DOI:
10.1039/C9RA08167D
(Paper)
RSC Adv., 2019,
9, 41775-41782
Evaluation of the digestibility of steam-exploded wheat straw by ruminal fermentation, sugar yield and microbial structure in vitro
Received
8th October 2019
, Accepted 12th December 2019
First published on 17th December 2019
Abstract
Wheat straw is considered an abundant lignocellulosic biomass source in China. However, its recalcitrance hinders the degradation of wheat straw by enzymes and microbes. In this study, we investigated the optimum steam explosion conditions of pretreated wheat straw by response surface methodology to improve its nutrition level as a feedstuff for the ruminant industry or as a feedstock for biofuel production. The highest volatile fatty acid (VFA) yield (30.50 mmol L−1) was obtained at 2.3 MPa, 90 s and a moisture content of 36.46%. Under optimal conditions, steam explosion significantly altered the fermentation parameters in vitro. Ionic chromatography showed that pretreating wheat straw could improve the production of fermentable sugar, which was ascribed to the degradation of cellulose and hemicellulose. In addition, high throughput 16S rRNA amplicon sequencing analysis revealed that steam explosion changed the microbial community and enhanced the colonization of cellulolytic bacteria. Our findings demonstrated that steam explosion pretreatment could greatly improve the digestibility of wheat straw by facilitating sugar production and microbial colonization.
Introduction
Wheat straw is a plentiful and available crop residue material around the world. Consisting principally of cellulose (30–40%), hemicellulose (20–30%), and lignin (10–20%), wheat straw serves as a potential feedstock source for ruminants or biofuel1,2 because it can be digested by rumen microorganisms and fibrolytic enzymes and produce volatile fatty acid (VFA).3,4 However, the complex and rigid structure of the lignin that surrounds cellulose makes it less available for hydrolysis. Cellulose crystallinity and its degree of polymerization are also parameters that increase its nature of recalcitrance.5 Therefore, an effective pretreatment is a prerequisite to improve the digestibility of wheat straw.
Steam explosion (SE) has been widely used in the pretreatment of lignocellulosic biomass according to its reduced environmental impact, low hazardous chemicals release and high-efficiency.6,7 SE pretreatment is based on the reduction of cellulose crystallinity, reducing the degree of lignification and hydrolysing numerous hemicelluloses.8–10 Several studies have reported that SE can largely improve biomass utilization efficiency as a source for biofuel production or for animal feed, such as cotton stalk, rapeseed straw and corn strove.8,11,12 Furthermore, for ruminants, the presence of bacteria is regarded as one of the most important contributors to digesting lignocellulosic biomass into monomers and oligomers, which are further converted into VFA, methane (CH4), carbon dioxide (CO2), etc.13 VFA serves as more than 70% of the energy for the host since it is easily absorbed by blood and ferried into the liver.14,15 Furthermore, VFA is an attractive precursor to biogas, alcohol-based fuel and biodegradable plastic.16,17 Indeed, the content of VFA produced in the rumen is an appealing parameter for determining the performance of SE treatment of lignocellulose.
Although changes in the physical structure and biomass composition of biomass after SE have been reported in previous studies,12,18 the effects of pretreated feedstuffs, after the optimization of explosion parameters, on sugar yield, microbial diversity in vitro fermentation were scarce. Hence, the objective of the present study was to investigate the optimal SE pretreatment parameters (steam pressure, treatment time and moisture content) to improve the digestibility of wheat straw by response surface methodology (RSM) analysis. Then, the influences of SE pretreatment on the fermentation parameters and sugar production in vitro were characterized. In addition, the bacterial community of the steam exploded wheat straw and subsequent in vitro cultures were proposed by high-throughput sequencing. Overall, the data analysis obtained assessed the digestibility of lignocellulosic feedstocks.
Results and discussion
RSM optimized SE pretreatment process
The amount of VFA produced in anaerobic digestion is closely related to the transformation of lignocellulosic biomasses into useful resources, with the higher production of VFA by rumens indicating a better utilization of substrate. To obtain the maximal VFA production (response value), wheat straw was performed under seventeen different steam explosion conditions according to the Box–Behnken design of RSM. ANOVA data indicated that the predicted response surface model was statistically significant (P < 0.05), the lack of fit was insignificant (P > 0.05) and the correlation coefficient value R2 was 0.8637 which confirmed that this regression model was suitable for predicting the influence of the three factors on VFA production. Additionally, the treatment time, the interaction between steam pressure and treatment time, and the moisture content quadratic effect had significant effects (P < 0.05) on VFA production (Fig. 1). Based on these results and the significance of the model, we selected steam pressure at 2.3 MPa, moisture of 36.46% and treatment time of 90 s for the optimal conditions of the SE pretreatment of wheat straw. Under the optimum conditions, the actual measured value of VFA was consistent with the predicted value. Compared with a previous report, when the evaluation was sugar yield, the optimum pretreated levels were obtained at 180 °C for 10 min.19 However, the optimum conditions in this study were moderate and more likely to be a reasonable pretreatment for wheat straw.
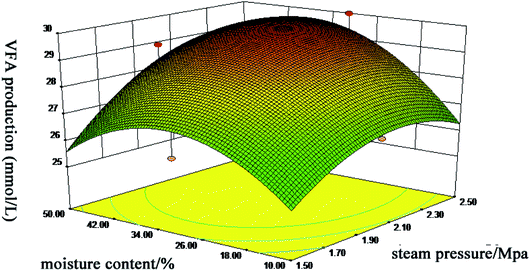 |
| Fig. 1 Response surface of steam pressure and moisture content versus VFA production during in vitro rumen fermentation. | |
Influence of SE treated wheat straw on rumen fermentation parameters in vitro
The results of the main rumen fermentation parameters, including pH value, NH3-N and VFA production after 72 h of incubation, were summarized in Table 1. Compared with untreated wheat straw, SE significantly decreased the pH value of the fermentation fluid (P < 0.01), which may be due to the increased production of organic acids by hydrolysis of acetyl groups in hemicellulose after SE pretreatment.20 More importantly, steam-exploded wheat straw was more likely to degrade carbohydrates into VFA by subsequent in vitro fermentation. Although the pH value decreased in the TRT group, it was still within the suitable range for the growth of rumen microorganisms.21 In addition, a higher (P < 0.01) concentration of NH3-N was observed in the TRT group, which indicated that SE promoted the degradation of nitrogen-containing compounds in the wheat straw. Ammonia is the main source of nitrogen for the growth of rumen fibrinolytic bacteria;22 therefore, the high concentration of ammonia was beneficial to the colonization of rumen bacteria and could improve wheat straw digestion in the rumen.
Table 1 Effect of steam explosion on in vitro rumen fermentation
Items |
Treatment |
SEM |
P value |
CON |
TRT |
pH |
6.61 |
6.58 |
0.006 |
<0.0001 |
NH3-N (mg dL−1) |
16.90 |
18.90 |
0.375 |
0.0050 |
VFA/(mmol L−1) |
27.11 |
30.50 |
0.403 |
<0.0001 |
Acetate/(mmol L−1) |
19.14 |
20.54 |
0.189 |
<0.0001 |
Propionate/(mmol L−1) |
5.65 |
7.72 |
0.175 |
<0.0001 |
Isobutyrate/(mmol L−1) |
0.16 |
0.23 |
0.008 |
<0.0001 |
Butyrate/(mmol L−1) |
1.84 |
2.03 |
0.026 |
<0.0001 |
Isovalerate/(mmol L−1) |
0.21 |
0.30 |
0.011 |
<0.0001 |
Valerate/(mmol L−1) |
0.10 |
0.16 |
0.009 |
0.0003 |
Acetate/propionate |
3.39 |
2.66 |
0.058 |
<0.0001 |
Carbohydrates in wheat straw must be fermented by rumen microorganisms and converted into VFA before absorption. SE treatment significantly increased (P < 0.01) the concentrations of total VFA, acetate, propionate, isobutyrate, butyrate, isovalerate and valerate (Table 1). A large increase in the VFA concentration in TRT group implied that SE could promote the release of nutrients in wheat straws. A relatively low (P < 0.01) ratio of acetate to propionate was observed in the present study, which suggested that the proportion of propionate produced by non-structural carbohydrates was high, while the proportion of acetate produced by structural carbohydrates was relatively low in the TRT group compared with those in the untreated group. These phenomena demonstrated that SE was an effective method to accelerate the conversion of structural carbohydrates to non-structural carbohydrates in wheat straw and further produced large amounts of VFA. This enhancement was probably attributed to (1) the waxy layer on the surface of exploded wheat straw disappearing and the surface becoming rougher and more disordered, which increased the microbial accessibility to nutrients,12,23,24 and (2) after pretreatment, the polymerization of lignin was reduced, and the linkages between lignin and hemicellulose was broken, improving the subsequent fermentation of wheat straw.12,25,26 Consistently, the results of pH value, NH3-N and VFA production showed that SE could increase the nutritional value and thus improve the utilization efficiency of wheat straw, which was consistent with the results of previous studies.27,28
Influence of steam explosion on sugar yield
Performing SE on wheat straw was similar to existing studies, resulting in cellulose and hemicellulose being depolymerized into numerous fermentable sugars, which facilitate the growth of microbial colonies producing cellulolytic enzymes.29 The quantities of the main soluble sugars in both groups were exhibited in Table 2, while minor sugars such as galactose and mannose were not measured because of their very low contents. The analysis identified that SE pretreatment enhanced the concentrations of hemicellulose-derived sugars (xylose and arabinose) (P < 0.05), since the promotion of hemicelluloses solubilization and hydrolysis occurred during pretreatment, and the main components of hemicelluloses were xylan and arabinan,30 which could degrade to xylose and arabinose, respectively. It should be noted that the removal of hemicellulose by loosening the bonds between hemicellulose and lignin during the SE process revealed an increase in specific surface area that was conducive to promoting enzymatic and microbial attacks and further converted hemicellulose to hemicellulose-derived sugars.31–33 The reason for the enhancement of fructose and maltose in SE pretreated wheat straw was that the starch was more effective to convert into low molecular substances by the pretreatment than the cellulose.34 Additionally, the ruminal microorganisms and enzymes could digest starch into fructose, maltose and other low-molecular-weight carbohydrates.35 In the present study, the value of cellobiose (cellulose-derived sugars) was also inevitably increased (P < 0.05) after SE treatment, which showed that SE enhanced the digestion of cellulose. Compared to the untreated wheat straw, the concentration of glucose (cellulose-derived sugars) was statistically similar in the TRT group, which was consistent with past reports, such as steam exploded corn straw12 and sugarcane bagasse.36 This might owe to the pretreatment provided adequate nutrition for the growth of rumen microorganisms and stimulated the metabolism and proliferation of microorganisms in the rumen so that rumen microorganism possessed better access to utilize glucose. It is generally recognized that most degradation products (e.g., phenolic compounds, furfural, p-coumaric acid, hydroxymethylfurfural) generated in SE could inhibit enzymatic or microbial activity.24,37 However, some rumen microorganisms could detoxify furfural and hydroxymethylfurfural, and metabolize ferulic acid and p-coumaric acid.38,39 Furthermore, the improved sugar yields clearly demonstrated that the SE does not adversely affect the metabolic activities of the ruminal microbiome and SE treatment was an effective method to render wheat straw into an adequately digestible feedstuff.
Table 2 Sugar analysis of wheat straw before and after steam explosion
Items |
Treatment |
SEM |
P |
CON |
TRT |
Arabinose (μg ml−1) |
0.03 |
0.07 |
0.0008 |
0.0434 |
Fructose (μg ml−1) |
49.15 |
105.16 |
6.0330 |
<0.0001 |
Maltose (μg ml−1) |
0.28 |
0.50 |
0.0838 |
0.0189 |
Cellobiose (μg ml−1) |
1.05 |
2.15 |
0.4580 |
0.0200 |
Xylose (μg ml−1) |
0.05 |
0.15 |
0.0186 |
0.0048 |
Glucose (μg ml−1) |
2.56 |
3.04 |
0.1300 |
0.4908 |
Influence of SE on rumen microbial diversity and structure
Principal coordinate analysis (PCoA) based on weighted UniFrac distance metrics was conducted to estimate ruminal bacterial changes. As depicted in Fig. 2, PCoA axes 1 and 2 accounted for 50.6% and 15.4% of the total variation, respectively. Compared with the CON group, the microbial communities in the TRT group were effectively separated by PCo1, indicating that SE played a significant role in changing the microbial community diversity.
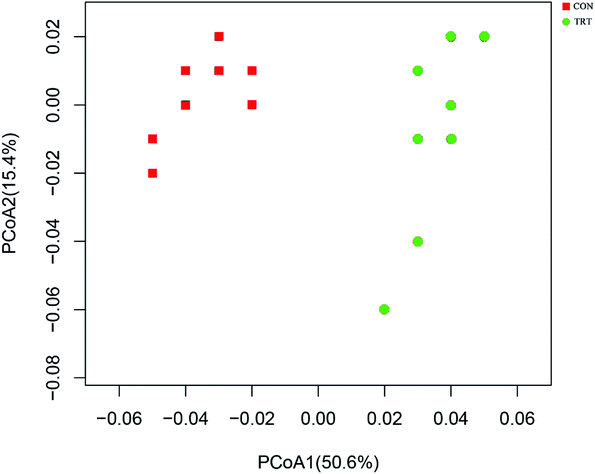 |
| Fig. 2 PcoA describes the bacterial composition. CON, untreated wheat straw; TRT, steam explosion treated wheat straw. | |
The bacterial communities after fermentation for 72 h in both groups were illustrated in Fig. 3. At the phylum level, the rumen microbiota was dominated by Firmicutes (52.23%) and Bacteroidetes (34.69%), which constituted more than 86% of the total community. Verrucomicrobia, Actinobacteria, Proteobacteria and Lentisphaerae accounted for 3.50%,1.67%, 1.48% and 1.01% of the microbial community, respectively (Fig. 3a). Compared with that of the CON group, the relative abundance of the phylum Firmicutes increased (P < 0.01) greatly in the TRT group (Fig. 3b). Firmicutes, containing amounts of species that secrete multiple cellulolytic enzymes and cellulosome complexes, were the major contributors to the digestion of cellulose and hemicellulose.40 Interesting, the structure of lignocellulosic biomass was disrupted and the accessibility of cellulose and hemicellulose to rumen microorganisms increased during the process of SE; hence, steam exploded wheat straw assisted in the colonization of Firmicutes during in vitro fermentation.41 However, SE had little effect on the relative abundance of the phylum Bacteroidetes (P > 0.05) (Fig. 3b). This difference in the relative abundance changes of Firmicutes and Bacteroidetes was most likely due to SE treatment could increase the digestibility of lignocellulose, while the Firmicutes contain more microorganisms that could digest lignocellulose than the Bacteroidetes.42 In addition, Bacteroidetes performed great capability of digesting small organic molecules.43
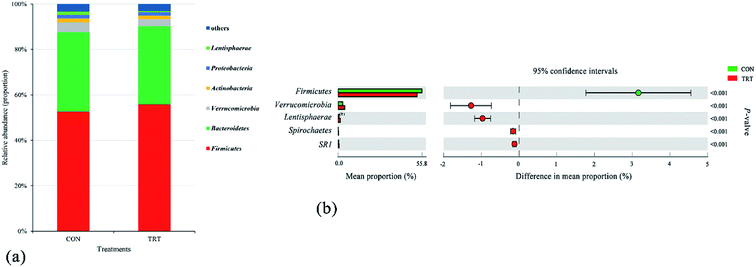 |
| Fig. 3 Composition of active bacterial communities at the phyla level. (a) Quantification of predominant bacterial phyla present in in vitro rumen fermentation. (b) Changes in the relative abundance of the predominant bacteria present in in vitro rumen fermentation at the phyla level (% is used to describe the phyla abundance). An extended error bar plot was generated by bioinformatics software (STAMP). Welch's-two-sided test was used, and Welch's inverted was 0.95. CON, untreated wheat straw; TRT, steam explosion treated wheat straw. | |
In the present study, eighteen genera that accounted for more than 0.1% of the total sequences were identified. The seven predominant taxa included Prevotella (5.02%), Succiniclasticum (4.88%), Subdivision5_genera_incertae_sedis (3.41%), Ruminococcus (2.62%), Acetobacteroides (2.48%), Saccharofermentans (2.21%), and Lachnospiracea_incertae_sedis (2.11%) (Fig. 4a). This result was consistent with previous findings.44,45 Compared with the untreated wheat straw, SE pretreated straw significantly increased the relative abundances of Saccharofermentans, Lachnospiracea_incertae_sedis, Pseudoflavonifractor, Clostridium XlVa, Paraprevotella, Sporobacter and Butyrivibrio (P < 0.01), significantly decreased the relative abundances of Succiniclasticum, Subdivision5_genera_incertae_sedis and Selenomonas (P < 0.01), and had no effect on the relative abundances of Prevotella, Ruminococcus, Acetobacteroides, Intestinimonas, Olsenella and Saccharibacteria_genera_incertae_sedis (Fig. 4b). Piao et al.46 and Dai et al.47 reported that the genera Prevotella, Ruminococcus, Butyrivibrio and Clostridium played important roles in the degradation process of feedstuffs in the rumen. Prevotella, as the main genus in the rumen, contributed to hydrolysing carbohydrates and crude proteins.48 Studies showed that the relative abundances of Prevotella was positively correlated with the concentration of crude protein in the rumen, while wheat straw had a low crude protein content regardless of steam explosion pretreatment, which provided a rational explanation for why no change was observed in the relative abundances of Prevotella in the TRT group.45,49 Reports showed that the genera Butyrivibrio and Clostridium belong to the phylum Firmicutes and these genera were associated with oligosaccharide-degrading and fibre-degrading processes;47,50 the genus Clostridium could produce abundant cellulases in the rumen.42 Therefore, the elevated relative abundance of Butyrivibrio and Clostridium in the TRT group revealed that SE promoted the colonization of fibrolytic bacteria. Given that wheat straw might consist low content of starch in TRT group, while starch was a basic nutrient for the growth of Succiniclasticum,51 the abundance of the Succiniclasticum genus significantly decreased in the TRT group. Overall, the results concluded that SE promoted the colonization of fibrinolytic bacteria in the rumen and, consequently, converted wheat straw into an easily edible biomass for animals.
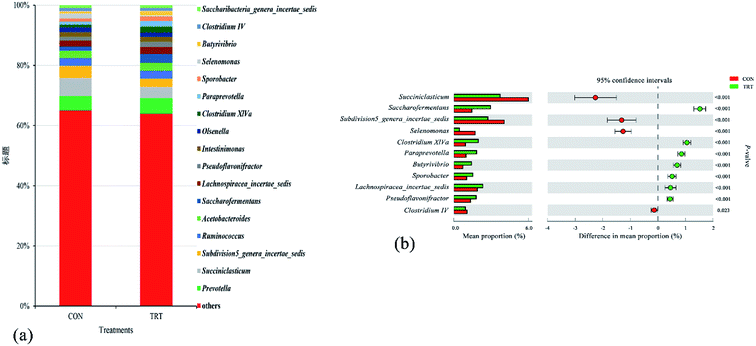 |
| Fig. 4 Composition of bacterial communities at the genus level. (a) Quantification of the predominant bacterial genera present in in vitro rumen fermentation. (b) Changes in the relative abundance of the predominant bacteria present in in vitro rumen fermentation at the genus level (% is used to describe the genus abundance). An extended error bar plot was generated by STAMP. Welch's-two-sided test was used and Welch's inverted was 0.95. CON, untreated wheat straw; TRT, steam explosion treated wheat straw. | |
Correlations between sugar production and predominant bacteria
As mentioned above, the sugar production of steam exploded wheat straw improved at a fermentation time of 72 h of in vitro. It is well known that soluble sugars, including monosaccharides (glucose and fructose) and disaccharides (cellobiose and maltose), were more available for the microorganisms in the ruminal cultures in vitro.52,53 In this fermentation process, the genera Prevotella and Butyrivibrio converted hemicellulose into xylose and arabinose;54 moreover, glucose, cellobiose and maltose were substrates for Butyrivibrio metabolism,55 which might explain the lack of change in the glucose concentration in the TRT group. In addition, Clostridium was known to digest cellulose into glucose, cellobiose and xylose;56 thus, the increased abundance of Clostridium after steam explosion as described above had a positive correlation with the elevated concentrations of the degradation products. Succiniclasticum is a typical fibrolytic ruminal bacteria, which can degrade fiber or cellobiose to produce succinic acid or acetate, respectively. One possible reason for the relative abundance of Succiniclasticum was lower in the TRT group (Fig. 4b) could be that the promotion of hydrolysis by SE treatment further declined the concentration of starch, which in the most important nutrient for the Succiniclasticum genus.51
Experimental
Substrate and inoculum
The winter wheat straw was harvested in June 2018 in Shijiazhuang, Hebei Province, China (114°26′E, 38°03′N). The air-dried wheat straw was manually cut to fragments with a length of 3–5 cm using scissors, kept in airtight sealed plastic bags and stored at room temprature prior to steam explosion. Fresh ruminal fluid for in vitro incubation was obtained from three ruminally cannulated lactating Holstein dairy cows. The cows were fed twice daily at 6:00 am and 5:00 pm with a total mixed ration (TMR) and had free access to water. Ruminal fluid was collected 2 h after the morning feeding and transferred within 30 min to the laboratory, where it was quickly filtered with four layers of cheesecloth. The filtered rumen fluid was then mixed with a buffer solution57 at a 1
:
2 (v/v) ratio under continual CO2 flow in a water bath at 39 °C.
Determination of optimal steam explosion parameters by in vitro incubation
Box–Behnken design was employed to determine the optimum steam explosion condition. The investigated parameters were steam pressure, treatment time and moisture content at the levels of 1.5, 2.0 and 2.5 MPa, 90, 150, 210 s and 10%, 30% and 50%, respectively. According to the Box–Behnken design, 17 treatment groups were established. Steam explosion pretreatment was performed in a 20 L reactor chamber (QB-300, Hebi Gentle Bioenergy Co. Ltd., China). At the end of the reaction, all pretreated samples were dried at 45 °C for 24 h, milled into powder, and screened through a 10-mesh screen. Finally, the pretreated wheat straw powder was stored in airtight sealed plastic bags and used for further analysis.
Steam-exploded wheat straw powder (0.5 g dry matter) was then fermented in a 100 ml serum bottle containing 75 ml of inoculum-buffer solution and kept in a 39 °C incubator for 72 h on an automated trace gas recording system for microbial fermentation. After incubation, rumen fluid was sampled for further VFA analysis.
Based on the VFA production during in vitro fermentation and the significance of the regression coefficients of the second-order polynomial model, the optimal steam explosion wheat straw (TRT group) condition for steam pressure was 2.30 MPa, the treatment time was 90 s, and the moisture content was 36.46%. Therefore, another batch of the raw material was pretreated under the optimum parameters, oven-dried at 45 °C for 24 h and milled to pass through a 10-mesh screen.
Characterization of steam exploded wheat straw by ruminal cultures in vitro
The batch experiments were conducted with biomass pretreated under the optimal steam explosion conditions, followed by the previously described processes. At the end of the 72 h incubation, the contents of each serum bottle were filtered through pre-weighed nylon bags (8 cm wide × 12 cm long, 42 μm aperture), immediately rinsed under running water until the water was clear, and subsequently oven-dried at 55 °C for 48 h. The incubated liquid samples were also collected and frozen at −80 °C for DNA extraction.
In vitro fermentation parameters
The pH value of the fermentation liquid was monitored by a portable pH meter (Seven GoTM portable pH meter, Mettler Toledo, Switzerland). The concentrations of VFA were determined by a gas chromatograph (7890B, Agilent Technologies, United States) equipped with a flame ionization detector and a capillary column (30 m × 0.250 mm × 0.25 μm; BD-FFAP, Agilent Technologies, United States). The ammonia-nitrogen (NH3-N) concentration was measured as described by Broderick and Kang.58
Sugar analysis
The sugars (glucose, fructose, xylose, cellobiose, maltose and arabinose) were quantified by ionic chromatography (Thermo Fisher Scientific, Massachusetts, United States). The flow rate was 0.5 ml min−1 and the temperature was 30 °C. A mixture of deionized water and 200 mM NaOH was used as the mobile phase.
Determination of the microbial community (DNA extraction, PCR amplification and Illumina sequencing)
DNA of the microbes was extracted with an extraction kit (E.Z.N.ATM Mag-Bind Soil DNA Kit, Omega, Norcross, Georgia, United States) based on the manufacturer's instructions. Then, the concentration and quality of DNA were determined by a spectrometer (Invitrogen, State of California, United States) and 1% agarose gel electrophoresis, respectively. The V3–V4 hypervariable regions of bacterial 16S rRNA were amplified by PCR using the following universal primers: V341F, 5′-CCTACGGGNGGCWGCAG-3′; V805R, and 5′-GACTACHVGGGTATCTAATCC-3′. The PCR system contained 15 μL of 2× Taq Master Mix, 1 μL of 10 μM Bar-PCR primer F, 1 μL of 10 μM Primer R, 10–20 ng of genomic DNA template and enough double distilled H2O to reach a total volume of 30 μl. The amplification procedure included an initial denaturation at 94 °C for 3 min, 5 cycles at 94 °C for 30 s, 45 °C for 20 s and 65 °C for 30 s, 20 cycles at 94 °C for 20 s, 55 °C for 20 s, 72 °C for 30 s, and then a final extension at 72 °C for 5 min. In the second round of PCR, Illumina bridge-type compatible PCR primers were added. The thermocycling parameters were as follows: 3 min initial denaturation at 95 °C; 5 cycles at 94 °C for 20 s, 55 °C for 20 s, 72 °C for 30 s, and finally 72 °C for 5 min.
DNA sequence data were quality-filtered and analysed using QIIME (R Core Team 2013). The average size of the clean reads was 416 bp, and the average sequencing depth was ca. 68
381. Operational taxonomic units (OTUs) were selected based on 97% similarity using UPARSE.59 The relative abundance of bacteria was calculated as a percentage.
Statistical analysis
All experiments were performed at least in triple. Quadratic model prediction and response surface figures were generated by Design-Expert 8.0.6 software (Stat-Ease, Minneapolis, USA). The effects of SE condition on pH, VFA, NH3-N concentrations, sugars production, and bacterial abundance and community were analyzed by One Way Analysis of Variance (ANOVA) using SAS 9.4 software (SAS Institute, Inc., Cary, NC, United States) with a probability level of P < 0.05 for significance of treatment.
Conclusion
The present study optimized steam explosion conditions and demonstrated the pretreatment could improve the degradation of wheat straw by ruminal microbiome. Steam explosion pretreatment improved fermentation, the relative abundance of cellulolytic bacteria and the yield of sugar in vitro. These findings revealed that steam explosion is an effective method for improving the nutrient of wheat straw as ruminant feedstuff or biofuel production.
Conflicts of interest
The authors declare that they have no competing interests.
Acknowledgements
The project was financially supported by the National Key Research and Development Plan (2016YFD0700205, 2017YFD0701604).
References
- M. K. Bhat, Biotechnol. Adv., 2000, 18, 355–383 CrossRef CAS PubMed.
- J. Li, R. Zhang, M. A. Siddhu, Y. He, W. Wang, Y. Li, C. Chen and G. Liu, Bioresour. Technol., 2015, 181, 345–350 CrossRef CAS PubMed.
- S.-Q. Tian, R.-Y. Zhao and Z.-C. Chen, Renewable Sustainable Energy Rev., 2018, 91, 483–489 CrossRef CAS.
- L. C. Ferreira, A. Donoso-Bravo, P. J. Nilsen, F. Fdz-Polanco and S. I. Perez-Elvira, Bioresour. Technol., 2013, 143, 251–257 CrossRef CAS PubMed.
- F.-X. Collard and J. Blin, Renewable Sustainable Energy Rev., 2014, 38, 594–608 CrossRef CAS.
- P. Alvira, E. Tomas-Pejo, M. Ballesteros and M. J. Negro, Bioresour. Technol., 2010, 101, 4851–4861 CrossRef CAS PubMed.
- A. Prasad, M. Sotenko, T. Blenkinsopp and S. R. Coles, Int. J. Life Cycle Assess., 2016, 21, 44–50 CrossRef CAS.
- Y. Huang, X. Wei, S. Zhou, M. Liu, Y. Tu, A. Li, P. Chen, Y. Wang, X. Zhang, H. Tai, L. Peng and T. Xia, Bioresour. Technol., 2015, 181, 224–230 CrossRef CAS PubMed.
- J. M. Lee, H. Jameel and R. A. Venditti, Bioresour. Technol., 2010, 101, 5449–5458 CrossRef CAS PubMed.
- W. Jin, L. Chen, M. Hu, D. Sun, A. Li, Y. Li, Z. Hu, S. Zhou, Y. Tu, T. Xia, Y. Wang, G. Xie, Y. Li, B. Bai and L. Peng, Appl. Energy, 2016, 175, 82–90 CrossRef CAS.
- I. P. Wood, A. Elliston, S. R. Collins, D. Wilson, I. Bancroft and K. W. Waldron, Bioresour. Technol., 2014, 162, 175–183 CrossRef CAS PubMed.
- S. Zhao, G. Li, N. Zheng, J. Wang and Z. Yu, Bioresour. Technol., 2018, 253, 244–251 CrossRef CAS PubMed.
- J. Gharechahi and G. H. Salekdeh, Biotechnol. Biofuels, 2018, 11, 216 CrossRef PubMed.
- E. N. Bergman, Physiol. Rev., 1990, 70, 567–590 CrossRef CAS PubMed.
- F. Godoy-Vitorino, K. C. Goldfarb, U. Karaoz, S. Leal, M. A. Garcia-Amado, P. Hugenholtz, S. G. Tringe, E. L. Brodie and M. G. Dominguez-Bello, ISME J., 2012, 6, 531–541 CrossRef CAS PubMed.
- J. Yu, J. Biotechnol., 2001, 86, 105–112 CrossRef CAS PubMed.
- M. T. Agler, B. A. Wrenn, S. H. Zinder and L. T. Angenent, Trends Biotechnol., 2011, 29, 70–78 CrossRef CAS PubMed.
- X. Zhang, Q. Yuan and G. Cheng, Carbohydr. Polym., 2017, 156, 351–356 CrossRef CAS PubMed.
- I. Ballesteros, M. J. Negro, J. M. Oliva, A. Cabanas, P. Manzanares and M. Ballesteros, Appl. Biochem. Biotechnol., 2006, 129–132, 496–508 CrossRef CAS PubMed.
- M. Laser, D. Schulman, S. G. Allen, J. Lichwa, M. J. Antal Jr and L. R. Lynd, Bioresour. Technol., 2002, 81, 33–44 CrossRef CAS PubMed.
- T. G. Nagaraja and E. C. Titgemeyer, J. Dairy
Sci., 2007, 90, E17–E38 CrossRef PubMed.
- M. P. Bryant, Fed. Proc., 1973, 32, 1809–1813 CAS.
- Z. Xiao, G. Han, J. Wei, Y. Zhang, X. Li and M. Li, Bioresources, 2016, 11, 6590–6599 Search PubMed.
- Z. H. Liu and H. Z. Chen, Bioresour. Technol., 2017, 223, 47–58 CrossRef CAS PubMed.
- L.-h. Zhang, D. Li, L.-j. Wang, T.-p. Wang, L. Zhang, X. D. Chen and Z.-h. Mao, Bioresour. Technol., 2008, 99, 8512–8515 CrossRef CAS PubMed.
- D. Sun, A. Alam, Y. Tu, S. Zhou, Y. Wang, T. Xia, J. Huang, Y. Li, Zahoor, X. Wei, B. Hao and L. Peng, Bioresour. Technol., 2017, 239, 74–81 CrossRef CAS PubMed.
- L. W. He, Q. X. Meng, D. Y. Li, F. Wang and L. P. Ren, Ital. J. Anim. Sci., 2016, 14, 727–733 Search PubMed.
- E. Viola, F. Zimbardi, M. Cardinale, G. Cardinale, G. Braccio and E. Gambacorta, Bioresour. Technol., 2008, 99, 681–689 CrossRef CAS PubMed.
- J. Miron, D. Ben-Ghedalia and M. Morrison, J. Dairy Sci., 2001, 84, 1294–1309 CrossRef CAS PubMed.
- E. Varga, K. Reczey and G. Zacchi, Appl. Biochem. Biotechnol., 2004, 113–116, 509–523 CrossRef CAS PubMed.
- M. C. Fernandes, M. D. Ferro, A. F. C. Paulino, J. A. S. Mendes, J. Gravitis, D. V. Evtuguin and A. Xavier, Bioresour. Technol., 2015, 186, 309–315 CrossRef CAS PubMed.
- M. Kapoor, T. Raj, M. Vijayaraj, A. Chopra, R. P. Gupta, D. K. Tuli and R. Kumar, Carbohydr. Polym., 2015, 124, 265–273 CrossRef CAS PubMed.
- J. Chang, W. Cheng, Q. Yin, R. Zuo, A. Song, Q. Zheng, P. Wang, X. Wang and J. Liu, Bioresour. Technol., 2012, 104, 587–592 CrossRef CAS PubMed.
- F. Kobayashi, T. Sawada, Y. Nakamura, M. Ohnaga, M. Godliving and T. Ushiyama, Appl. Biochem. Biotechnol., 1998, 69, 177–189 CrossRef CAS PubMed.
- K. Barsuhn and S. F. Kotarski, J. Chromatogr., 1991, 546, 273–287 CrossRef CAS PubMed.
- P. Thungklin, S. Sittijunda and A. Reungsang, Int. J. Hydrogen Energy, 2018, 43, 9924–9934 CrossRef CAS.
- J. Zhu, L. Shi, L. Zhang, Y. Xu, Q. Yong, J. Ouyang and S. Yu, Bioprocess Biosyst. Eng., 2016, 39, 1619–1626 CrossRef CAS PubMed.
- D. Salvachua, H. Smith, P. C. St John, A. Mohagheghi, D. J. Peterson, B. A. Black, N. Dowe and G. T. Beckham, Bioresour. Technol., 2016, 214, 558–566 CrossRef CAS PubMed.
- B. B. Cao, X. Jin, H. J. Yang, S. L. Li and L. S. Jiang, J. Sci. Food Agric., 2016, 96, 650–655 CrossRef PubMed.
- L. Zhang, J. Chung, Q. Jiang, R. Sun, J. Zhang, Y. Zhong and N. Ren, RSC Adv., 2017, 7, 40303–40310 RSC.
- L. Treu, P. G. Kougias, S. Campanaro, I. Bassani and I. Angelidaki, Bioresour. Technol., 2016, 216, 260–266 CrossRef CAS PubMed.
- T. H. Do, T. K. Dao, K. H. V. Nguyen, N. G. Le, T. M. P. Nguyen, T. L. Le, T. N. Phung, N. M. van Straalen, D. Roelofs and N. H. Truong, Asian-Australas. J. Anim. Sci., 2018, 31, 738–747 CrossRef PubMed.
- A. E. Naas, A. K. Mackenzie, J. Mravec, J. Schückel, W. G. T. Willats, V. G. H. Eijsink and P. B. Pope, mBio, 2014, 5, 1401–1414 CrossRef PubMed.
- W. Jin, Y. Wang, Y. Li, Y. Cheng and W. Zhu, Anaerobe, 2018, 50, 1–8 CrossRef PubMed.
- J. Liu, M. Zhang, C. Xue, W. Zhu and S. Mao, J. Dairy Sci., 2016, 99, 9668–9681 CrossRef CAS PubMed.
- H. Piao, M. Lachman, S. Malfatti, A. Sczyrba, B. Knierim, M. Auer, S. G. Tringe, R. I. Mackie, C. J. Yeoman and M. Hess, Front. Microbiol., 2014, 5, 307 Search PubMed.
- X. Dai, Y. Tian, J. Li, Y. Luo, D. Liu, H. Zheng, J. Wang, Z. Dong, S. Hu and L. Huang, Appl. Environ. Microbiol., 2015, 81, 1375–1386 CrossRef PubMed.
- J. Chiquette, M. J. Allison and M. A. Rasmussen, J. Dairy Sci., 2008, 91, 3536–3543 CrossRef CAS PubMed.
- D. M. Stevenson and P. J. Weimer, Appl. Microbiol. Biotechnol., 2007, 75, 165–174 CrossRef CAS PubMed.
- S. M. Carver, M. C. Nelson, R. Lepisto, Z. Yu and O. H. Tuovinen, Bioresour. Technol., 2012, 104, 424–431 CrossRef CAS PubMed.
- D. An, X. Dong and Z. Dong, Anaerobe, 2005, 11, 207–215 CrossRef CAS PubMed.
- I. J. Lean, T. K. Webster, W. Hoover, W. Chalupa, C. J. Sniffen, E. Evans, E. Block and A. R. Rabiee, J. Dairy Sci., 2005, 88, 2524–2536 CrossRef CAS PubMed.
- S. R. Stokes, W. H. Hoover, T. K. Miller and R. P. Manski, J. Dairy Sci., 1991, 74, 860–870 CrossRef CAS PubMed.
- M. A. Cotta and R. L. Zeltwanger, Appl. Environ. Microbiol., 1995, 61, 4396–4402 CAS.
- H. J. Strobel and K. A. Dawson, FEMS Microbiol. Lett., 1993, 113, 291–296 CrossRef CAS PubMed.
- Z. X. Song, X. H. Li, W. W. Li, Y. X. Bai, Y. T. Fan and H. W. Hou, Bioresour. Technol., 2014, 157, 91–97 CrossRef CAS PubMed.
- K. H. Menke, Animal Research and Development, 1988, 28, 7–55 Search PubMed.
- G. A. Broderick and J. H. Kang, J. Dairy Sci., 1980, 63, 64–75 CrossRef CAS.
- R. C. Edgar, B. J. Haas, J. C. Clemente, C. Quince and R. Knight, Bioinformatics, 2011, 27, 2194–2200 CrossRef CAS PubMed.
Footnote |
† These authors contributed equally to this work. |
|
This journal is © The Royal Society of Chemistry 2019 |