. Chem., Int. Ed., 2013, 52, 7532–7535 (
Angew. Chem.
, 2013
, 125
, 7680–7683
) CrossRef CAS PubMed.
-
(a) G. Liu and Y. Wu, Top. Curr. Chem., 2009, 292, 195–209 CrossRef;
(b) M. S. Chen and M. C. White, J. Am. Chem. Soc., 2004, 126, 1346–1347 CrossRef CAS PubMed;
(c) G. Liu and S. S. Stahl, J. Am. Chem. Soc., 2007, 129, 6328–6335 CrossRef CAS PubMed;
(d) G. Yin, Y. Wu and G. Liu, J. Am. Chem. Soc., 2010, 132, 11978–11987 CrossRef CAS PubMed.
- For further information see ESI.†.
- Due to the hazardous nature of DCE different solvents like DCM, THF, toluene, acetonitrile, ethanol and PhF were screened during the optimization of the Rh-catalyzed reaction. Unfortunately, DCE showed the best result and was therefore chosen. See ESI† for further information.
- Synthesis of 17 see ESI† or Scheme 4.
- U. Gellrich, A. Meißner, A. Steffani, M. Kähny, H.-J. Drexler, D. Heller, D. A. Plattner and B. Breit, J. Am. Chem. Soc., 2014, 136, 1097–1104 CrossRef CAS PubMed.
- In assumption, that the formation of π-allyl rhodium species B is not reversible the stereoselectivity determining step takes place during the allene hydrometalation and not during the reductive elimination step.
-
(a) B. M. Trost and T. R. Verhoeven, J. Org. Chem., 1976, 41, 3215–3216 CrossRef CAS;
(b) H. Kurosawa, J. Organomet. Chem., 1987, 334, 243–253 CrossRef CAS;
(c) B. M. Trost, T. zhang and J. D. Sieber, Chem. Sci., 2010, 1, 427–440 RSC.
- H. Morita, Y. Hirasawa, T. Shinzato and J. Kobayashi, Tetrahedron, 2004, 7015–7023 CrossRef CAS.
- For a review of lycopodium alkaloids, see; X. Ma and D. R. Gang, Nat. Prod. Rep., 2004, 21, 752–772 RSC.
- For earlier discovered alkaloids from this family see:
(a)
W. A. Ayer and L. S. Trifonov, The Alkaloids, ed. G. A. Cordell and A. Brossi, Academic Press, New York, 1985, vol. 26, p. 241 Search PubMed;
(b) W. A. Y. Fukazawa, P. P. Singer and B. Altenkirk, Tetrahedron Lett., 1973, 14, 5045–5048 CrossRef.
-
(a) J. S. Liu, Y. I. Zhu, C. M. Yu, Y. U. Zhou, Y. Han, F. W. Wu and B. F. Qi, Can. J. Chem., 1986, 64, 837–839 CrossRef CAS;
(b) X. C. Tang, P. D. Sarno, K. Sugaya and E. Giacobini, J. Neurosci. Res., 1989, 24, 276 CrossRef CAS PubMed;
(c) Y. Hirasawa, H. Morita, M. Shiro and J. Kobayashi, Org. Lett., 2003, 5, 3991 CrossRef CAS PubMed.
-
(a) C. H. Heathcock, E. F. Kleinman and E. S. Binkley, J. Am. Chem. Soc., 1982, 104, 1054–1068 CrossRef CAS;
(b) M. S. Canham, J. D. France and E. L. Overman, J. Am. Chem. Soc., 2010, 132, 3991 CrossRef PubMed;
(c) D. L. Comins, C. A. Brooks, R. S. Al-awar and R. R. Goehring, Org. Lett., 1999, 1, 229–231 CrossRef CAS PubMed;
(d) C. F. Yen and C. C. Liao, Angew. Chem., Int. Ed., 2002, 41, 4090–4093 CrossRef CAS;
(e) J. Ramharter, H. Weinstabl and J. Mulzer, J. Am. Chem. Soc., 2010, 132, 14338–14339 CrossRef CAS PubMed.
- B. B. Snider and J. F. Grabowski, J. Org. Chem., 2007, 72, 1039–1042 CrossRef CAS PubMed.
- Y. Nishikawa, M. Kitajima, N. Kogure and H. Takayama, Tetrahedron, 2009, 1608–1617 CrossRef CAS.
- N. Veerasamy, E. C. Carlson, N. D. Collet, M. Saha and R. G. Carter, J. Org. Chem., 2013, 78, 4779–4800 CrossRef CAS PubMed.
- Price of (S)-piperidine ethanol (603€ per g) listed by ChemPur (11.01.2019).
Footnote |
† Electronic supplementary information (ESI) available. CCDC 1840179–1845388. For ESI and crystallographic data in CIF or other electronic format see DOI: 10.1039/c8sc05502e |
|
This journal is © The Royal Society of Chemistry 2019 |