DOI:
10.1039/C8SC04134B
(Edge Article)
Chem. Sci., 2019,
10, 688-693
Photoredox-mediated remote C(sp3)–H heteroarylation of free alcohols†
Received
17th September 2018
, Accepted 27th October 2018
First published on 30th October 2018
Abstract
We report an efficient and economical method for remote δ C(sp3)–H heteroarylation of free aliphatic alcohols using a hypervalent iodine PFBI-OH oxidant under photoredox catalysis. The reaction sequence involves in situ alcoholysis of PFBI-OH with alcohol, generation of an alkoxy radical intermediate by SET reduction, 1,5-HAT, and Minisci-type C–C bond formation. This method uses a slight excess of alcohols, can facilitate reaction at δ methyl and methylene positions, and has been successfully applied to modification of complex drug molecules.
Introduction
Selective C(sp3)–H functionalization of easily accessible aliphatic alcohols could streamline the synthesis of alcohols of complex structures. Radical-mediated reactions based on the 1,5-hydrogen atom transfer (1,5-HAT) of an alkoxyl radical intermediate have been widely used to functionalize the remote δ C(sp3)–H bond of alcohol derivatives even in complex molecular settings.1,2 While great success has been achieved using various pre-activated derivatives of alcohols,3 the corresponding reactions of free alcohols are more desirable but pose a significant challenge due to the strong O–H bond (∼105 kcal mol−1).4 A few exciting advances featuring new catalysis strategies have emerged recently (Scheme 1A). Notably, Zuo demonstrated δ C–H amination of primary alcohols with azodiformate using a cerium photocatalyst.5 Jiao reported a δ C–C bond forming reaction with sulfonyl oxime ether using a Ag(I) catalyst and K2S2O8 oxidant.6 Zhu reported a δ C–H Minisci-type heteroarylation of alcohols using a PhI(OTFA)2 (PIFA) oxidant and LED light irradiation.7 In Zhu's report, 5 equiv. of alcohols are typically required and the δ C–H bonds of alcohols are limited to unactivated secondary and tertiary C–H. Herein, we report an efficient and economical protocol for δ C(sp3)–H heteroarylation of free aliphatic alcohols with various N-heteroarenes using a perfluorinated hydroxybenziodoxole (PFBI-OH) oxidant under photoredox catalysis (Scheme 1C).
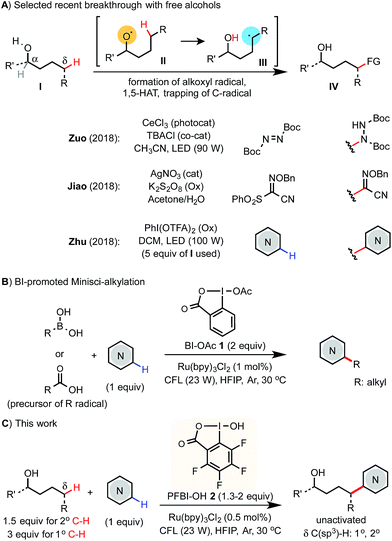 |
| Scheme 1 Radical-mediated remote C(sp3)–H functionalization of free alcohol. | |
Results and discussion
The Minisci reaction via radical pathways offers a convenient strategy to access complex heteroarenes from simple precursors.8,9 In our previous studies, we discovered that benziodoxole reagent BI-OAc 1, a cyclic hypervalent iodine(III), can promote C–H alkylation of various electron-deficient N-heteroarenes with alkyl boronic acid and alkyl carboxylic acid under photoredox catalysis (Scheme 1B).10,11 Interestingly, these two reactions proceed through different mechanisms.
The I-OAc bond of BI-OAc 1 can be activated by single electron transfer (SET) reduction by photoexcited Ru(II)* to form an acetate anion and BI radical (see fluorinated analog 35 in Scheme 4E), which reacts with boronic acid (RB(OH)2) to generate an alkyl radical intermediate following deboronation.10 In contrast, carboxylic acid (RCO2H) can undergo ester exchange with BI-OAc to form BI-O2CR, which can be activated by SET reduction to generate a BI anion and carboxyl radical, which provides an R radical following decarboxylation.11 Encouraged by the BI-OAc-mediated activation of carboxylic acid and a recent study by Chen on BI-mediated β C–C scission reactions of cycloalkyl alcohols under photoredox catalysis,12 we began to test whether common aliphatic alcohols can react with a suitable BI reagent to generate an alkoxyl radical, which can be trapped with N-heteroarenes to give useful products.13–15 As shown in Table 1, we were pleased to find that the reaction of pentanol 3 (1.5 equiv.) with 4-chloroquinoline 4 (1 equiv.) gave alkylation product 4a with an exclusive δ regioselectivity in 80% isolated yield using 1.35 equiv. of PFBI-OH 2 and 0.5 mol% of Ru(bpy)3Cl2 in hexafluoroisopropanol (HFIP) solvent at 30 °C under irradiation with a 23 W compact fluorescent lamp (CFL).16 In comparison, the use of BI-OAc 1, BI-OH 5, and other benziodoxoles bearing different aromatic substituents gave considerably lower yield (entries 2–4). Reaction with acyclic I(III) reagents including PhI(OTFA)27 or PhI(OAc)2 also proceeded in low yield under our optimized conditions (entries 5 and 7). Other important observations regarding the reaction optimization include the following: (1) no α-heteroarylation product 4a′ was obtained.17 Little butyl-substituted product 4a′′ (<2%) via the β-scission pathway of a pentoxy radical intermediate was obtained.18 (2) HFIP solvent is critical for obtaining high yield (entries 12 and 13). (3) While the use of 1 equiv. of alcohol 3 gave 42% yield of 4a, increasing the amount of 3 from 1.5 to 2 equiv. only slightly improved the yield (entries 15 and 16). (4) The reaction yield is sensitive to the amount of 2 used (entries 17 and 18). (5) A reaction conducted under a CFL in the absence of Ru(bpy)3Cl2 gave no product (entry 11). (6) Pre-stirring of 3 and 2 is unnecessary.
Table 1 Heteroarylation of 3 with 4
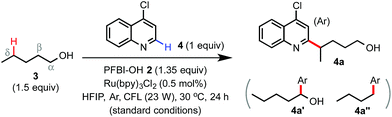
|
Entry |
Change from the standard conditions, reagents (equiv.) |
Yield of 4aa (%) |
NMR yield.
Isolated yield on a 0.4 mmol scale.
|
1 |
Standard conditions |
84 (80b) |
2 |
2 → Bl-OAc 1 |
25 |
3 |
2 → Bl-OH 5 |
3 |
4 |
2 → PFBl-OAc 6 |
30 |
5 |
2 → PhI(OTFA)27 |
4 |
6 |
2 → PhI(OTFA)27 (2.3), CFL → blue (LED, 100 W), HFIP → CH2Cl2, no Ru(bpy)3Cl2 |
3 |
7 |
2 → PhI(OAc)2 |
28 |
8 |
Ru(bpy)3Cl2 (0.5 → 1 mol%) |
61 |
9 |
Ru(bpy)3Cl2 → Ir(ppy)3 |
13 |
10 |
Ru(bpy)3Cl2 → [Ir(dF(CF3)ppy)2(dtbpy)]PF6 |
32 |
11 |
No Ru(bpy)3Cl2 |
<1 |
12 |
HFIP → CH2Cl2 |
3 |
13 |
HFIP → CF3CH2OH |
38 |
14 |
HFIP → HFIP/CH2Cl2 (1/5) |
8 |
15 |
3 (1.5 → 1) |
42 |
16 |
3 (1.5 → 2) |
86 |
17 |
2 (1.35 → 1.5) |
70 |
18 |
2 (1.35 → 1.2) |
63 |
|
We next examined the scope of alcohols and N-heteroarenes under optimized conditions (Schemes 2 and 3). In general, reaction of primary and secondary alcohols proceeded in good to excellent yield with exclusive δ selectivity. Alcohols bearing relatively weak benzylic (4f), allylic (4h), α C–H of ether (4j), and tertiary (4e) C(sp3)–H bonds also worked well. A wide range of functional groups including terminal alkene and alkyne (4h and 4g), Cbz (4k), azido (4i), ester (4l), aldehyde and ketone (13 and 10), halo (4l), and even pinacol boronate (15 and 26) groups were tolerated. Alcohols without any β-substituent usually gave little β-scission/alkylation side product. As shown in 4p, a small amount of 4-heptyl substituted byproduct (16%) was formed with 2-propylpentanol. While the radical functionalization of δ methylene C–H bonds of alcohols and their derivatives has been widely demonstrated in previous studies, we were pleased to find that our reaction at the more challenging δ methyl group also proceeded in good yield (see 4t, 4u, 23–25, 28 and 29) under slightly more forced conditions with 3 equiv. of alcohols and 2 equiv. of PFBI-OH 2. In comparison, N-heteroarylation at the methide position gave little product (see 4v) probably due to oxidation of the 3° C-radical to a 3° cation.19 As shown by 4s, tertiary alcohols gave little δ functionalization product.20
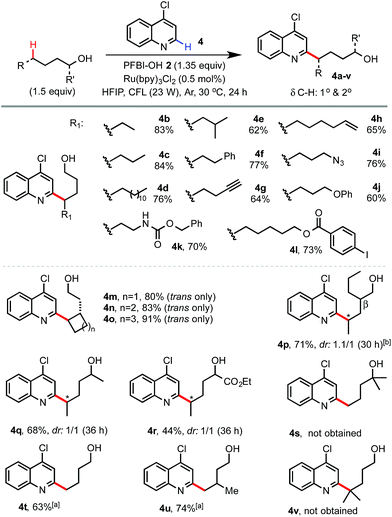 |
| Scheme 2 Scope of alcohols. Isolated yields on a 0.4 mmol scale. a3 equiv. of 1-alcohol and 2 equiv. of PFBI-OH were used, 36 h. b16% of 4-heptyl substituted side product was obtained. | |
As shown in Scheme 3, electron deficient N-heteroarenes showed good to excellent reactivity with various alcohols under the standard conditions. Chemoselectivity typical of Minisci reactions was observed for heteroarenes such as quinolines (8–11), isoquinolines (12–15), phenanthridine (16), phthalazine (17), quinoxaline (18), azaindole (19), and benzothiazole (20). Reaction of symmetric phthalazine (17) and pyridines (21 and 25) mainly gave mono-alkylation products. Reaction of complex N-heteroarene-containing drug molecules also worked well. For instance, reaction of famciclovir with 7-octyn-1-ol gave 27 in 53% yield. Reaction of quinoxyfen with 9-borylnonanol gave 26 in 67% yield. Reaction of tarocin A1 and camptothecin with 1-butanol gave 28 and 29 bearing a simple alkyl alcohol handle in good yield.
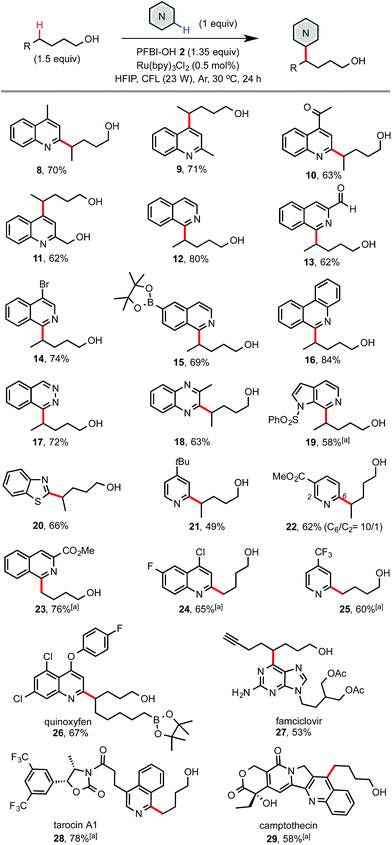 |
| Scheme 3 Scope of N-heteroarenes. Isolated yield on a 0.4 mmol scale. a3 equiv. of alcohol and 2 equiv. of PFBI-OH were used, 36 h. | |
Preliminary experiments were carried out to probe the reaction mechanism (Scheme 4). Similar to the ester exchange reaction of BI-OAc 1 with carboxylic acids, PFBI-OH 2 can readily undergo alcoholysis with 1-butanol in HFIP at rt to give PFBI-OBu 30 (Scheme 4A). Reaction of 4-chloroquinoline 4 with 30 under similar photoredox conditions gave product 4t in comparable yield as with using PFBI-OH 2 and BuOH (63% in Scheme 2). Stern–Volmer (SV) fluorescence quenching experiments of Ru(bpy)3Cl2 showed that the Ru(II)* excited state is quenched by PFBI-OBu 30, but not by 4-chloroquinoline 4 (Scheme 4C). In comparison, quenching of Ru(II)* by PFBI-OH 2 also occurs but with a much smaller SV quenching constant (KSV) than when using 30 (83 vs. 382), indicating a weaker oxidative quenching ability of 2.21 As shown in Scheme 4D, reaction of 4 with isobutanol 31, bearing a β substituent but lacking δ C–H bonds, gave product 32 in high yield. This indicated that the corresponding isobutoxy radical is generated and then undergoes β-scission to form an isopropyl radical.18 Based on these pieces of evidence, we propose that the reaction of alcohol 33 starts with alcoholysis with PFBI-OH 2 to form 34 (Scheme 4E). 34 can be activated by SET reduction by Ru(II)* to give Bz′O anion 36 and alkoxyl radical 37.21 The fluoro substitution on benziodoxole probably makes the iodo center of PFBI-OH 2 more electrophilic for alcoholysis and makes 34 more easily reducible by SET.22 1,5-HAT reaction of 37 gives C-radical 38, which reacts with N-heteroarenes to give 41. SET oxidation of 41 by Ru(III) gives the alkylated product 42 and regenerates Ru(II). Alternatively, 41 could be oxidized by 34 to form 42 and 37, propagating a radical chain reaction. In principle, alkoxyl radical 37 can also undergo β-scission to give 39 and shortened alkyl radical 40. In our system, we found that this competing pathway was negligible for alcohols bearing no β substituent (see 40, R′′ = H).23
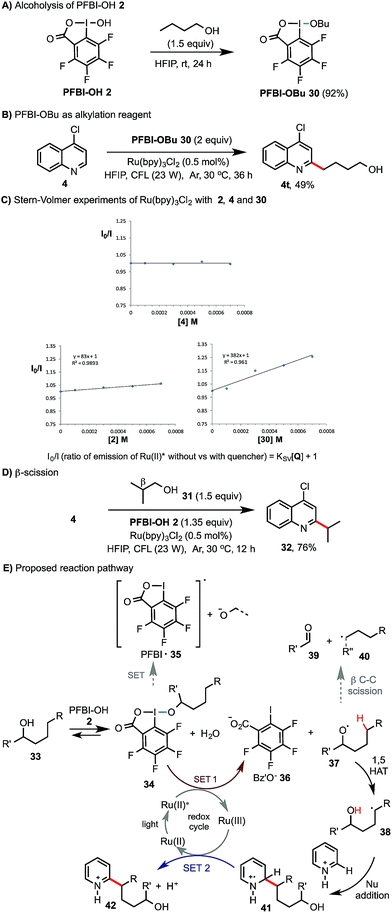 |
| Scheme 4 Mechanistic study. | |
Conclusions
In summary, we have developed an efficient and economical method for remote C(sp3)–H heteroarylation of free aliphatic alcohols under mild conditions using photoredox catalysis. The reaction sequence involves facile in situ alcoholysis of PFBI-OH with alcohol, generation of an alkoxy radical intermediate by SET reduction, 1,5-HAT, and Minisci-type C–C bond formation. The reaction shows broad substrate scope for both alcohols and N-heteroarenes. Importantly, this method uses a slight excess of alcohols, can facilitate reaction at the δ methyl and methylene positions, and has been successfully applied to modification of complex drug molecules. The high electrophilicity of PFBI-OH is critical to achieving high efficiency without the use of a large excess of alcohols. Remote C–H functionalization reactions of other types of substrate using a similar strategy are currently under investigation.
Conflicts of interest
There are no conflicts to declare.
Acknowledgements
We gratefully thank the National Natural Science Foundation of China (21672105, 21725204, and 91753124), Natural Science Foundation of Tianjin (18JCZDJC32800), and Laviana for financial support of this work.
Notes and references
- For selected recent reviews on alkoxyl radical-mediated reactions, see:
(a) L. M. Stateman, K. M. Nakafuku and D. A. Nagib, Synthesis, 2018, 1569 CAS;
(b) J.-J. Guo, A. Hu and Z. Zuo, Tetrahedron Lett., 2018, 59, 2103 CrossRef CAS;
(c) K. Jia and Y. Chen, Chem. Commun., 2018, 54, 6105 RSC.
- For selected reviews on radical functionalization of sp3 C–H bonds, see:
(a) T. Newhouse and P. S. Baran, Angew. Chem., Int. Ed., 2011, 50, 3362 CrossRef CAS PubMed;
(b) M. C. White, Science, 2012, 335, 807 CrossRef CAS PubMed;
(c) T. Dohi and Y. Kita, ChemCatChem, 2014, 6, 76 CrossRef CAS;
(d) M. Yan, J. C. Lo, J. T. Edwards and P. S. Baran, J. Am. Chem. Soc., 2016, 138, 12692 CrossRef CAS PubMed;
(e) D. Ravelli, S. Protti and M. Fagnoni, Chem. Rev., 2016, 116, 9850 CrossRef CAS PubMed;
(f) H. Yi, G. Zhang, H. Wang, Z. Huang, J. Wang, A. K. Singh and A. Lei, Chem. Rev., 2017, 117, 9016 CrossRef CAS PubMed.
- For selected examples of remote C(sp3)–H functionalization of alcohols using pre-activated derivatives, see:
(a) D. H. R. Barton, J. M. Beaton, L. E. Celler and M. M. Pechet, J. Am. Chem. Soc., 1960, 82, 2640 CrossRef CAS;
(b) K. Heusler and J. Kalvoda, Angew. Chem., Int. Ed., 1964, 3, 525 CrossRef;
(c) J. I. Concepción, C. G. Francisco, R. Hernández, J. A. Salazar and E. Suárez, Tetrahedron Lett., 1984, 25, 1953 CrossRef;
(d) A. L. J. Beckwith and B. P. Hay, J. Am. Chem. Soc., 1988, 110, 4415 CrossRef CAS;
(e) R. Kundu and Z. T. Ball, Org. Lett., 2010, 12, 2460 CrossRef CAS PubMed;
(f) J. Zhang, Y. Li, F. Zhang, C. Hu and Y. Chen, Angew. Chem., Int. Ed., 2016, 55, 1872 CrossRef CAS PubMed;
(g) C. Wang, K. Harms and E. Meggers, Angew. Chem., Int. Ed., 2016, 55, 13495 CrossRef CAS PubMed.
-
Y.-R. Luo, Comprehensive Handbook of Chemical Bond Energies, CRC Press, Boca, Raton, FL, 2007 Search PubMed.
- A. Hu, J.-J. Guo, H. Pan, H. Tang, Z. Gao and Z. Zuo, J. Am. Chem. Soc., 2018, 140, 1612 CrossRef CAS PubMed.
- Y. Zhu, K. Huang, J. Pan, X. Qiu, X. Luo, Q. Qin, J. Wei, X. Wen, L. Zhang and N. Jiao, Nat. Commun., 2018, 9, 2625 CrossRef PubMed.
- During the preparation of this manuscript, the following work by Zhu was published online. X. Wu, H. Zhang, N. Tang, Z. Wu, D. Wang, M. Ji, Y. Xu, M. Wang and C. Zhu, Nat. Commun., 2018, 9, 3343 CrossRef PubMed.
-
(a) F. Minisci, E. Vismara and F. Fontana, Heterocycles, 1989, 28, 489 CrossRef CAS;
(b) M. A. J. Duncton, Med. Chem. Commun., 2011, 2, 1135 RSC.
- For selected recent examples of Minisci alkylation of N-heteroarenes, see:
(a) Y. Fujiwara, V. Domingo, I. B. Seiple, R. Gianatassio, M. D. Bel and P. S. Baran, J. Am. Chem. Soc., 2011, 133, 3292 CrossRef CAS PubMed;
(b) G. A. Molander, V. Colombel and V. A. Braz, Org. Lett., 2011, 13, 1852 CrossRef CAS PubMed;
(c) Y. Fujiwara, J. A. Dixon, F. O'Hara, E. D. Funder, D. D. Dixon, R. A. Rodriguez, R. D. Baxter, B. Herle, N. Sach, M. R. Collins, Y. Ishihara and P. S. Baran, Nature, 2012, 492, 95 CrossRef CAS PubMed;
(d) A. P. Antonchick and L. Burgmann, Angew. Chem., Int. Ed., 2013, 52, 3267 CrossRef CAS PubMed;
(e) J. Jin and D. W. C. MacMillan, Nature, 2015, 525, 87 CrossRef CAS PubMed;
(f) L. Fang, L. Chen, J. Yu and L. Wang, Eur. J. Org. Chem., 2015, 1910 CrossRef CAS;
(g) D.-C. Wang, R. Xia, M.-S. Xie, G.-R. Qu and H.-M. Guo, Org. Biomol. Chem., 2016, 14, 4189 RSC;
(h) M. C. Quattrini, S. Fujii, K. Yamada, T. Fukuyama, D. Ravelli, M. Fagnoni and I. Ryu, Chem. Commun., 2017, 53, 2335 RSC.
- G.-X. Li, C. A. Morales-Rivera, Y. Wang, F. Gao, G. He, P. Liu and G. Chen, Chem. Sci., 2016, 7, 6407 RSC.
- J. Wang, G.-X. Li, G. He and G. Chen, Asian J. Org. Chem., 2018, 7, 1307 CrossRef CAS.
-
(a) K. Jia, F. Zhang, H. Huang and Y. Chen, J. Am. Chem. Soc., 2016, 138, 1514 CrossRef CAS PubMed;
(b) K. Jia, Y. Pan and Y. Chen, Angew. Chem., Int. Ed., 2017, 56, 2478 CrossRef CAS PubMed.
- For selected reviews on photoredox-mediated organic reactions, see:
(a) J. M. R. Narayanam and C. R. J. Stephenson, Chem. Soc. Rev., 2011, 40, 102 RSC;
(b) C. K. Prier, D. A. Rankic and D. W. C. MacMillan, Chem. Rev., 2013, 113, 5322 CrossRef CAS PubMed;
(c) M. H. Shaw, J. Twilton and D. W. C. MacMillan, J. Org. Chem., 2016, 81, 6898 CrossRef CAS PubMed.
- For selected reviews on hypervalent iodine chemistry, see:
(a) T. Wirth, Angew. Chem., Int. Ed., 2005, 44, 3656 CrossRef CAS PubMed;
(b) A. Yoshimura and V. V. Zhdankin, Chem. Rev., 2016, 116, 3328 CrossRef CAS PubMed;
(c) Y. Li, D. P. Hari, M. V. Vita and J. Waser, Angew. Chem., Int. Ed., 2016, 55, 4436 CrossRef CAS PubMed.
- For selected examples of using benziodoxole reagents in visible light-mediated reactions, see:
(a) H. Huang, G. Zhang, L. Gong, S. Zhang and Y. Chen, J. Am. Chem. Soc., 2014, 136, 2280 CrossRef CAS PubMed;
(b) Q.-Q. Zhou, W. Guo, W. Ding, X. Wu, X. Chen, L.-Q. Lu and W.-J. Xiao, Angew. Chem., Int. Ed., 2015, 54, 11196 CrossRef CAS PubMed;
(c) F. Le Vaillant, T. Courant and J. Waser, Angew. Chem., Int. Ed., 2015, 54, 11200 CrossRef CAS PubMed;
(d) Y. Wang, G.-X. Li, G. Yang, G. He and G. Chen, Chem. Sci., 2016, 7, 2679 RSC.
- For the original report of PFBI-OH 2, see: R. D. Richardson, J. M. Zayed, S. Altermann, D. Smith and T. Wirth, Angew. Chem., Int. Ed., 2007, 46, 6529 CrossRef CAS PubMed . PFBl-OH can be readily prepared on the gram scale in 2 steps from a cheap precursor (2,3,4,5-tetrafluorobenzoic acid, <$250 per kg).
- Previously reported Minisci alkylation reactions with alcohols mainly take place on the weak α-C(sp3)–H bond of alcohols; for selected examples, see:
(a) F. Minisci, A. Citterio and E. Vismara, Tetrahedron, 1985, 41, 4157 CrossRef CAS;
(b) C. A. Correia, L. Yang and C.-J. Li, Org. Lett., 2011, 13, 4581 CrossRef CAS PubMed;
(c) T. D. Neubert, Y. Schmidt, E. Conroy and D. Stamos, Org. Lett., 2015, 17, 2362 CrossRef CAS PubMed.
- For selected examples of radical reactions facilitated by β-C–C scission of alcohols, see:
(a) R. Ren, Z. Wu, Y. Xu and C. Zhu, Angew. Chem., Int. Ed., 2016, 55, 2866 CrossRef CAS PubMed;
(b) D. D. Bume, C. R. Pitts and T. Lectka, Eur. J. Org. Chem., 2016, 26 CrossRef CAS;
(c) A. Nikolaev, C. Y. Legault, M. Zhang and A. Orellana, Org. Lett., 2018, 20, 796 CrossRef CAS PubMed;
(d) X. Wu, M. Wang, L. Huan, D. Wang, J. Wang and C. Zhu, Angew. Chem., Int. Ed., 2018, 57, 1640 CrossRef CAS PubMed.
- G.-X. Li, C. A. Morales-Rivera, F. Gao, Y. Wang, G. He, P. Liu and G. Chen, Chem. Sci., 2017, 8, 7180 RSC.
- Alcoholysis of PFBI-OH 2 with tertiary alcohol is probably hampered by sterics.
- HFIP is a weak acid. We suspect that proton-coupled electron transfer may be involved in SET reduction of 34, forming Bz′OH and 37. In comparison, formation of PFBI radical 35via SET reduction of 34 is possibly less favoured due to the weaker basicity of the alkoxyl O on 34. PFBI-OH 2 could also be activated by SET reduction to generate PFBI radical 35. The use of slightly more alcohol than PFBI-OH 2 (1.5 vs. 1.35 equiv.) might help suppress the formation of 35.
- Our previous study showed that PFBI radical 35 is more electrophilic for H-abstraction than the corresponding plain BI radical (ref. 19).
- Even reactions with butanol gave little propyl-substituted side products (see 4t). As seen with isobutanol 31, some β-substituted alcohols can readily undergo β-scission to form more stabilized C-radical 40, which can be effectively engaged in the subsequent Minisci reaction. Detailed study of this transformation will be reported in a future paper.
Footnote |
† Electronic supplementary information (ESI) available. See DOI: 10.1039/c8sc04134b |
|
This journal is © The Royal Society of Chemistry 2019 |
Click here to see how this site uses Cookies. View our privacy policy here.