DOI:
10.1039/C9RA07809F
(Paper)
RSC Adv., 2019,
9, 39466-39474
Rapid and catalyst free synthesis of new bis(benzo[g]chromene) and bis(pyrano[3,2-c]chromene) derivatives and optimization of reaction conditions using response surface methodology†
Received
25th September 2019
, Accepted 25th November 2019
First published on 2nd December 2019
Abstract
4,4′-(1,4-phenylene)bis(2-(alkylamino)-3-nitro-4H-benzo[g]chromene-5,10-dione) and 4,4′-(1,4-phenylene)bis(2-(alkylamino)-3-nitropyrano[3,2-c]chromen-5(4H)-one) derivatives are synthesized by a one-pot, multi-component reaction of N-alkyl-1-(methylthio)-2-nitroethenamine (derived from the reaction of various amines and 1,1-bis(methylthio)-2-nitroethene) with terephthalaldehyde or isophthalaldehyde, and 2-hydroxy-1,4-naphthoquinone or 4-hydroxycoumarin in EtOH/H2O (85
:
15) as the solvent at 89 °C. Response surface methodology (RSM) is used to investigate the effect of reaction temperature and water content of aqueous ethanol on the product yields and reaction time. The notable features of this work are the optimization of reaction conditions with minimal experiments, absence of catalyst, good yields, simple work-up and the non-chromatographic purification of products.
Introduction
Response surface methodology (RSM), is one of the most commonly used experimental designs for optimizing process conditions.1–7 Traditionally, optimization has been carried out by monitoring the influence of one factor at a time on an experimental response.8 RSM is a more economical method because a small number of experiments are carried out for monitoring the interaction of the independent variables in the response. In conventional optimization, the increase in the number of experiments necessary to perform the research, leads to an increase in time, expense, and the consumption of reagents and materials for experiments.8,9 Hence, response surface methodology (RSM) may be considered as an efficient way to deal with the limitations of the conventional method.10 In the recent years, several studies used successfully RSM for optimizing reaction conditions such as optimization of reaction parameters for the synthesis of chromium methionine complex (Xiao et al., 2013),5 optimization of the synthesis of highly functionalized pyrimido[1,2-b]indazoles via 6-endo-dig cyclization (Roopan et al., 2016),11 and optimization of the reaction conditions for the synthesis of 1-(2-aminoethyl)-2-imidazolidone (Cai et al., 2017).12
Multi-component reactions (MCRs) represent a powerful and efficient method for construction of complex molecules with biological potential.13 They offer remarkable advantages like convergence, operational simplicity, facile automation, reduction in the number of workups, extraction and purification processes.14 Therefore, the design of novel MCRs has attracted great attention from synthetic organic chemists.15–20
Chromenes and their fused analogues are a group of biologically active molecules occurring extensively in nature with a wide range of molecular modifications.21 Among them benzochromenes are one of the privileged scaffolds with a medicinal pharmacophore such as antibacterial,22 antimicrobial,23 antitumor,24 and anti-inflammatory.25–28 Also pyranochromenes are an important and essential category of chromenes and have extensive bioactivities, such as anti-HIV, anti-tuberculosis,29 antitumor,30 anti-cancer, anti-anaphylactic,31 and anticoagulant32 activities.
By the way, oxygen analogues of polycyclic aromatic hydrocarbons33 represent an important class of molecules because the incorporation of oxygen atoms into an aromatic hydrocarbon system can modulate its pharmacological activity as well as electrical or optical properties.34 Therefore, the synthesis of these class of chromene compounds has fascinated much noticed in organic synthesis and many researchers have reported synthesis of these important compounds over the past few years.35–49
Ketene dithioacetals are important precursors in organic synthesis of a variety of heterocyclic compounds and they have received increasing attention recently.50–54 As part of our continuing research to be develop novel methods for multi-component synthesis of new biologically active heterocyclic compounds from dithioacetals and because of the importance of using RSM in optimization of reaction conditions, herein, we report an efficient synthesis of 4,4′-(1,4-phenylene)bis(2-(alkylamino)-3-nitro-4H-benzo[g]chromene-5,10-dione) and 4,4′-(1,4-phenylene)bis(2-(alkylamino)-3-nitropyrano[3,2-c]chromen-5(4H)-one) derivatives via one-pot, multi-component reaction of various amines, 1,1-bis(methylthio)-2-nitroethene (nitro ketene dithioacetal), terephthalaldehyde or isophthalaldehyde, and 2-hydroxy-1,4-naphthoquinone or 4-hydroxycoumarin in EtOH/H2O (85
:
15) at 89 °C without any catalyst. Central composite design (CCD) one of the most popular RSM55 is selected as the experimental design method for optimizing reaction conditions.
Result and discussion
In the current study, we introduce an efficient one-pot, multi-component reaction of various amines 1, 1,1-bis(methylthio)-2-nitroethene 2, terephthalaldehyde or isophthalaldehyde 3, and 2-hydroxy-1,4-naphthoquinone 4 or 4-hydroxycoumarin 5 for the synthesis of 4,4′-(1,4-phenylene)bis(2-(alkylamino)-3-nitro-4H-benzo[g]chromene-5,10-dione) 6 and 4,4′-(1,4-phenylene)bis(2-(alkylamino)-3-nitropyrano[3,2-c]chromen-5(4H)-one) 7 derivatives in EtOH/H2O (85
:
15) as solvent at 89 °C without any catalyst (Scheme 1).
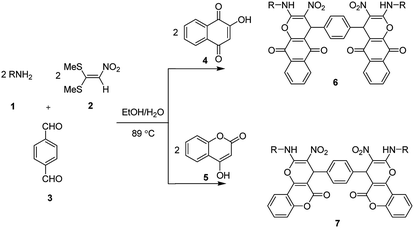 |
| Scheme 1 Synthetic scheme for the products 6 and 7. | |
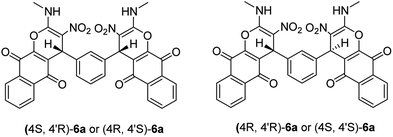 |
| Scheme 2 The two diastereoisomers of 6a. | |
Response surface methodology (RSM) using central composite design (CCD) with five replicates at the center point was applied to optimize the reaction conditions. We used the reaction of N-methyl-1-(methylthio)-2-nitroethenamine (2 mmol), terephthalaldehyde (1 mmol), and 2-hydroxy-1,4-naphthoquinone (2 mmol) for the preparation of compound 6b as a model. The two independent variables (temperature (A) and water content of aqueous ethanol (B)), levels (Table 1), and the results obtained after running the experiments are represented in Table 2. The two responses were analyzed: yield of product (R1) and reaction time (R2).
Table 1 Selected variables and levels used in central composite design
Variables |
Code |
Units |
Levels |
−1 |
0 |
+1 |
Reaction temperature |
A |
°C |
25 |
62.5 |
100 |
Water content of aqueous ethanol |
B |
% |
0 |
50 |
100 |
Table 2 Process variables and experimental data for two factors, three levels response surface designa
Run |
A (°C) |
B (%) |
R1 (%) |
R2 (h) |
A = temperature (°C), B = water content of aqueous ethanol (%), R1 = yield of reaction, R2 = reaction time. |
1 |
36 |
85 |
0 |
24 |
2 |
100 |
50 |
64 |
0.25 |
3 |
62 |
100 |
5 |
24 |
4 |
62 |
50 |
20 |
7 |
5 |
62 |
50 |
26 |
7 |
6 |
62 |
50 |
16 |
7 |
7 |
62 |
50 |
22 |
7 |
8 |
62 |
0 |
47 |
2 |
9 |
36 |
15 |
27 |
24 |
10 |
89 |
15 |
84 |
0.5 |
11 |
62 |
50 |
30 |
7 |
12 |
89 |
85 |
5 |
24 |
13 |
25 |
50 |
0 |
24 |
Optimum conditions with respect to yield, purity of product and reaction time were as follow: temperature 89 °C, water content of aqueous ethanol 15%. Verification experiments, carried out at the predicted conditions showed values reasonably close to those predicted and further confirmed the adequacy of predicted models.
On the basis of the CCD, the cubic model relationship between the experimental yield (R1) and the process variables (temperature (A) and water content of aqueous ethanol (B)) and the quadratic model between the reaction time (R2) and the process variables, in coded units are obtained from eqn (1) and (2) respectively.
|
R1 = 22.80 + 22.63A − 14.85B − 13.00AB + 4.60A2 + 1.60B2 − 11.65A2B − 7.13AB2
| (1) |
|
R2 = 7.00 − 7.14A + 6.83B + 5.88AB + 3.95A2 + 4.39B2
| (2) |
where
R1 and
R2 represent the experimental yield and reaction time respectively, then
A (temperature) and
B (water content of aqueous ethanol) are the coded variables in the reaction.
Fig. 1 and 2 show the linear correlation between the actual and predicted yield and time, respectively. According to the figures, the predicted yield is consistent with the experimental yield and the model can be used to predict the yield of product successfully.
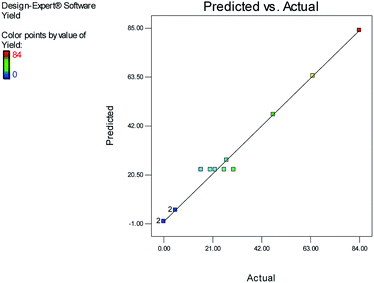 |
| Fig. 1 Linear correlation between the actual and predicted yield. | |
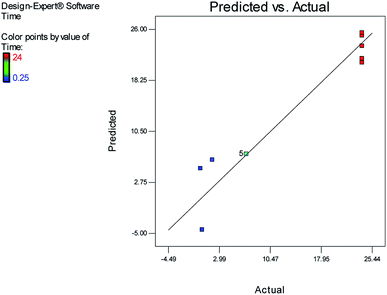 |
| Fig. 2 Linear correlation between the actual and predicted time. | |
The ANOVA for response surface cubic and quadratic models are reported in Tables 3 and 4. According to the Table 3, the model F-value of 46.05 implies the cubic model is significant. There is only a 0.03% chance that a “Model F-Value” this large could occur due to noise. Values of “Prob > F” less than 0.0500 indicate model terms are significant. In this case A, B, AB, A2B are significant model terms. The “Lack of Fit F-value” of 0.00 implies the Lack of Fit is not significant relative to the pure error. There is a 100.00% chance that a “Lack of Fit F-value” this large could occur due to noise. Non-significant Lack of Fit is good and we want the model to fit. The “Pred. R-Squared” of 0.9761 is in reasonable agreement with the “Adj. R-Squared” of 0.9633. “Adeq. Precision” measures the signal to noise ratio. A ratio greater than 4 is desirable. Our ratio of 22.155 indicates an adequate signal. According to the Table 4, the model F-value of 19.38 implies the quadratic model is significant. There is only a 0.06% chance that a “Model F-Value” this large could occur due to noise. The “Pred. R-Squared” of 0.5210 is not as close to the “Adj. R-Squared” of 0.8845 as one might normally expect. This may indicate a large block effect. Also “Adeq. Precision” measures the signal to noise ratio. A ratio greater than 4 is desirable. Our ratio of 12.884 indicates an adequate signal.
Table 3 ANOVA for response surface cubic model
Source |
Sum of Square |
Degree of freedom |
Mean square |
F value |
p-value Prob > F |
Model |
7530.28 |
7 |
1075.75 |
46.05 |
0.0003 significant |
A-temperature |
2048.00 |
1 |
2048.00 |
87.67 |
0.0002 |
B-percentage of water in ethanol |
882.00 |
1 |
882.00 |
37.76 |
0.0017 |
AB |
676.00 |
1 |
676.00 |
28.94 |
0.0030 |
A2 |
147.20 |
1 |
147.20 |
6.30 |
0.0538 |
B2 |
17.81 |
1 |
17.81 |
0.76 |
0.4225 |
A2B |
271.48 |
1 |
271.48 |
11.62 |
0.0191 |
AB2 |
101.60 |
1 |
101.60 |
4.35 |
0.0914 |
A3 |
0.000 |
0 |
|
|
|
B3 |
0.000 |
0 |
|
|
|
Residual |
116.80 |
5 |
23.36 |
|
|
Lack of fit |
0.000 |
1 |
0.000 |
0.000 |
1.0000 not significant |
Pure error |
116.80 |
4 |
29.20 |
|
|
Correlation total |
7647.08 |
12 |
|
|
|
Table 4 ANOVA for response surface quadratic model
Source |
Sum of square |
Degree of freedom |
Mean square |
F value |
p-value |
Model |
1133.23 |
5 |
226.65 |
19.38 |
0.0006 significant |
A-temperature |
407.37 |
1 |
407.37 |
34.84 |
0.0006 |
B-percentage of water in ethanol |
372.82 |
1 |
372.82 |
31.89 |
0.0008 |
AB |
138.06 |
1 |
138.06 |
11.81 |
0.0109 |
A2 |
108.71 |
1 |
108.71 |
9.30 |
0.0186 |
B2 |
134.10 |
1 |
134.10 |
11.47 |
0.0117 |
Residual |
81.85 |
7 |
11.69 |
|
|
Lack of fit |
81.85 |
3 |
27.26 |
|
|
Pure error |
0.000 |
4 |
0.000 |
|
|
Correlation total |
1215.08 |
12 |
|
|
|
The effects of temperature and water content of aqueous ethanol on the total reaction yield, and the total reaction time are shown in Fig. 3 and 4, respectively. The total yield varied from 0% to 84%. As temperature increased, the yield increased and the reaction time decreased. The changes in yield versus the water content of aqueous ethanol is minor compared to that of temperature. As the water content of aqueous ethanol decreased, the yield increased and the reaction time decreased. The highest yield (84%) was obtained at 89 °C and at 15% water. The lowest yield (0%) were at 36 °C, 85% water, and at 25 °C, 50% water.
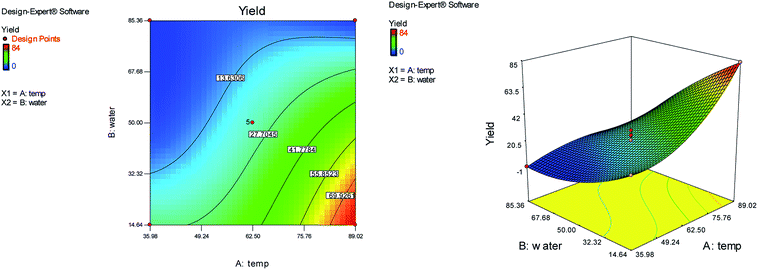 |
| Fig. 3 The effects of temperature and water content of aqueous ethanol on the total reaction yield. | |
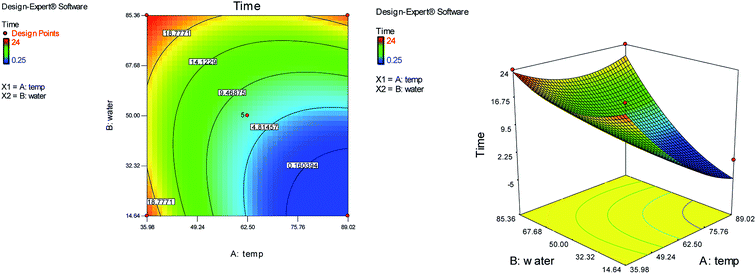 |
| Fig. 4 The effects of temperature and the water content of aqueous ethanol on the total reaction time. | |
After obtaining the optimized reaction conditions, we investigated the scope of this reaction by varying the structure of the amines 1, phthalaldehydes 3, and CH acids (4 and 5). The reaction proceeds cleanly under the same reaction conditions to afford corresponding fused heterocyclic derivatives 6 and 7 (Schemes 3 and 4) in 75–91% yields. The results are shown in Table 5. The reaction did not work, when the reaction was performed using ortho-phthalaldehyde, and benzylamine. The steric hindrance makes it less reactive toward reaction.
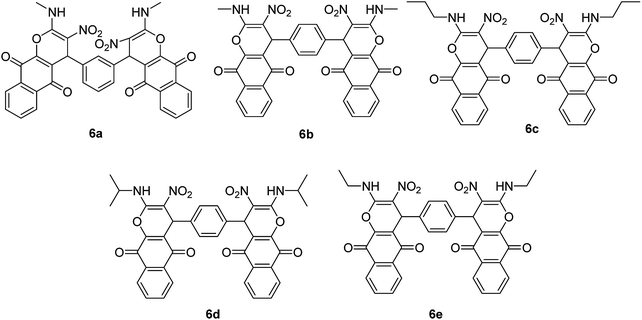 |
| Scheme 3 Products 6a–e. | |
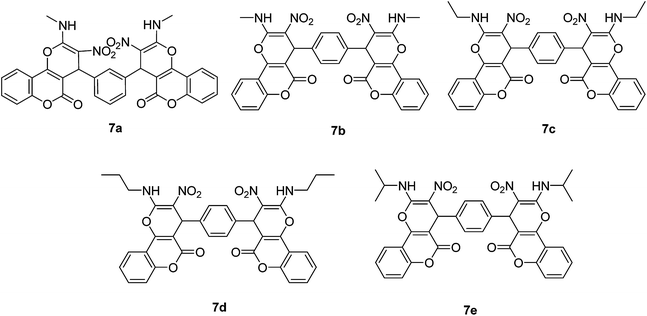 |
| Scheme 4 Products 7a–e. | |
Table 5 One-pot, multi-component synthesis of bis(benzo[g]chromene) (6a–e) and bis(pyrano[3,2-c]chromene) derivatives (7a–e)
Entry |
RNH2a |
Phthalaldehydea |
Product |
Time (min) |
Yield (%) |
Various amines (2 mmol), 1,1-bis(methylthio)-2-nitroethene (2 mmol), phthalaldehyde (1 mmol), 2-hydroxy-1,4-naphthoquinone (2 mmol) and 4-hydroxycumarin (2 mmol) were used. The reactions were run in EtOH/H2O (85 : 15) at 89 °C, without any catalyst. |
1 |
Methylamine |
Isophthalaldehyde |
6a |
25 |
86 |
2 |
Methylamine |
Terephthalaldehyde |
6b |
30 |
84 |
3 |
Propylamine |
Terephthalaldehyde |
6c |
40 |
81 |
4 |
Isopropylamine |
Terephthalaldehyde |
6d |
35 |
79 |
5 |
Ethylamine |
Terephthalaldehyde |
6e |
45 |
75 |
6 |
Methylamine |
Isophthalaldehyde |
7a |
15 |
87 |
7 |
Methylamine |
Terephthalaldehyde |
7b |
15 |
91 |
8 |
Ethylamine |
Terephthalaldehyde |
7c |
30 |
82 |
9 |
Propylamine |
Terephthalaldehyde |
7d |
20 |
89 |
10 |
Isopropylamine |
Terephthalaldehyde |
7e |
25 |
85 |
11 |
Benzylamine |
Terephthalaldehyde |
n.r. |
— |
— |
12 |
Methylamine |
Orthophthalaldehyde |
n.r. |
— |
— |
Structural elucidation of the newly synthesized compounds was accomplished using their IR, mass, 1H NMR, and 13C NMR spectroscopy (see the ESI†). Compounds 6 and 7 have two stereogenic centers, and therefore two diastereoisomers are expected. For examples the two diastereoisomers of 6a is shown in Scheme 2. The 1H and 13C NMR spectra of the products are consistant with the presence of two diastereoisomers. We were not able to separate compounds 6 and 7 in pure form. However, their NMR data can be extracted from the mixture of the two diastereoisomers in a nearly 50
:
50 ratio (based on integration of methine protons). The 1H and 13C NMR spectra of two diastereoisomers are similar except for the alkyl amine and methine groups. Also we did not obtain the good NMR spectra of these samples because of its insolubility in any solvent (all products are very insoluble compounds).
For examples the IR spectrum of 6b indicated absorption bands due to the NH stretching (3613 cm−1) as well as bands at 1667, 1467 and 1363, 1245, and 1192 cm−1 due to the C
O, NO2, C–N, and C–O groups. The 1H NMR spectrum of 6b showed multiplets for four CH3 groups (δ 3.15–3.23 ppm), two singlets for four CH groups (δ 5.23 and 5.28 ppm), multiplets for the aromatic region (δ 7.01–8.04 ppm), and multiplets for four NH groups (δ 10.23–10.35 ppm). The 1H-decoupled 13C NMR spectrum of 6b showed three signals for CH3 and CH groups (δ 29.9, 37.4, 38.0 ppm), and signals at 108.5, 177.6, 182.8, 183.5, and 184.7 ppm, which were assigned C–NO2 and C
O groups, respectively. The mass spectrum of this structure was in accordance with the proposed structure.
A proposed mechanism for the synthesis of 6 and 7 is shown in Schemes 5 and 6. Initially, the Knoevenagel condensation between terephthalaldehyde 3 (1 mmol) and 2-hydroxy-1,4-naphthoquinone 4 (or 4-hydroxycumarin 5) (2 mmol) affords 9 (or 12) which undergoes Michael addition with N-alkyl-1-(methylthio)-2-nitroethenamine 8 (2 mmol) derived from the addition of alkylamine 1 (2 mmol) to 1,1-bis(methylthio)-2-nitroethene 2 (2 mmol), to give 10 (or 13). Thus the intermediate 10 (or 13) undergoes imine-enamine tautomerisation to form 11 (or 14) followed by O-cyclization to form 6 (or 7) via the elimination of MeSH.
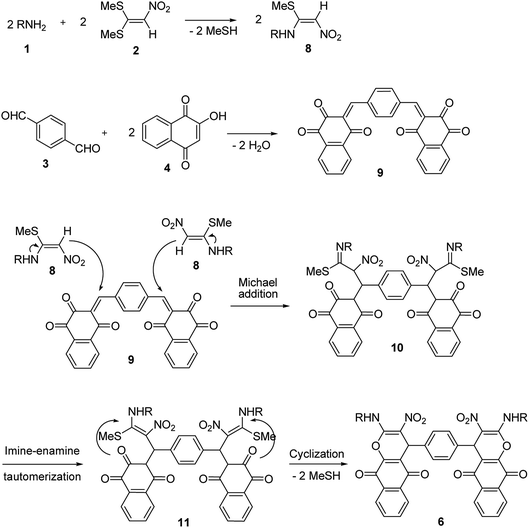 |
| Scheme 5 Proposed mechanism for the synthesis of product 6. | |
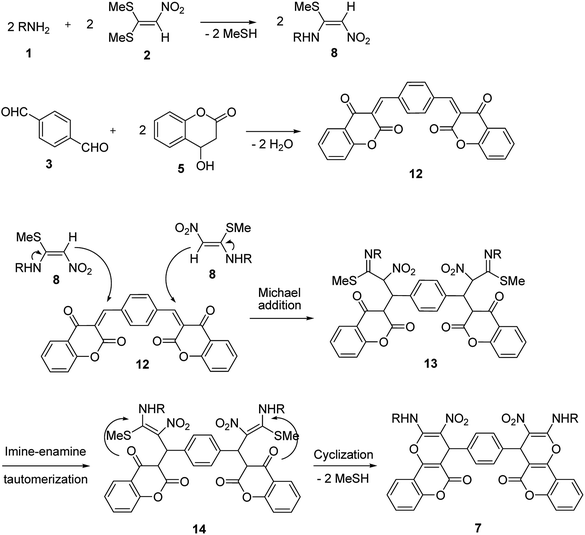 |
| Scheme 6 Plausible mechanism for the formation of product 7. | |
Experimental
General
The various amines, 1,1-bis(methylthio)-2-nitroethene, terephthalaldehyde, isophthalaldehyde, 2-hydroxy-1,4-naphthoquinone, 4-hydroxycumarin, and solvents were purchased from Sigma-Aldrich chemical company and were used as received without further purification. Melting points were determined with an electrothermal 9100 apparatus. Infrared (IR) spectra were recorded on a Bruker Tensor 27 spectrometer. Nuclear magnetic resonance (NMR) spectra were obtained on a Bruker DRX-300 Avance instrument (300 MHz for 1H and 75.4 and 62.8 MHz for 13C) with DMSO as solvent. Chemical shifts are expressed in parts per million (ppm), and coupling constant (J) are reported in hertz (Hz). Mass spectra were recorded with an Agilent 5975C VL MSD with Triple-Axis Detector operating at an ionization potential of 70 eV.
General procedure for the synthesis of product 6 and 7
A mixture of amine (2 mmol), 1,1-bis(methylthio)-2-nitroethene (2 mmol, 0.330 g) and 10 mL EtOH/H2O (85
:
15) in a 50 mL flask was stirred for 6 hours at 89 °C. After completion of the reaction (monitored by TLC, ethyl acetate/n-hexane, 1
:
1), phthalaldehyde (1 mmol), 2-hydroxy-1,4-naphthoquinone (2 mmol, 0.348 g) or 4-hydroxycumarin (2 mmol, 0.324 g) were added to the reaction mixture, and it was stirred at 89 °C for the time given in Table 5. Then, the reaction mixture was filtered to give the crude product. The solid was washed with EtOH to give pure product 6 or 7 in good to high yield.
4,4′-(1,3-phenylene)bis(2-(methylamino)-3-nitro-4H-benzo[g]chromene-5,10-dione) (6a). Orange solid: mp: 302–305 °C, yield: 0.556 g (86%). 1H NMR (300 MHz, DMSO-d6): δ 3.19 (6H, s, 2CH3), 3.21 (6H, s, 2CH3), 5.24 (2H, s, 2CH), 5.27 (2H, s, 2CH), 7.13–8.07 (24H, m, ArH), 10.28 (4H, m, 4NH). 13C NMR (75 MHz, DMSO-d6): δ 29.0 (4NCH3), 36.6 (2CH), 36.8 (2CH), 107.2 (2C–NO2), 107.4 (2C–NO2), 123.5, 124.5, 125.0, 125.1, 126.5, 127.2, 129.5, 130.7, 131.2, 134.9, 135.1, 141.3, 147.5, 147.8, 157.4, 157.5, 176.5 (4C
O), 182.2 (2C
O), 182.3 (2C
O).
4,4′-(1,4-phenylene)bis(2-(methylamino)-3-nitro-4H-benzo[g]chromene-5,10-dione) (6b). Orange solid: mp: 249–251 °C, yield: 0.543 g (84%). IR (KBr) (νmax/cm−1): 3613, 1667, 1467, 1363, 1245, 1192. MS (EI, 70 eV): m/z (%) = 390 (57), 361 (10), 333 (16), 84 (66), 66 (100). 1H NMR (300 MHz, DMSO-d6): δ 3.15–3.23 (12H, m, 4CH3), 5.23 (2H, s, 2CH), 5.28 (2H, s, 2CH), 7.01–8.04 (24H, m, ArH), 10.23–10.35 (4H, m, 4NH). 13C NMR (62 MHz, DMSO-d6): δ 29.9 (4NCH3), 37.4 (2CH), 38.1 (2CH), 108.5 (2C–NO2), 124.2, 126.9, 127.5, 129.0, 129.4, 129.8, 131.4, 131.9, 132.4, 133.7, 134.3, 135.9, 139.1, 141.3, 148.9, 158.7, 177.6 (2C
O), 182.8 (2C
O), 183.5 (2C=O), 184.7 (2C
O).
4,4′-(1,4-phenylene)bis(3-nitro-2-(propylamino)-4H-benzo[g]chromene-5,10-dione) (6c). Orange solid: mp: 264–266 °C, yield: 0.569 g (81%). IR (KBr) (νmax/cm−1): 3457, 1678, 1522, 1380, 1233, 1146. MS (EI, 70 eV): m/z (%) = 390 (100), 361 (12), 333 (24), 276 (25), 246 (81), 174 (20), 143 (37), 104 (58), 68 (55). 1H NMR (300 MHz, DMSO-d6): δ 0.80 (12H, t, 4CH3), 1.50–1.61 (8H, m, 4CH2), 2.75–2.80 (8H, m, 4CH2), 5.44 (4H, s, 4CH), 7.53–7.86 (24H, m, Ar), 8.80 (2H, br s, 2NH), 9.94 (2H, br s, 2NH).
4,4′-(1,4-phenylene)bis(2-(isopropylamino)-3-nitro-4H-benzo[g]chromene-5,10-dione) (6d). Orange solid: mp: 281–283 °C, yield: 0.555 g (79%). 1H NMR (300 MHz, DMSO-d6): δ 1.10–1.23 (24H, m, 8CH3), 4.30 (4H, m, 4CH), 5.51 (4H, s, 4CH), 7.38–7.87 (24H, m, Ar), 8.80 (2H, br s, 2NH), 9.94 (2H, br s, 2NH).
4,4′-(1,4-phenylene)bis(2-(ethylamino)-3-nitro-4H-benzo[g]chromene-5,10-dione) (6e). Orange solid: mp: 279–281 °C, yield: 0.505 g (75%). 1H NMR (300 MHz, DMSO-d6): δ 1.12 (12H, t, 3JHH = 7.5 Hz, 4CH3), 2.80–2.90 (8H, m, 4CH2), 5.45 (4H, s, 4CH), 7.50–8.87 (24H, m, Ar), 8.80 (2H, br s, 2NH), 9.65 (2H, br s, 2NH). 13C NMR (62 MHz, DMSO-d6): δ 12.4 (2CH3), 13.9 (2CH3), 36.2 (2CH2), 36,4 (2CH2), 37.0 (2CH), 38.0 (2CH), 111.0 (4C–NO2), 126.5, 127.1, 128.3, 128.7, 129.1, 130.7, 131.7, 132.4, 133.2, 134.5, 135.1, 136.1, 177.0 (2C
O), 183.7 (2C
O), 184.4 (2C
O), 185.0 (2C
O).
4,4′-(1,3-phenylene)bis(2-(methylamino)-3-nitropyrano[3,2-c]chromen-5(4H)-one) (7a). White solid: mp: 312–314 °C, yield: 0.541 g (87%). IR (KBr) (νmax/cm−1): 3610, 1729, 1674, 1458, 1364, 1266, 1160. MS (EI, 70 eV): m/z (%) = 256 (6), 200 (12), 136 (11), 96 (13), 81 (46), 69 (100). 1H NMR (300 MHz, DMSO-d6): δ 3.16–3.29 (12H, m, 4CH3), 5.03 (4H, s, 4CH), 7.13–8.02 (24H, m, Ar), 10.25–10.40 (4H, m, 4NH). 13C NMR (62 MHz, DMSO-d6): δ 30.1 (4NHCH3), 38.4 (4CH), 108.2, 109.0, 113.9, 118.0, 124.1, 126.5, 128.2, 129.3, 129.5, 134.6, 142.6, 153.4, 158.3, 160.5.
4,4′-(1,4-phenylene)bis(2-(methylamino)-3-nitropyrano[3,2-c]chromen-5(4H)-one) (7b). White solid: mp: 325–327 °C, yield: 0.566 g (91%). 1H NMR (300 MHz, DMSO-d6): δ 3.25–3.32 (12H, m, 4CH3), 5.02 (4H, s, 4CH), 7.19–8.02 (24H, m, Ar), 10.30–10.35 (4H, m, 4NH). 13C NMR (62 MHz, DMSO-d6): δ 30.1 (4NCH3), 38.3 (4CH), 109.2, 118.0, 124.2, 126.4, 129.5, 134.5, 141.4, 153.5, 158.3, 160.5.
4,4′-(1,4-phenylene)bis(2-(ethylamino)-3-nitropyrano[3,2-c]chromen-5(4H)-one) (7c). White solid: mp: 290–292 °C, yield: 0.533 g (82%). MS (EI, 70 eV): m/z (%) = 256 (7), 137 (15), 97 (38), 81 (73), 69 (100). 1H NMR (300 MHz, DMSO-d6): δ 1.30 (12H, t, 4CH3), 3.70–3.85 (8H, m, 4CH2NH), 5.02 (4H, s, 4CH), 7.06–7.93 (24H, m, Ar), 10.43 (4H, s, 4NH). 13C NMR (62 MHz, DMSO-d6): δ 16.5 (4CH3), 38.3 (4CH), 38.5 (4CH2), 104.6, 108.1, 109.1, 114.1, 116.8, 118.1, 121.3, 124.0, 125.6, 126.4, 127.9, 129.0, 129.5, 130.5, 132.2, 134.5, 139.0, 141.4, 153.5, 157.8, 160.6, 179.2.
4,4′-(1,4-phenylene)bis(3-nitro-2-propylaminopyrano[3,2-c]chromen-5(4H)-one) (7d). White solid: mp: 297–299 °C, yield: 0.603 g (89%). H NMR (300 MHz, DMSO-d6): δ 0.90 (12H, t, 4CH3), 1.70–1.80 (8H, m, 4CH2), 3.65–3.80 (8H, m, 4CH2NH), 5.03 (4H, s, 4CH), 7.05–7.98 (24H, m, Ar), 10.44 (4H, s, 4NH). 13C NMR (62 MHz, DMSO-d6): δ 12.6 (4CH3), 24.2 (4CH2), 37.3 (4CH), 44.8 (4CH2NH), 104.6, 108.1, 109.1, 114.1, 116.8, 118.1, 121.3, 123.9, 125.6, 126.5, 127.9, 129.0, 129.5, 132.2, 134.5, 141.4, 153.4, 157.9, 160.6, 169.0.
4,4′-(1,4-phenylene)bis(2-(isopropylamino)-3-nitropyrano[3,2-c]chromen-5(4H)-one) (7e). White solid: mp: 313–315 °C, yield: 0.576 g (85%). H NMR (300 MHz, DMSO-d6): δ 1.30–1.40 (24H, d, 8CH3), 4.45–4.60 (4H, m, 4CHNH), 5.02 (4H, s, 4CH), 7.19–7.95 (24H, m, Ar), 10.10–10.25 (4H, m, 4NH). 13C NMR (62 MHz, DMSO-d6): δ 23.8 (8CH3), 38.2 (4CH), 46.4 (4CHNH), 104.6, 114.1, 116.8, 118.1, 121.5, 124.2, 125.6, 127.9, 129.0, 129.6, 130.7, 132.2, 134.6, 153.4, 153.9, 157.4, 160.6, 169.0.
Conclusion
In summary, a simple and catalyst free method for the synthesis of 4,4′-(1,4-phenylene)bis(2-(alkylamino)-3-nitro-4H-benzo[g]chromene-5,10-dione) and 4,4′-(1,4-phenylene)bis(2-(alkylamino)-3-nitropyrano[3,2-c]chromen-5(4H)-one) derivatives was reported for the first time. These compounds are obtained from a one-pot, multi-component reaction between various amines, 1,1-bis(methylthio)-2-nitroethene, terephthalaldehyde or isophthalaldehyde, and 2-hydroxy-1,4-naphthoquinone or 4-hydroxycoumarin in EtOH/H2O (85
:
15) at 89 °C. After optimization of reaction conditions by response surface methodology (RSM) all the products could be obtained in good yields (from 75% to 91%). The key advantages of this new synthetic route are atom economy, environmental friendliness, simple operational process and the non-chromatographic purification of products. This study has shown that the experimental results are in good agreement with the predicted values, and the model successfully can be used to predict the synthesis of such derivatives. Further studies on the synthetic application and physical properties examination are currently ongoing.
Conflicts of interest
The authors declare no competing financial interest.
Acknowledgements
We acknowledge the financial support of this research from Imam Khomeini International University. We thank the Iran National Science Foundation (No. 97021367) for financial support.
Notes and references
- A. Aydar, Utilization of Response Surface Methodology in Optimization of Extraction of Plant Materials, in Statistical Approaches with Emphasis on Design of Experiments Applied to Chemical Processes, 2018, pp. 157–169 Search PubMed.
- S. S. A. Amr, H. A. Aziz and M. J. K. Bashir, Appl. Water Sci., 2014, 4, 231–239 CrossRef.
- A. Morshedi and M. Akbarian, Indian Journal of Fundamental and Applied Life Sciences, 2014, 54, 2434–2439 Search PubMed.
- P. Pan, W. Jin, X. Li, Y. Chen, J. Jiang, H. Wan and D. Yu, PLoS One, 2018, 13, 1–14 Search PubMed.
- H. Tang, Q. Xiao, H. Xu and Y. Zhang, Org. Process Res. Dev., 2013, 17, 632–640 CrossRef CAS.
- N. P. T. Nhan, T. T. Hien, L. T. H. Nhan, P. N. Q. Anh, L. T. Huy, N. T. C. Trinh, D. T. Nguyen and L. G. Bach, Metallurgy Technology and Materials VI, Solid State Phenomena, 2018, 279, pp. 235–239 Search PubMed.
- H. V. Srikanth, J. Venkatesh and S. Godiganur, Biofuel, 2018, 3, 1–11 Search PubMed.
- M. A. Bezerra, R. E. Santelli, E. P. Oliveira, L. S. Villar and L. A. Escaleira, Talanta, 2008, 76, 965–977 CrossRef CAS PubMed.
- T. A. Wani, A. Ahmad, S. Zargar, N. Y. Khalil and I. A. Darwish, Chem. Cent. J., 2012, 6, 134–142 CrossRef CAS PubMed.
- H. Aslani, R. Nabizadeh, S. Nasseri, A. Mesdaghinia, M. Alimohammadi, A. H. Mahvi, N. Rastkari and S. Nazmara, Desalin. Water Treat., 2016, 57, 25317–25328 CrossRef CAS.
- J. Palaniraja, S. M. Roopan and G. M. Rayalu, RSC Adv., 2016, 6, 24610–24616 RSC.
- Y. He, J. Cai and Y. Zhang, J. Chem. Eng. Mater. Sci., 2017, 5, 155–167 CAS.
- L. M. Ramos, M. O. Rodrigues and B. A. D. Neto, Org. Biomol. Chem., 2019, 17, 7260–7269 RSC.
- S. Samai, G. C. Nandi, R. Kumar and M. S. Singh, Tetrahedron Lett., 2009, 50, 7096–7098 CrossRef CAS.
- J. Wiemann, L. Fischer, J. Kessler, D. Strohl and R. Csuk, Bioorg. Chem., 2018, 81, 567–576 CrossRef CAS PubMed.
- H. G. O. Alvim, J. R. Correa, J. A. F. Assumpcao, W. A. da Silva, M. O. Rodrigues, J. L. de Macedo, M. Fioramonte, F. C. Gozzo, C. C. Gatto and B. A. D. Neto, J. Org. Chem., 2018, 83, 4044–4053 CrossRef CAS PubMed.
- M. Konstantinidou, K. Kurpiewska, J. Kalinowska-Tluscik and A. Domling, Eur. J. Org. Chem., 2018, 2018, 6714–6719 CrossRef CAS.
- X. del Corte, E. M. de Marigorta, F. Palacios and J. Vicario, Molecules, 2019, 24, 2951–2961 CrossRef PubMed.
- E. M. de Marigorta, J. M. de los Santos, A. M. O. de Retana, J. Vicario and F. Palacios, Synthesis, 2018, 50, 4539–4554 CrossRef.
- S. Yu, R. Hua, X. Fu, G. Liu, D. Zhang, S. Jia, H. Qiu and W. Hu, Org. Lett., 2019, 21, 5737–5741 CrossRef CAS.
- J. Zheng, M. He, B. Xie, L. Yang, Z. Hu, H. B. Zhou and C. Dong, Org. Biomol. Chem., 2018, 16, 472–479 RSC.
- F. Khorami and H. R. Shaterian, Res. Chem. Intermed., 2015, 41, 171–3191 CrossRef.
- F. Yang, H. Wang, L. Jiang, H. Yue, H. Zhang, Z. Wang and L. Wang, RSC Adv., 2015, 5, 5213–5216 RSC.
- M. Sabbaghan, I. Yavari and Z. Hossaini, Comb. Chem. High Throughput Screening, 2010, 13, 813–817 CrossRef CAS PubMed.
- J. Khalafy, S. Ilkhanizadeh and M. Ranjbar, J. Heterocycl. Chem., 2018, 55, 951–956 CrossRef CAS.
- A. Upadhyay, V. K. Singh, R. Dubey, N. Kumar, L. K. Sharma and R. K. P. Singh, Tetrahedron Lett., 2017, 58, 4323–4327 CrossRef CAS.
- T. V. Sal’nikova, M. V. Dmitriev and A. N. Maslivets, Russ. J. Org. Chem., 2018, 54, 652–653 CrossRef.
- A. Shaabani, R. Ghadari, A. Sarvary and A. H. Rezayan, J. Org. Chem., 2009, 74, 4372–4374 CrossRef CAS PubMed.
- L. Z. Fekri, M. Nikpassand, S. Pourmirzajani and B. Aghazadeh, RSC Adv., 2018, 8, 22313–22320 RSC.
- S. J. Roudbaraki, S. S. Mansoor and M. Ghashang, Polycyclic Aromat. Compd., 2019, 39, 1–8 CrossRef.
- S. Chehab, Y. Merroun, T. Ghailane, R. Ghailane, S. Boukhris and A. Souizi, J. Turk. Chem. Soc., Sect. A, 2018, 5, 355–370 CAS.
- J. S. Ghomi, F. Eshteghal and M. A. Ghasemzadeh, Acta Chim. Slov., 2014, 61, 703–708 Search PubMed.
- M. Stępień, E. Gońka, M. Żyła and N. Sprutta, Chem. Rev., 2017, 117, 3479–3716 CrossRef PubMed.
- S. Qin, B. Chen, J. Huang and Y. Han, New J. sChem., 2018, 42, 12766–12772 RSC.
- A. N. Nadaf and K. Shivashankar, Synth. Commun., 2018, 48, 809–815 CrossRef CAS.
- A. Monfared and Z. Esmaeeli, Iran. J. Pharm. Res., 2016, 15, 343–367 CAS.
- L. Kovacikova, R. Gasparova, A. Bohac, M. Durana and M. Lacova, ARKIVOC, 2010, 9, 188–203 Search PubMed.
- A. M. Shahi, M. Nikpassand, H. F. B. Shaidaei and L. Z. Fekri, J. Heterocycl. Chem., 2019, 56, 1413–1419 CrossRef.
- G. M. Ziarani, A. Badiei, M. Azizi and P. Zarabadi, Iran. J. Chem. Chem. Eng., 2011, 30, 59–65 Search PubMed.
- S. A. Fouad, S. A. Hessein, S. Y. Abbas, A. M. Farrag and Y. A. Ammar, Croat. Chem. Acta, 2018, 91, 99–107 CrossRef CAS.
- G. Zhu, Z. Yi, J. Zhou, Z. Chen, P. Guo, Y. Huang, J. Chen, H. Song and W. Yi, ACS Omega, 2018, 3, 13494–13502 CrossRef CAS PubMed.
- I. Zemanova, M. Potancokova and R. Gasparova, Nova Biotechnol. Chim., 2016, 15, 85–89 CAS.
- T. Clarina, G. R. P. Dharsini and V. Rama, Chem. Sci. Trans., 2017, 6, 523–534 CAS.
- R. Ghahremanzadeh, T. Amanpour and A. Bazgir, J. Heterocyclic Chem., 2009, 46, 1266–1270 CrossRef CAS.
- M. Miao, M. Jin, H. Xu, P. Chen, S. Zhang and H. Ren, Org. Lett., 2018, 20, 5718–5722 CrossRef CAS PubMed.
- S. Nasri and M. Bayat, J. Mol. Struct., 2018, 1164, 77–83 CrossRef CAS.
- D. P. Sangi, J. L. Monteiro, K. L. Vanzolini, Q. B. Cass, M. W. Paixaoa and A. G. Correa, J. Braz. Chem. Soc., 2014, 25, 887–889 CAS.
- L. Pan, X. Bi and Q. Liu, Chem. Soc. Rev., 2013, 42, 1251–1286 RSC.
- M. Afsharnezhad, M. Bayat and F. S. Hosseini, Mol. Diversity, 2019, 1–11 Search PubMed.
- K. M. Wang, S. J. Yan and J. Lin, Eur. J. Org. Chem., 2014, 2014, 1129–1145 CrossRef CAS.
- P. H. Yang, Res. Chem. Intermed., 2016, 42, 5617–5637 CrossRef CAS.
- K. M. Wang, S. J. Ya and J. Lin, Eur. J. Org. Chem., 2014, 2014, 1129–1145 CrossRef CAS.
- P. H. Yang, Res. Chem. Intermed., 2016, 42, 5617–5637 CrossRef CAS.
- F. S. Hosseini and M. Bayat, Curr. Org. Synth., 2018, 15, 1–7 CrossRef.
- S. C. Cira, A. Dag and A. Karakus, Adv. Mater. Sci. Eng., 2016, 2016, 1–13 CrossRef.
Footnote |
† Electronic supplementary information (ESI) available. See DOI: 10.1039/c9ra07809f |
|
This journal is © The Royal Society of Chemistry 2019 |
Click here to see how this site uses Cookies. View our privacy policy here.