DOI:
10.1039/C9RA08417G
(Paper)
RSC Adv., 2019,
9, 37101-37108
A Zn(II) metal–organic framework based on bimetallic paddle wheels as a luminescence indicator for carcinogenic organic pollutants: phthalate esters†
Received
15th October 2019
, Accepted 6th November 2019
First published on 13th November 2019
Abstract
Based on the multifunctional ligand 3-(1H-1,2,4-triazol-1-yl)isophthalic acid (H2TIA), a three-dimensional coordination polymer, namely {[Zn(TIA)]·DMA}n (Zn-1) was synthesized solvothermally. Single-crystal X-ray diffraction analyses confirmed that Zn-1 is a 3D framework composed of binuclear Zn2 paddle wheels with one-dimensional channels long the a direction. Further topological analyses revealed that MOF Zn-1 existed as a (3,6)-connected rtl binodal net {4·62}2{42·610·83}. Furthermore, the luminescence explorations indicate that complex Zn-1 is the first MOF for luminescent probing of phthalate esters (carcinogenic organic pollutants) with a high quenching-efficiency constant and low fluorescence-detection limit.
Introduction
Owning to their appealing architectures and potential application value in catalysis,1 gas storage/separation,2 luminescence,3 and so on,4 metal–organic frameworks (MOFs), especially, those with large cavities or channels have attracted tremendous attention. In particular, a great deal of research has focused on exploring their applications in luminescence sensing, which in turn can be used to detect environmental pollutants selectively and sensitively. Distinguished from conventional carbon-based materials and porous zeolites, the ordered structure, the aperture and shape of the pore, along with the functionalities of MOFs are controllable.5 For example, the structure of the MOFs can be devised and regulated through reasonably selecting and adjusting of organic linkers and metal salts or clusters. Even so, the initiators characteristics and properties would heavily take impact on the final structures of coordination polymer. Besides, chemical reaction conditions, such as solvent, pH value, temperature, counter ions and so on, would also create impress influence on their final structure.6 Comparatively speaking, the rational selection of an appropriate organic ligand with a certain spatial configuration and active sites is considered to be the most effective strategy for constructing MOFs with the anticipated topological structures and desirable functions.
Due to the tunable channel size, large surface area and functional sites, MOFs always display amazing interaction properties between the coordination polymers and the analytes, and that makes MOFs the very sensible sensors of much more excellent properties than other luminescent materials.7 In particular, MOFs which have d10 or 4f metal centers always exhibit fascinating luminescent properties, they are considered as potential chemosensors, such as selective detectors for anions,3f,8 heavy metal cations,9 vapours,10 toxic organic molecules,3d,9d,9e,11 and biomolecules.3e,12 While, an unmet challenge is to design MOFs for quick recognizing persistent toxic organics, especially for phthalate esters (carcinogenic organic pollutants), which are believed having severe impacts on environmental protection and public health.
Phthalate esters (PAEs) are a class of organic chemical reagents, which were already and are being extensively used in commercial and industrial plastics additives. As one of the most produced and consumed chemicals in the world, PAEs undoubtedly exist in atmosphere,13 water,14 soil,15 sediment.16 And more seriously, their metabolites were reported already been found in human urine and serum.17 Recent researching results have shown that PAEs also may have potential carcinogenesis effects on human and animals.18 In addition, studies have also shown a correlation between PAE levels and precocious breast development, long-term exposure to PAE for women can lead to endometriosis and gestational duration.19 In addition, Gao's research group had also found that PAEs may cause central nervous system depression and obvious renal injury.20 Therefore, PAEs have posed great potential and obvious threaten to human and wildlife reproduction. Heavy efforts have already been taken to diminish and erase the pollution and ruin from PAEs. Precisely and rapid detection of PAEs, especially of trace concentration and amount, was believed the first and key step to success.
An effective method was processed for the controllable synthesis of MOFs as phthalate esters sensor, in which, aromatic organic molecules with non-bonded functional ligand sites were selected as the precursors. The aromatic ring of the precursors has fluorescent properties, and the nonbonded functional sites will interact with d10 or 4f metal centers which will enhance fluorescence properties. Lately, 1,2,4-triazole and its derivatives with several N-donor atoms, as well as aromatic polyacids with several O-donor atoms are widely used to construct novel MOFs. Corresponding, a great number of coordination polymers with novel architectures, higher stabilities and peculiar functions have been reported successively.11a,21 In this context, a multi-functional organic linker 3-(1H-1,2,4-triazol-1-yl)isophthalic acid (H2TIA), which contains long π–π conjugate chain was selected as the MOFs' precursor, for it combines the advantages of the two kinds of functional groups (triazole groups and aromatic carboxylic acid groups). Besides, d10 metal centers such as Zn2+, Ag+, and Cd2+, were usually adopted in synthesizing of luminescent MOFs, because these cations can adjust the emission wavelength and intensity of organic linkers. A new MOF {[Zn(TIA)]·DMA}n (Zn-1) with one-dimensional channels was successfully obtained from H2TIA and Zn(NO3)2, which showed as a 3D framework with rtl topology. Fluorescence recognition experiments demonstrate that micro-porous framework of Zn-1 has high efficiency in detecting phthalate esters under ambient conditions through “turn-off” luminescent detection (Scheme 1).
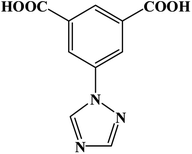 |
| Scheme 1 The structure of the ligand 3-(1H-1,2,4-triazol-1-yl)isophthalic acid (H2TIA). | |
Experimental details
Materials and physical measurement
Most of the starting materials and solvents were commercially available and used without further treatment, except that the solvent dimethyl sulfoxide (DMSO) was treated by 4A molecular sieve and distilled under vacuum. 3-(1H-1,2,4-triazol-1-yl)isophthalic acid (H2TIA) was synthesized according to the literature methods.22 Elemental analyses for C, H, and N were taken on a PerkinElmer elemental analyzer. Infrared spectra were determined on a Bruker TENOR 27 spectrometer in the region of 4000–400 cm−1. Powder X-ray diffraction patterns (PXRD) were carried out on a D/Max-2500 X-ray diffractometer with Cu-Kα radiation (λ = 1.5406 Å) at room temperature. UV-vis spectra were collected on a UV-2600 spectrophotometer. Thermogravimetric test (TG) was measured on a NETZSCH TG 209 instrument under N2 atmosphere with the heating rate controlled at 10 °C min−1. Emission/excitation spectra were recorded on F97pro fluorescence spectrometer. Gas adsorption isotherms were performed by a volumetric method on a Micromeritics ASAP 2020HD88 surface area and pore analyzer.
Preparation of {[Zn(TIA)]·DMA}n (Zn-1)
H2TIA (17.5 mg, 0.075 mmol), Zn(NO3)2·4H2O (30 mg, 0.1 mmol) and 6 mL CH3OH–DMA (2
:
4, in v/v) were added in turn into a 15 mL Teflon-lined stainless steel vessel, and then the mixture was heated at 80 °C for three days. After that, the autoclave was cooled to room temperature at a rate of 1.2 °C h−1. Colorless rectangular crystals Zn-1 (20.54 mg) were obtained (yield 71% based on H2TIA). Elemental analysis for C14H14N4O5Zn (383.66): C 42.42, H 3.68, N 14.61%. Found: C 42.68, H 3.58, N 14.24%. IR (KBr pellet): 3503 (b), 3413 (b), 2360 (s), 1654 (s), 1623 (s), 1523 (w), 1480 (w), 1486 (w), 1374 (m), 1288 (s), 1159 (m), 1084 (m), 1055 (w), 858 (m), 720 (m), 546 (m) cm−1.
Luminescence sensing experiments
The finely pressed powder samples of MOF Zn-1 (0.2 mg, optimum dosage of Zn-1 was determined by fluorescence test, Fig. S6†) were added in organic solvents (5 mL) or water. The mixture was firstly ultrasonicated for half an hour to form a stable suspension and then used for fluorescence experiment. The selected solvents are esters (ethyl acetate (EAC), diethyl phthalate ester (DEPAE), di-n-butyl phthalate ester (DBPAE), di-n-octyl phthalate ester (DOPAE)), alcohols (methanol, ethanol), amides (N,N-dimethyl formamide (DMF), N-methyl-2-pyrrolidone (NMP), N,N-dimethylacetamide (DMA)), methyl chloride (CH2Cl2, CHCl3), nitriles (CH3CN), dimethyl sulfoxide (DMSO), cyclic ether (tetrahydrofuran (THF)), aromatic hydrocarbon (toluene) and H2O. For the fluorescence quenching experiments to carcinogenic organic pollutants, phthalate esters with different volumes (μL) were added into the mixture of standard Zn-1 (0.2 mg) emulsion in DMF (5 mL).
Results and discussion
Crystal structure descriptions
Crystal structure of {[Zn(TIA)]·DMA}n (Zn-1). Complex Zn-1 crystallizes in the monoclinic P21/c space group (Table S1, ESI†). In Zn-1, each asymmetric unit is composed by a Zn2+ ion, one TIA2− ligand and a free DMA. As displayed in Fig. 1a, the central Zn2+ ion existed in a rare penta-coordinating mode finished by one N and four O donors from five different TIA2− ligands to form a tetragonal pyramidal polyhedron configuration. Two Zn2+ ions are tetra-bridged by four μ2-η1:η1 carboxylate groups which come from four different TIA2− ligands, to form a binuclear core with the Zn–Zn distance of 2.967(2) Å (Fig. 1a). The binuclear coordinating units are further connected by carboxylate groups and triazole N atoms of TIA2− ligands to form a 3D framework (Fig. 1b), in which one-dimensional channels exist along a direction. In Zn-1, the triazolyl ring, the two carboxylate groups in the same TIA2− ligand are not completely coplanar with the central phenyl ring.
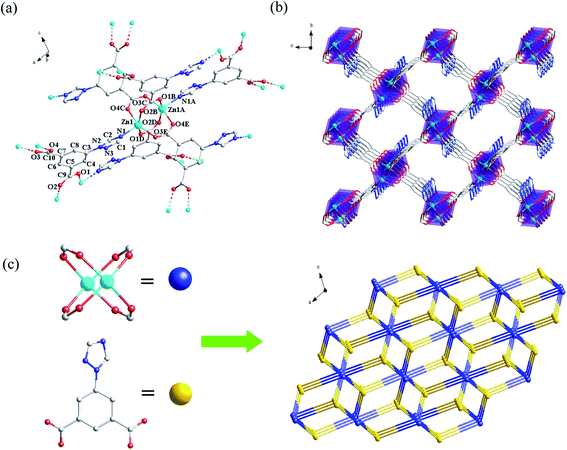 |
| Fig. 1 (a) The coordination environment of ZnII ions in compound Zn-1. Hydrogen atoms were omitted for clarity. Symmetry operator: A = 1 − x, −0.5 + y, 1.5 − z; B = x, y, 1 + z; C = 1 + x, 1.5 − y, 0.5 + z; D = 2 − x, 1 − y, 1 − z; E = 2 − x, 1 − y, 2 − z. (b) The 3D framework of Zn-1 viewed along a direction. (c) Topology network of the framework Zn-1. | |
In the 3D framework, if each Zn2 binuclear unit is treated as a six-connected node and each TIA2− is regarded as a three-connected node, Zn-1 can be regarded as a (3,6)-connected rtl binodal net, with the short (Schläfli) vertex symbol of {4·62}2{42·610·83}, which is shown in Fig. 1c. By using the program of PLATON, the ratio of the accessible porous volume (utilizable) in every unit cell is calculated as 53.5%.
PXRD, thermal stability and sorption properties
As shown in Fig. S2 (ESI†), powder X-ray diffraction patterns for compound Zn-1 were carried out to confirm the phase purity of crystalline materials. The main peak positions of the experiment data coincide with the corresponding simulated ones, suggesting the good phase purity of the bulk crystals Zn-1.
For the purpose of detecting the thermal stability of the framework in Zn-1, thermogravimetric analyses (TGA) was performed at the heating rated of 10 °C min−1 under a N2 atmosphere in the temperature range of 30–800 °C. The TGA curve (Fig. S4†) of Zn-1 displays as a two-step weight loss, the first step weight loss of 22.93% before 220 °C (calcd 22.68%) contributed by the loss of free DMA molecule in the lattice. The second step weight loss attributes to the collapse of the 3D framework at 366 °C, which demonstrates the framework is relatively stable.
The permanent porosity of MOF Zn-1 was further detected by the N2 sorption isotherm, which was carried out at 77 K. As displayed in Fig. S5,† it was a type-I isotherm with a BET surface area of 31.46 m2 g−1 (Langmuir surface area 47.83 m2 g−1).
Photoluminescent properties of Zn-1 and H2TIA in solid state
Organic compounds which contain wide π–π conjugated systems and aromatic rings, along with their relevant metal–organic coordination polymers, have aroused extensive interest of chemists owning to their distinctive luminescent properties and latent applications in fluorescent devices, such as light-emitting diodes (LEDs). The architecture of novel coordination polymers by reasonable selection of conjugated organic connectors and transition metal centers (such as Zn2+, Cd2+, and Ag+) may be one of the most efficacious methods to obtain new kinds of luminescent materials, because of the capability to adjust the emission wavelength and intensity of organic linkers. Herein, the photo-luminescent properties of the aromatic H2TIA and its relevant complex Zn-1 in the solid state have been investigated.
At ambient temperature, the organic linker H2TIA exhibits a broad emission band with the maximum at 350 nm (λex = 290 nm), which is assigned as π → π* transitions caused by the large conjugated aromatic triazole and phenyl rings (Fig. 2).23 On comparison, Zn-1 shows an intense fluorescence emission band centered at 366 nm (λex = 290 nm), which is contributed by the intraligand emission. Compared with organic ligand H2TIA, the enhancement of fluorescence intensity is attributed to that the rigidity of the ligand significantly increases by metal–ligand coordination, and the non-radiative decay between intraligand transition states decreases accordingly. For coordination polymers, the emission band based on organic ligand is preferable, since the band gaps of host material can be altered along with exchanged guest molecules diffusing into the channels based on host–guest interaction, thus resulting in different fluorescent response on the basis of the change of luminescent intensity or position.24 Consequently, MOF Zn-1 may be used as a fluorescent sensor owning to its structural particularities which contain 1D channels decorated by a number of open coordinating locations. In addition, MOF Zn-1 is insoluble in general organic solvents, therefore its selective sensing ability was investigated for small molecular organic solvents as well as the firmness of the framework and the durability of the micropore.
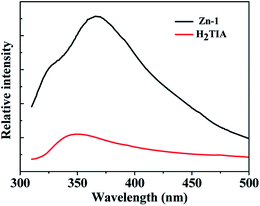 |
| Fig. 2 Fluorescence spectra for H2TIA and Zn-1 in solid-state at the excitation wavelength of 290 nm. | |
Luminescent sensing properties of Zn-1 to phthalate esters
Grounded samples of MOF Zn-1 (2 mg) were immersed in selected solvents (5 mL), which included CH3OH, EtOH, EAC, phthalate esters (DEPAE, DBPAE, DOPAE), DMF, DMA, CH2Cl2, CHCl3, CH3CN, DMSO, toluene, THF, and H2O. As exhibited in Fig. 3, the testing results showed that the fluorescence intensities of complex Zn-1 were solvent dependent. For phthalate esters (DEPAE, DBPAE, DOPAE), obvious luminescence quenching phenomena to Zn-1 were observed, with the highest quenching efficiencies as 99.96%, 99.98%, and 99.97%, respectively. However, other solvents exerted a certain degree of attenuation to the luminescence intensities of Zn-1. These results demonstrate that Zn-1 may be potential fluorescence sensors for phthalate esters (PAEs, a class of carcinogenic organic pollutants).
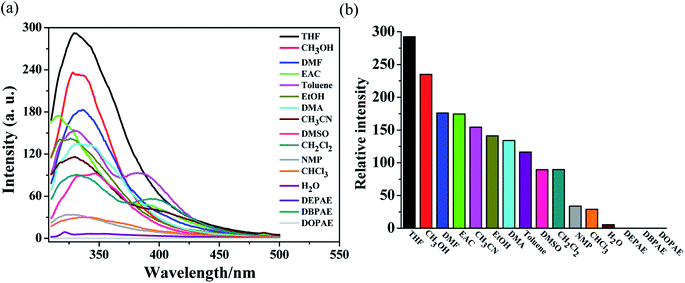 |
| Fig. 3 (a) Emission spectra of Zn-1 dispersed in different organic solvents when excited at 290 nm. (b) Relative luminescence intensities in various solvents at the wavelength of 325 nm. | |
For the purpose of further investigating the sensing abilities of polymer Zn-1 to PAEs series, the selective sensing tests were conducted by using different amount of PAEs in DMF solution (Fig. 4–6). Suspensions of Zn-1 in DMF solution were prepared by the same method as above mentioned except by gradually increasing the amount of phthalate esters. It is obvious that the fluorescence intensity of DEPAE@Zn-1 weakened dramatically with the increase of diethyl phthalate ester (DEPAE) content and became a half at a low diethyl phthalate ester content of 0.04 vol% (2 mM), and almost completely disappeared at a concentration of 0.4 vol% (20 mM). The quenching percentage of Zn-1 toward diethyl phthalate ester was evaluated to be 99.1%, thus MOF Zn-1 can be seen as a good candidate for highly selectively sensing the DEPAE.
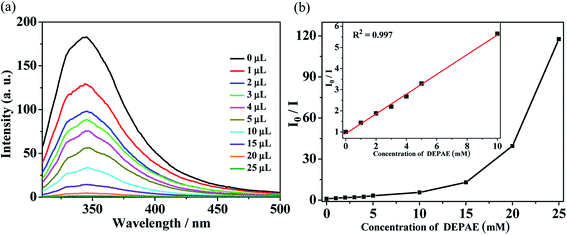 |
| Fig. 4 (a) Luminescence spectra and (b) SV curve for Zn-1 by gradual addition of different volume (μL) of pure diethyl phthalate ester (DEPAE) in DMF solution. | |
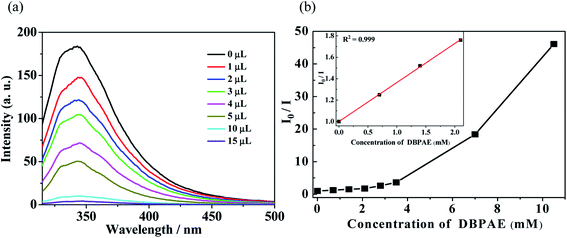 |
| Fig. 5 (a) Luminescence spectra and (b) SV curve for Zn-1 by gradual addition of different volume (μL) of pure di-n-butyl phthalate ester (DBPAE) in DMF solution. | |
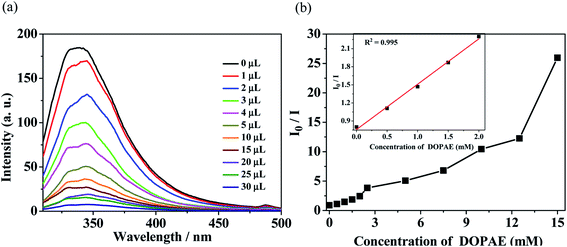 |
| Fig. 6 (a) Luminescence spectra and (b) SV curve for Zn-1 by gradual addition of different volume (μL) of pure di-n-octyl phthalate ester (DOPAE) in DMF solution. | |
The Stern–Volmer plots of relative luminescent intensity (I0/I) versus the concentration of DEPAE were used to quantify the quenching efficiency, which are exhibited in Fig. 4b. The quenching efficiency can be fitted through the equation: I0/I = 1 + KSV[DEPAE]. I0 and I are the luminescence intensity of Zn-1 before and after addition of the analytes DEPAE, [DEPAE] denotes the molar concentration of DEPAE, and KSV is the quenching rate constant. As shown in Fig. 4b, I0/I versus DEPAE concentration plots were almost linear under the condition of low concentration, the quenching constant KSV can be determined as 4.67 × 102 M−1 (insets of Fig. 4b). Consequently, it can be concluded that DEPAE can be effectively recognized by Zn-1 in a very low concentration range.
In order to probe the fluorescent quenching characteristics of other common PAE series such as DBPAE, DOPAE, a series of experiments were conducted as follows: the samples of Zn-1 (2 mg) was ground and immersed in 5 mL DMF solution and ultrasonicated 20 min to form stable suspensions, and then DBPAE and DOPAE were separately and gradually added to the standard suspensions. The curve of intensity versus the concentration of DBPAE and DOPAE are shown in Fig. 5 and 6, respectively. For DBPAE, when the concentration was 2.1 mM, the fluorescence intensity has almost halved, with the quenching efficiency of 43%. At the concentration of 10.5 mM, the luminescence quenching efficiency was as high as 98% (Fig. 5). DOPAE displayed similar property with a quenching efficiency of 47% (at 1.87 mM) and 96% (at 26 mM) (Fig. 6).
What's more, the quenching mechanism of coordination polymer to PAEs was further studied, the UV-absorption spectra of Zn-1 and PAEs in ethanol solution were determined in the range of 200–450 nm. As depicted in Fig. S7,† the excitation peak of Zn-1 overlapped with the absorption band of PAE's, which indicated the competition of absorption of the light source energy between ligand H2TIA and PAEs. The energy absorbed by ligand H2TIA was transferred to PAEs, which resulted in emission quenching of Zn-1 by means of an excitation energy transfer mechanism.
To measure the quality of a chemosensor, in addition to taking into account the stability, selectivity and sensitivity, the reusability should also be considered as an important factor. Therefore, the tests for the recyclability of Zn-1 to PAEs (DEPAE, DBPAE, DOPAE) were carried out (Fig. 7). The luminescence intensities of Zn-1 could almost restore to their initial level after @PAE-Zn-1 being washed with DMF solvent for a few times. In addition, powder X-ray diffraction experiments further confirmed that the framework of MOF Zn-1 remained unchanged after fluorescence recycling experiments (Fig. S3†). Special stability of the framework and recyclability of MOF Zn-1 indicates the potential applicability as a fluorescent sensor for PAEs organic pollutants.
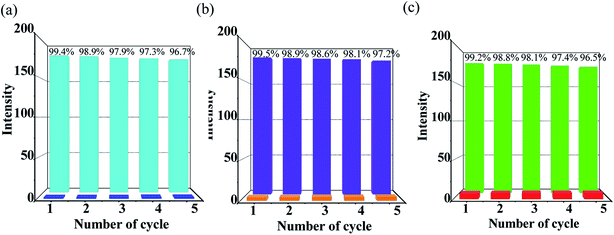 |
| Fig. 7 Cyclic response of luminescence intensities at 345 nm of Zn-MOF (Zn-1) for detecting of (a) DEPAE, (b) DBPAE and (c) DOPAE, intensity percentage = I/I0 × 100%. | |
According to the reported results,8c,9e,25 these multifunctional ligands which contain long π–π conjugated systems have a wide application prospect in fluorescent probing field. Such as the 2D layer complexes {[Cd2L2(H2O)4]·H2O}n and {[Zn2L2(H2O)4]·H2O}n and 3D polymer [Cd3L3(DMF)2]n all exhibited highly selective and sensitive chemosensors for CrVI-anions in aqueous medium. In our previous work, we found MOF [Cd0.5(TBC)]n as a bifunctional luminescence sensor for benzaldehyde and Fe2+ ion. In the near future, we will continue to explore the application of these multifunctional ligands in fluorescent probing.
Conclusion
In conclusion, one porous Zn-MOF (Zn-1) was successfully synthesized by using a multifunctional organic linker H2TIA as the building block. Zn-1 exists as a 3,6-connected rtl 3D framework with 1D channels, which was composed by binuclear Zn2 paddle wheels. In addition, the well-defined correlation between the luminescence intensity of Zn-1 and the concentration of phthalate esters provides a reliable relationship that makes MOF Zn-1 as a low-cost and user-friendly luminescence sensor to determine the concentration of different target species. As far as I know, MOF Zn-1 is the first specialized and efficient luminescence sensor for phthalate esters (carcinogenic organic pollutants). The present study highlights the practical applications of luminescent MOFs as sensors and provides a novel Zn-MOF-based sensor for persistent organic pollutants, which is of great importance for living environments and human health.
Conflicts of interest
There are no conflicts to declare.
Acknowledgements
This work was financially supported by the National Natural Science Foundation of China (21371133).
References
-
(a) J. Liu, L. Chen, H. Cui, J. Zhang, L. Zhang and C. Y. Su, Chem. Soc. Rev., 2014, 43, 6011–6061 RSC;
(b) Y. Z. Chen, Z. U. Wang, H. Wang, J. Lu, S. H. Yu and H. L. Jiang, J. Am. Chem. Soc., 2017, 139, 2035–2044 CrossRef CAS PubMed;
(c) W. G. Cui, G. Y. Zhang, T. L. Hu and X. H. Bu, Coord. Chem. Rev., 2019, 387, 79–120 CrossRef CAS;
(d) H. Xu, C. S. Cao, H. S. Hu, S. B. Wang, J. C. Liu, P. Cheng, N. Kaltsoyannis, J. Li and B. Zhao, Angew. Chem., Int. Ed., 2019, 58, 6022–6027 CrossRef CAS PubMed.
-
(a) M. Du, C. P. Li, M. Chen, Z. W. Ge, X. Wang, L. Wang and C. S. Liu, J. Am. Chem. Soc., 2014, 136, 10906–10909 CrossRef CAS PubMed;
(b) T. L. Hu, H. L. Wang, B. Li, R. Krishna, H. Wu, W. Zhou and Y. F. Zhao, Nat. Commun., 2015, 6, 7328 CrossRef CAS PubMed;
(c) C. Freire, M. Nunes, C. Pereira, D. M. Fernandes, A. F. Peixoto and M. Rocha, Coord. Chem. Rev., 2019, 394, 104–134 CrossRef CAS.
-
(a) Z. Hu, B. J. Deibert and J. Li, Chem. Soc. Rev., 2014, 43, 5815–5840 RSC;
(b) Y. Cui, F. Zhu, B. Chen and G. Qian, Chem. Commun., 2015, 51, 7420–7431 RSC;
(c) X. Zhang, W. Wang, Z. Hu, G. Wang and K. Uvdal, Coord. Chem. Rev., 2015, 284, 206–235 CrossRef CAS;
(d) H. F. Fu, L. B. Yan, N. T. Wu, L. F. Ma and S. Q. Zang, J. Mater. Chem. A, 2018, 6, 9183–9191 RSC;
(e) S. Y. Wu, Y. N. Lin, J. W. Liu, W. Shi, G. M. Yang and P. Cheng, Adv. Funct. Mater., 2018, 28, 1707169 CrossRef;
(f) X. Lian and B. Yan, Dalton Trans., 2016, 45, 18668–18675 RSC;
(g) S. Y. Wu, H. Min, W. Shi and P. Cheng, Adv. Mater., 2019, 31, 1805871 CrossRef;
(h) W. Huang, F. F. Pan, Y. Liu, S. D. Huang, Y. J. Li, J. Yong, Y. Li, A. M. Kirillov and D. Y. Wu, Inorg. Chem., 2017, 56, 6362–6370 CrossRef CAS;
(i) W. Huang, J. Jiang, D. Y. Wu, J. Xu, B. Xue and A. M. Kirillov, Inorg. Chem., 2015, 54, 10524–10526 CrossRef CAS PubMed;
(j) J. Z. Gu, Y. H. Cui, J. Wu and A. M. Kirillov, RSC Adv., 2015, 5, 78889–78901 RSC.
-
(a) H. Furukawa, N. Ko, Y. B. Go, N. Aratani, S. B. Choi, E. Choi, A. O. Yazaydin, R. Q. Snurr, M. O'Keeffe, J. Kim and O. M. Yaghi, Science, 2010, 329, 424–428 CrossRef CAS;
(b) Q. L. Zhu and Q. Xu, Chem. Soc. Rev., 2014, 43, 5468–5512 RSC.
-
(a) B. Li, H. M. Wen, Y. J. Cui, W. Zhou, G. D. Qian and B. L. Chen, Adv. Mater., 2016, 28, 8819–8860 CrossRef CAS PubMed;
(b) D. A. Reed, B. K. Keitz, J. Oktawiec, J. A. Mason, T. Runcevski, D. J. Xiao, L. E. Darago, V. Crocella, S. Bordiga and J. R. Long, Nature, 2017, 550, 96 CrossRef CAS;
(c) X. Z. Lian, Y. Fang, E. Joseph, Q. Wang, J. L. Li, S. Banerjee, C. Lollar, X. Wang and H. C. Zhou, Chem. Soc. Rev., 2017, 46, 3386–3401 RSC;
(d) J. P. Zhao, J. Xu, S. D. Han, Q. L. Wang and X. H. Bu, Adv. Mater., 2017, 29, 1606966 CrossRef;
(e) P. Q. Liao, N. Y. Huang, W. X. Zhang, J. P. Zhang and X. M. Chen, Science, 2017, 356, 1193–1196 CrossRef CAS PubMed;
(f) Y. W. Li, H. Yan, T. L. Hu, H. Y. Ma, D. C. Li, S. N. Wang, Q. X. Yao, J. M. Dou, J. Xu and X. H. Bu, Chem. Commun., 2017, 53, 2394–2397 RSC;
(g) X. X. Guo, S. B. Geng, M. J. Zhuo, Y. Chen, M. J. Zaworotko, P. Cheng and Z. J. Zhang, Coord. Chem. Rev., 2019, 391, 44–68 CrossRef CAS.
-
(a) S. Saha, G. Das, J. Thote and R. Banerjee, J. Am. Chem. Soc., 2014, 136, 14845–14851 CrossRef CAS;
(b) M. H. Zeng, Z. Yin, Y. X. Tan, W. X. Zhang, Y. P. He and M. Kurmoo, J. Am. Chem. Soc., 2014, 136, 4680–4688 CrossRef CAS PubMed;
(c) B. Garai, A. Mallick and R. Banerjee, Chem. Sci., 2016, 7, 2195 RSC;
(d) D. Peng, L. Yin, P. Hu, B. Li, Z. W. Ouyang, G. L. Zhuang and Z. X. Wang, Inorg. Chem., 2018, 57, 2577–2583 CrossRef CAS PubMed.
- Z. Xiang, C. Fang, S. Leng and D. Cao, J. Mater. Chem. A, 2014, 2, 7662–7665 RSC.
-
(a) C. A. Bauer, T. V. Timofeeva, T. B. Settersten, B. D. Patterson, V. H. Liu, B. A. Simmons and M. D. Allendorf, J. Am. Chem. Soc., 2007, 129, 7136–7144 CrossRef CAS PubMed;
(b) B. L. Chen, L. B. Wang, F. Zapata, G. D. Qian and E. B. Lobkovsky, J. Am. Chem. Soc., 2008, 130, 6718–6719 CrossRef CAS PubMed;
(c) C. X. Wang, Y. P. Xia, Z. Q. Yao, J. L. Xu, Z. Chang and X. H. Bu, Dalton Trans., 2019, 48, 387–394 RSC.
-
(a) W. Liu, T. Jiao, Y. Li, Q. Liu, M. Tan, H. Wang and L. Wang, J. Am. Chem. Soc., 2004, 126, 2280–2281 CrossRef CAS PubMed;
(b) B. L. Chen, L. B. Wang, Y. Q. Xiao, F. R. Fronczek, M. Xue, Y. J. Cui and G. D. Qian, Angew. Chem., Int. Ed., 2009, 48, 508–511 CrossRef;
(c) Y. W. Li, J. R. Li, L. F. Wang, B. Y. Zhou, Q. Chen and X. H. Bu, J. Mater. Chem. A, 2013, 1, 495–499 RSC;
(d) F. Y. Yi, M. L. Gu, S. C. Wang, J. Q. Zheng, L. Q. Pan and L. Han, Inorg. Chem., 2018, 57, 2654–2662 CrossRef CAS PubMed;
(e) M. Z. Wu, J. Y. Shi, P. Y. Chen and L. Tian, New J. Chem., 2019, 43, 10575–10582 RSC.
-
(a) G. Lu and J. T. Hupp, J. Am. Chem. Soc., 2010, 132, 7832–7833 CrossRef CAS PubMed;
(b) L. E. Kreno, J. T. Hupp and R. P. V. Duyne, Anal. Chem., 2010, 82, 8042–8046 CrossRef CAS PubMed;
(c) J. Liu, F. X. Sun, F. Zhang, Z. Wang, R. Zhang, C. Wang and S. L. Qiu, J. Mater. Chem., 2011, 21, 3775–3778 RSC.
-
(a) L. Wang, G. L. Fan, X. F. Xu, D. M. Chen, L. Wang, W. Shi and P. Cheng, J. Mater. Chem. A, 2017, 5, 5541–5549 RSC;
(b) Y. Rachuri, B. Parmar and E. Suresh, Cryst. Growth Des., 2018, 18, 3062–3072 CrossRef CAS;
(c) Y. Zhou and B. Yan, Chem. Commun., 2016, 52, 2265–2268 RSC.
-
(a) S. Y. Zhang, W. Shi, P. Cheng and M. J. Zaworotko, J. Am. Chem. Soc., 2015, 137, 12203–12206 CrossRef CAS;
(b) Y. Zhou, Q. Yang, D. N. Zhang, N. Gan, Q. P. Li and J. Cuan, Sens. Actuators, B, 2018, 262, 137–143 CrossRef CAS;
(c) X. L. Qu and B. Yan, Inorg. Chem., 2019, 58, 524–534 CrossRef CAS.
- X. Q. Pei, M. Song, M. Guo, F. F. Mo and X. Y. Shen, Atmos. Environ., 2013, 68, 17–23 CrossRef CAS.
-
(a) L. Li, J. Liang, Z. B. Gong, N. N. Zhang and H. L. Duan, Sci. Total Environ., 2017, 580, 388–397 CrossRef PubMed;
(b) K. K. Selvaraj, G. Sundaramoorthy, P. K. Ravichandran, G. K. Girijan, S. Sampath and B. R. Ramaswamy, Environ. Geochem. Health, 2015, 7, 83–96 CrossRef.
- L. Niu, Y. Xu, C. Xu, L. Yun and W. Liu, Environ. Pollut., 2014, 195, 16–23 CrossRef CAS.
-
(a) C. Lin, C. J. Lee, W. M. Mao and F. Nadim, J. Hazard. Mater., 2009, 161, 270–275 CrossRef CAS;
(b) E. P. Hines, A. M. Calatat, M. J. Silva, P. Mendola and S. E. Fenton, Environ. Health Perspect., 2009, 117, 86–92 CrossRef CAS PubMed.
-
(a) Y. Guo, Q. Wu and K. Kannan, Environ. Int., 2011, 37, 893–898 CrossRef CAS PubMed;
(b) Y. Kim, E. H. Ha, E. J. Kim, H. Park, M. Ha, J. H. Kim, Y. C. Hong, N. Chang and B. N. Kim, Environ. Health Perspect., 2011, 119, 1495–1500 CrossRef CAS PubMed;
(c) E. P. Hines, A. M. Calatat, M. J. Silva, P. Mendola and S. E. Fenton, Environ. Health Perspect., 2009, 117, 86–92 CrossRef CAS PubMed.
- R. Sun and H. Zhuang, Food Anal. Methods, 2015, 8, 1990–1999 CrossRef.
- G. Toft, B. A. G. Jonsson, C. H. Lindh, T. K. Jensen, N. H. Hjollund, A. Vested and J. P. Bonde, Environ. Health Perspect., 2012, 120, 458–463 CrossRef CAS PubMed.
- H. T. Gao, R. Xu, W. X. Cao, L. L. Qian, M. Wang, L. G. Lu, Q. Xu and S. Q. Yu, Food Chem. Toxicol., 2017, 101, 94–104 CrossRef CAS PubMed.
-
(a) D. Zhao, D. J. Timmons, D. Yuan and C. Zhou, Acc. Chem. Res., 2011, 44, 123–133 CrossRef CAS PubMed;
(b) J. P. Zhang, Y. B. Zhang, J. B. Lin and X. M. Chen, Chem. Rev., 2012, 112, 1001–1033 CrossRef CAS PubMed;
(c) H. Deng, S. Grunder, K. E. Cordova, C. Valente, H. Furukawa, M. Hmadeh, F. Gándara, A. C. Whalley, Z. Liu, S. Asahina, H. Kazumori, M. O'Keeffe, O. Terasaki, J. F. Stoddart and O. M. Yaghi, Science, 2012, 336, 1018–1023 CrossRef CAS;
(d) D. M. Chen, W. Shi and P. Cheng, Chem. Commun., 2015, 51, 370–372 RSC.
-
(a) X. J. Shi, P. Y. Chen, Z. M. Yin, T. Li, M. Z. Wu and L. Tian, Polyhedron, 2018, 141, 87–93 CrossRef CAS;
(b) J. Park, J. R. Li, E. C. Sañudo, D. Q. Yuan and H. C. Zhou, Chem. Commun., 2012, 48, 883–885 RSC.
-
(a) S. J. Dalgarno, S. A. Tucker, D. B. Bassil and J. L. Atwood, Science, 2005, 309, 2037–2039 CrossRef CAS PubMed;
(b) Y. B. Dong, P. Wang, J. P. Ma, X. X. Zhao, H. Y. Wang, B. Tang and R. Q. Huang, J. Am. Chem. Soc., 2007, 129, 4872–4873 CrossRef CAS PubMed;
(c) G. J. McManus, J. J. Perry, M. Perry, B. D. Wagner and M. J. Zaworotko, J. Am. Chem. Soc., 2007, 129, 9094–9101 CrossRef CAS PubMed;
(d) P. Wang, J. P. Ma, Y. B. Dong and R. Q. Huang, J. Am. Chem. Soc., 2007, 129, 10620–10621 CrossRef CAS PubMed.
- J. Rocha, L. D. Carlos, F. A. A. Paz and D. Ananias, Chem. Soc. Rev., 2011, 40, 926–940 RSC.
- Y. P. Xia, C. X. Wang, R. Feng, K. Li, Z. Chang and X. H. Bu, Polyhedron, 2018, 153, 110–114 CrossRef CAS.
Footnote |
† Electronic supplementary information (ESI) available. CCDC 1946451 (for Zn-1). For ESI and crystallographic data in CIF or other electronic format see DOI: 10.1039/c9ra08417g |
|
This journal is © The Royal Society of Chemistry 2019 |
Click here to see how this site uses Cookies. View our privacy policy here.