DOI:
10.1039/C9RA07109A
(Paper)
RSC Adv., 2019,
9, 40397-40403
Ultrahigh permeance of a chemical cross-linked graphene oxide nanofiltration membrane enhanced by cation–π interaction†
Received
5th September 2019
, Accepted 29th October 2019
First published on 6th December 2019
Abstract
Cross-linking with large flexible molecules is a common method to improve the stability and control the interlayer spacing of graphene oxide (GO) membranes, but it still suffers from the limitation of low water flux. Herein, a novel high flux GO membrane was fabricated using a pressure-assisted filtration method, which involved a synergistic chemical cross-linking of divalent magnesium ions and 1,6-hexanediamine (HDA) on a polyethersulfone (PES) support. The membrane cross-linked with magnesium ions and HDA (GOHDA–Mg2+) exhibited a high water flux up to 144 L m−2 h−1 bar−1, about 7 times more than that of cross-linked GO membranes without adding magnesium ions (GOHDA), while keeping excellent rejection performance. The GOHDA–Mg2+ membrane also showed an outstanding stability in water for a long time. The effects of magnesium ions on the GOHDA–Mg2+ membrane were analyzed using several characterization methods, including Fourier transform infrared spectroscopy (FT-IR), scanning electron microscopy (SEM), X-ray photoelectron spectroscopy (XPS) and X-ray diffraction (XRD). The results indicated that magnesium ions not only promoted reasonable cross-linking, but also improved the stacking of GO sheets to give lower mass transfer resistance channels for water transport in the membranes, resulting in the ultrahigh permeance of the GO membranes.
Introduction
Graphene oxide (GO) is a two-dimensional network with a thickness of one atom, which has great potential in the field of water treatment because of its excellent hydrophilicity,1 remarkable stacking property2 and other unique properties.3 In GO membranes, a large number of oxygen-containing functional groups, such as hydroxyl, epoxy, carboxyl and carbonyl, are randomly distributed outside the pristine graphitic sp2 region.4 These oxygen functional groups act as water nanochannel spacers to introduce water molecules into the sp2 region which can allow water molecules to flow without resistance.5 Therefore, GO-based membranes are considered as the next generation of nanofiltration membranes.
In the practical application of GO nanofiltration membranes, the interlayer spacing between the neighboring GO nanosheets, water flux, efficient rejection and stable performance of the membrane are all crucial in water purification.6,7 However, the GO membrane is prone to swelling in water due to the large number of hydrophilic oxygen functional groups, which is not conducive to the stable performance of GO membrane in practical application.8 There have been many effective efforts to stabilize the interlayer spacing and prevent the swelling tendency of GO membranes. For example, by cross-linking with organic large molecules and ions,6,9–11 as well as by reducing the GO membrane to decrease the interlayer spacing, the stability is significant improved.7,12 Despite the great progress, these membranes still suffer from limitation of low water flux (<27 L m−2 h−1 bar−1).2,13,14 The decreasing of the interlayer spacing and excessive cross-linking between sheets, not only decrease the water channel,7,10,11 but also increase the mass transfer resistance,9 leading to the low permeance. Therefore, GO membranes is still under the expectation, which requires further increase of water flux without sacrificing stability and rejection.2 These challenges hinder the potential applications of GO membranes in water purification.
In our previous work, accurate cationic control of the interlayer spacing of GO membranes with Ångström precision using ions, has been achieved.11 The existence of cations adsorbed on GO surface, can greatly improve the flatness of the GO nanosheets, which is conducive to the stacking of GO sheets to form reasonable water nanochannels.11,15 Therefore, chemical cross-linking together with ions, potentially promoting reasonable cross-linking and improving the water channels of membrane in terms of flatness and surface with low mass transfer resistance.
For Mg2+, it is the most divalent cation and abundant in seawater, which has the same strong cation–π interaction with the graphene sheets as the high multivalence metal ions (Fe2+, Co2+, Cu2+, Cd2+, Cr2+ and Pb2+) have.16 Interestingly, GO membranes controlled by Mg2+ ions have the largest interlayer spacing compared with other metal ions in seawater.11 The large interlayer spacing in GO membrane is the prerequisite for ultrahigh water permeation. However, due to strong cation–π interaction between Mg2+ and GO flakes, Mg2+ ions can be adsorbed on the GO surface during the cross-linking process, which can prevent excessive chemical cross-linking and improve the water channels of membrane in terms of flatness and low mass transfer resistance surface during the cross-linking reaction.
In this study, the GO membrane with high water permeance for dyes rejection was prepared by pressure-assisted filtration method, which was a synergistic chemical cross-linking of divalent magnesium (Mg2+) ions and hexamethylenediamine (HDA) (GOHDA–Mg2+) on a polyethersulfone (PES) support. We also prepared the cross-linking GO membrane only by HDA for comparison (GOHDA).
Experimental
Preparation graphene oxide (GO) suspension
Graphene oxide (GO) was prepared from commercial graphite powder by a modified Hummers' method as previously reported.11,17 Graphite powders were firstly pre-oxidized by concentrated H2SO4, K2S2O8, and P2O5 solution with continuous stirring for 4.5 hours. Secondly the mixture suspension was washed by DI water and vacuum drying for a night. Then, oxide graphite was further oxidized in concentrated H2SO4 and KMnO4, diluted with a lot of DI water. 30% H2O2 is further trickled to remove excess KMnO4. The product was separated by centrifugation and washed with 1
:
10 HCl aqueous solution and DI water. Finally, the GO suspension was prepared after half an hour of ultrasound.
Preparation cross-linking GO membrane
MgCl2 were added into 150 mg L−1 GO and stirred evenly. The concentration of Mg2+ in the mixed suspension was 0.25 M, 0.125 M, 0.05 M, 0.025 M and 0 M, respectively. Next, 1,6-hexanediamine was dissolved in the above mixed suspension to prepared 0.075 M aqueous solution. The mixtures were left to rest overnight at room temperature, 3 mL solution was dissolved to 40 mL, respectively. Then, they were filtered through a polyethersulfone (PES) membrane under a pressure of 1 bar. Finally, the membranes were washed with 1
:
10 HCl aqueous solution and DI water. The membranes prepared at a series of concentrations of Mg2+ were named as GOHDA–0.25Mg2+, GOHDA–0.125Mg2+, GOHDA–0.05Mg2+, GOHDA–0.025Mg2+ and GOHDA, respectively. GOHDA–0.25Mg2+, GOHDA–0.125Mg2+, GOHDA–0.05Mg2+ and GOHDA–0.025Mg2+ are collectively referred to as GOHDA–Mg2+.
Filtration experiment
The permeation and rejection performance of the cross-linking membranes were tested by using a vacuum filter system with an effective membrane area of 11.34 cm2. The rejection tests were performed with 10 mg mL−1 rhodamine B (RB), pararosaniline (PR), and methyl blue (MB) solutions, respectively. The molecular weights of the three dyes are 479.01, 323.82 and 319.86 g mol−1, respectively. When filtration went steady, the water flux (Jw) and Rejection (R) was measured at 1 bar by using the following eqn (1) and (2): |
 | (1) |
|
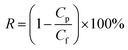 | (2) |
where Jw is the water flux (L m−2 h−1 bar−1), V is the volume of the permeation water (L), A is the effective membrane area (m2). Δt is the permeation time (h) and the P is the filtration pressure (bar). Cp and Cf are the concentration of permeation and feed dye solution which were measured by ultraviolet spectrophotometry, respectively.
Results and discussion
The chemical properties of GO and cross-linking GO membrane
The FT-IR spectra of the GO, GOHDA–0.25Mg2+ and GOHDA are shown in Fig. 1b. The chemical structure of GO was clearly changed by HDA. The FTIR spectrum of the pristine GO suggested the presence typical vibrations, such as, the hydroxyl C–OH (stretching at 3594 cm−1), carbonyl C
O (stretching at 1730 cm−1), carboxyl –OH (bending at 1418 cm−1), aromatic (stretching vibrations at 1622 cm−1) and epoxy C–O (stretching at 1020–1227 cm−1).18–21 For GOHDA–0.25Mg2+ and GOHDA, the peaks of hydroxyl, epoxy and carboxyl decreased dramatically after cross-linking with HDA, and a new absorption peak was observed at 1550 cm−1 which represent the bending vibration of N–H.9,10,22 The reduction of hydroxyl, epoxy and carboxyl groups and the new generation of amine confirmed that HDA likely reacts with these oxygen-containing groups to form C–N covalent bonds, during the condensation reaction of HDA with hydroxyl23 and carboxyl24 and the nucleophilic addition reaction of amine with epoxy.24 In addition, compared with the GOHDA, the GOHDA–0.25Mg2+ has more oxygen-containing groups and less amine groups, indicating a weaker reduction and lower degree of cross-linking due to Mg2+ added in the reaction process.
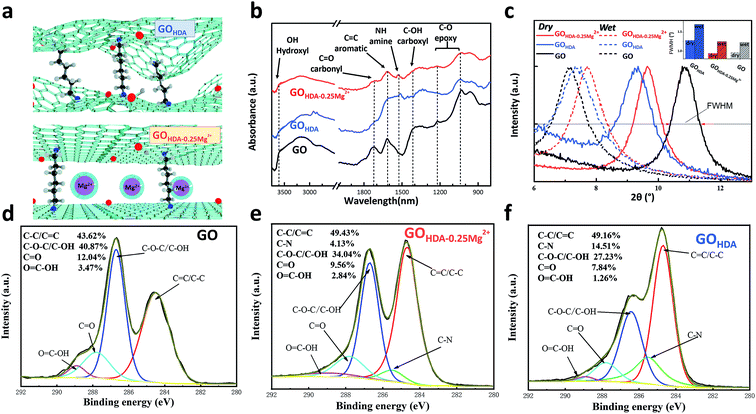 |
| Fig. 1 (a) A schematic of GOHDA–0.25Mg2+ and GOHDA; (b) FTIR spectra of GO, GOHDA–0.25Mg2+ and GOHDA; (c) XRD spectra of GO, GOHDA–0.25Mg2+ and GOHDA; X-ray photoelectron spectrometer spectra of (d) GO, (e) GOHDA–0.25Mg2+ and (f) GOHDA. | |
To further reveal the chemical properties of GO membranes, C 1s of XPS spectra were used to analyze the elemental compositions of the chemical bonds. As shown in Fig. 1d–f, the deconvoluted C 1s spectra were divided into four peaks at binding energies of 284.6, 286.7, 287.8, 288.9 eV, which corresponded with C
C/C–C, C–O/C–O–C, C
O and O
C–O, respectively.7,25,26 The contents of C–O–C/C–O were 40.87%, 34.04% and 27.23% for GO, GOHDA and GOHDA–0.25Mg2+ respectively, which significantly decreased by cross-linking. And the intensities of O
C–O were also decreased dramatically. Importantly, a new peak appears at 285.5 eV representing the C–N bond,9,10 which are 4.13% and 14.51% for GOHDA–0.25Mg2+ and GOHDA, respectively. The results demonstrated that the GOHDA–0.25Mg2+ has an effective cross-linking similar to GOHDA, while a weaker reduction and lower degree of cross-linking, which is consistent with our FT-IR spectra results. It indicates that under the interaction of Mg2+, it is beneficial to reasonable cross-linking between GO and HDA. It not only ensures the stability of the membrane, but also facilitates the formation of channels with low mass transfer resistance.
In addition, we used XPS to detect the atomic concentrations. Fig. S2b† shows the survey XPS scans of the prepared cross-linked membranes. We can see that there are no observable Mg2+ ion signals. During the filtration, the filtrates were collected when the filtration process went steady (after about 20 min), which can help to rule out the adsorption effect by the membrane. Thus, the high rejection for dyes remained constant with increasing membrane thickness, is mainly due to stable size exclusion effect based on stable chemical cross-linking with HDA and the water channels of membrane improved by Mg2+ during the cross-linking reaction.
Effects of Mg2+ on the interlayer spacing of cross-linked GO
As mentioned above, the water channel of membrane is an important parameter for permeation. These membranes were further analyzed by XRD. There were clear shifts of the interlayer spacing (indicated by the Bragg peaks of XRD) relative to the GO membrane that had been immersed in pure water, as shown in Fig. 1c. Immersion in pure water resulted in a GO membrane spacing from 8.5 Å to 12.8 Å, consistent with early reports.11 In contrast, the shifts of interlayer spacing of GOHDA and GOHDA–0.25Mg2+ between dry and wet state were smaller. The interlayer spacing of GOHDA were 9.3 Å and 11.9 Å in dry and wet state, respectively, due to the limitation of newly formed C–N bonds between the GO sheets.9,10 Similarly, those of GOHDA–0.25Mg2+ in dry and wet state were 9.1 Å and 11.4 Å. However, as shown in the inset of Fig. 1c, the full width at half maxim (FWHM) of GOHDA–0.25Mg2+ is obviously narrower than that of GOHDA, indicating that GOHDA–0.25Mg2+ has better uniformity of the water channel, than that of the GOHDA membrane. Thus, the channel can be shown in the schematic of Fig. 1a. The rippling and wrinkled structure was aligned flattened by Mg2+, which is conducive to the stacking of GO sheets to form a surface with low mass transfer resistance for water transport.11,15 Therefore, it can be predicted that the GOHDA–0.25Mg2+ membrane has a relatively high permeance while maintaining high rejection.
Morphology of the GO and cross-linking GO
The Atomic Force Microscope (AFM) image of GO flakes was observed, as shown in Fig. S2a.† The thickness of GO monolayer is about 0.96 nm. Fig. 2a–f show the SEM images of surface and cross-section morphology of the GO, GOHDA and GOHDA–0.25Mg2+ membranes. The thickness of the cross-linked membrane was about 200 nm, while the thickness of GO membrane was 150 nm. These SEM images showed that the resulting thin layered membrane was continuous and free of macro pores or defects, which is critical for a highly efficient separation process.11 As shown in Fig. 2f, GOHDA–0.25Mg2+ membrane is obviously a multi-layers structure like GO membrane. As shown in Fig. 2g, we also evaluated the stability of the membrane immersed in water. The GO membrane disintegrated after 20 min without mechanical stirring, and then seriously dispersed after 60 min. But GOHDA–0.25Mg2+ and GOHDA still remain stable, even when the water was stirred by glass rod, indicating that GOHDA–0.25Mg2+ membrane can overcome the swelling problem in water like GOHDA.
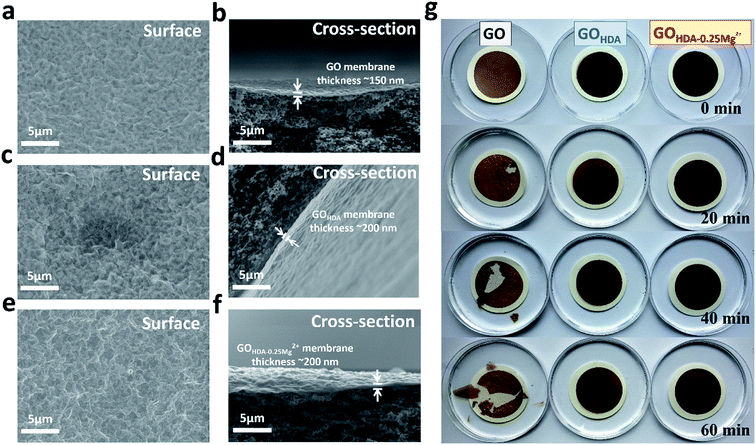 |
| Fig. 2 (a) Surface morphology and (b) cross-section morphology of GOHDA–0.25Mg2+. (c) Surface morphology and (d) cross-section morphology of GO. (e) Surface morphology and (f) cross-section morphology of GOHDA. (g) Stability of GO, GOHDA and GOHDA–0.25Mg2+ membranes in water. These pictures were taken after the water had been stirred with a glass stirring rod. | |
Permeance of cross-linking GO
We performed dyes permeation tests to verify the water flux and the reject rate of GO, GOHDA and GOHDA–0.25Mg2+ membranes. As shown in Fig. 3a–c, HDA resulted in a decrease in water flux for rejecting methylene blue, pararosaniline and rhodamine B from 12.5 L m−2 h−1 bar−1, 30.6 L m−2 h−1 bar−1 and 23.5 L m−2 h−1 bar−1 for GO membrane to 11.2 L m−2 h−1 bar−1, 10.8 L m−2 h−1 bar−1 and 13.4 L m−2 h−1 bar−1 for the GOHDA membrane, respectively. But it didn't make much difference to the reject rate. The performance of GOHDA is consistent with early reports.9,10 In addition, we also observed the performance of the GO membranes controlled only by Mg2+ (GOMg2+), which prepared with the same experimental process as did the cross-linking experiments, as shown in Fig. S3.† The water flux of GOMg2+ for rejecting methylene blue was 39 L m−2 h−1 bar−1, which is only slightly higher than that of GO. In contrast, GOHDA–0.25Mg2+ membranes have ultrahigh water flux, which were 143.2, 114.4 and 144.2 L m−2 h−1 bar−1 for the three dyes, respectively, while still rejected dyes as those of GOHDA. Interestingly, the water fluxes are nearly 10 times higher than those of GOHDA membranes without sacrificing dyes rejection, as shown in Fig. 3d. It further demonstrated that GOHDA–0.25Mg2+ membrane has much better uniformity and lower mass transfer resistance than that of GOHDA. We also listed the separation performance of GO-based membranes previously reported for organic dyes. As shown in Table 1, GOHDA–0.25Mg2+ showed great advantage on the water flux.
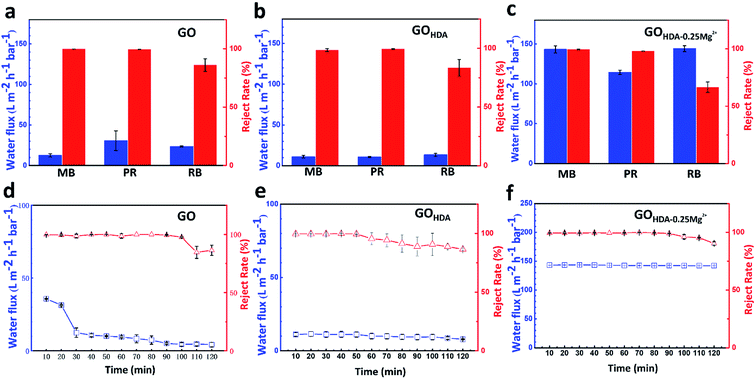 |
| Fig. 3 Permeance and rejection of three organic dyes (10 mg L−1) of (a) GOHDA–0.25Mg2+, (b) GO and (c) GOHDA membranes at a transmembrane pressure of 1.0 bar. Flux of the (d) GOHDA–0.25Mg2+, (e) GO and (f) GOHDA membranes during the filtration of water for 120 min. | |
Table 1 Permeation of graphene-based membrane for dyes rejectiona
Membrane |
Dyes |
Reject rate (%) |
Permeance(L m−2 h−1 bar−1) |
Reference |
CCG: chemically converted graphene; PECs: polyelectrolyte complexes; TMC: 1,3,5-benzenetricarbonyl trichloride; MCNT: multi-walled carbon nanotube; NSC-GO: ultrafiltration nanostrand-channelled GO. |
CCG |
Methylene blue |
>99 |
3.26–21.81 |
12 |
GO + PECs |
Methylene blue |
99.3 ± 0.1 |
0.87 ± 0.02 |
27 |
TMC + GO |
Methylene blue |
46–66 |
8–27.6 |
14 |
rGO/MCNT |
Rhodamine B |
100 |
52.7 |
28 |
NSC-GO |
Rhodamine B |
87 ± 3 |
279 |
29 |
GO |
Pararosaniline |
86.48–98.88 |
11.13–20.23 |
30 |
Methylene blue |
90.15–98.97 |
GOHDA–0.25Mg2+ |
Methylene blue |
99.42 |
143.2 |
This work |
Pararosaniline |
97.90 |
114.4 |
Rhodamine B |
66.54 |
144.2 |
The stability of water flux and dyes rejection of GOHDA–0.25Mg2+ and GOHDA membranes was analyzed. The fluxes were measured for 2 h and recorded every 10 min after adding DI water into the feed side. As shown in Fig. 3d–f, the fluxes of the GOHDA–0.25Mg2+ and GOHDA were about 114.4 L m−2 h−1 bar−1 and 11.2 L m−2 h−1 bar−1, respectively, which were very stable during the whole filtration process compared with the flux varies from 35.7 L m−2 h−1 bar−1 to 4.3 L m−2 h−1 bar−1 of the GO membrane. It demonstrated that GOHDA–0.25Mg2+ and GOHDA all have the outstanding stability in filtration process, which is attributed to the C–N bond formed between GO and HDA.
The performance of the GO membranes, which were synergistically cross-linked by K+ (GOHDA–K+) or Fe3+ (GOHDA–Fe3+) were also observed, as shown in Fig. S4.† The membrane cross-linked with K+ and Fe3+ ions and HDA (GOHDA–K+ and GOHDA–Fe3+) exhibited water flux of 40.1 L m−2 h−1 bar−1 and 140.6 L m−2 h−1 bar−1, respectively, while keeping >99% rejection for methylene blue, indicating high multivalence metal ions are beneficial to permeability. Compared with these tested metal cations, the Mg2+ ions still have the greatest advantage in terms of water flux.
Effect of thickness and concentration of Mg2+ on permeance of cross-linking GO membrane
It is clear that Mg2+ can greatly improve the permeance of the cross-linking membrane. Considering potential influence of Mg2+ concentration on cross-linking, we prepared GO membranes with a series of concentrations (from 0.25 M to 0.025 M) of Mg2+, which were named as GOHDA–0.25Mg2+, GOHDA–0.125Mg2+, GOHDA–0.05Mg2+, and GOHDA–0.025Mg2+, respectively. As shown in Fig. 4a–c, we used GOHDA–Mg2+ membranes, which have a thickness of about 200 nm, to investigate the permeation with different concentrations of Mg2+. As the concentration of Mg2+ decreased from 0.25 M to 0.025 M, the water fluxes gradually reduced from 142.2 to 71.5 L m−2 h−1 bar−1 for methylene blue, from 114.4 to 70.3 L m−2 h−1 bar−1 for pararosaniline, and from 114.2 to 71.6 L m−2 h−1 bar−1 for rhodamine B, respectively, while the rejections remained constant. In the case of the lowest Mg2+ concentration of 0.025 M, the water fluxes were still about seven times that without Mg2+. It further indicated that Mg2+ plays a key role in improving the water flux of cross-linking GO membrane.
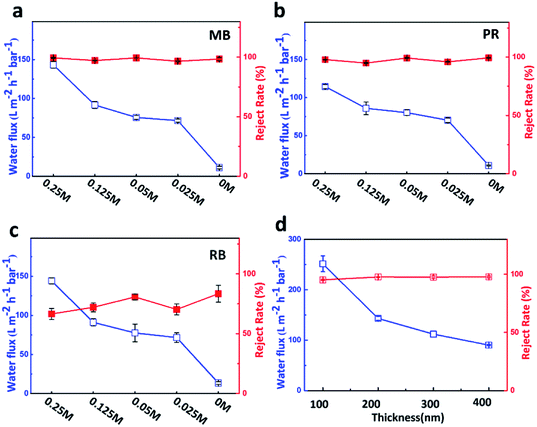 |
| Fig. 4 Permeance and reject rate of (a) methylene blue, (b) pararosaniline and (c) rhodamine B (10 mg L−1) by GOHDA–Mg2+ membrane formed with different Mg2+ concentrations solutions. (d) Permeance and reject rate of methylene blue of GOHDA–0.25Mg2+ membranes with different thickness. | |
In addition, the effect of thickness of membrane on water flux and reject rate were also explored, as shown in Fig. 4d. The thickness of membrane can be controlled by the amount of GO suspension loaded on the substrate. GOHDA–0.25Mg2+ membranes with four thickness of 100 nm, 200 nm, 300 nm and 400 nm, were prepared by using 1.5 mL, 3 mL, 4.5 mL and 6 mL of mixture GO solution. With increasing of the thickness of the membrane, the water flux decreases dramatically from 251.5 to 90.2 L m−2 h−1 bar−1 while the rejection remained constant, suggesting that the highest water flux could be further improved without sacrificing dyes rejection by controlling membrane thickness.
The cation–π interactions between hydrated Mg2+ and GO sheets
To illustrate the underlying physical mechanism of Mg2+ taking place in the process, we performed UV absorption spectra of GO solutions mixed with 0.25 M, 0.125 M, 0.05 M, and 0.025 M Mg2+, respectively. As shown in Fig. 5, the characteristic peak at ∼230 nm was attributed to the π–π* from the aromatic double bond conjugate.13,16,31 Compared with the GO in pure water, the intensity of GO mixed with Mg2+ solution was significantly decreased. It is showed that the trend was positively correlated with the concentration of Mg2+ solution. It indicated that the conjugated double bonds of aromatic groups in GO are greatly influenced by the concentration of salt solution.11 Our previous density functional theory computations show that high multivalence metal ions should have a strong cation–π interaction with the graphene sheet, resulting in ions enrichment on surface of graphene.16 Hence, the enrichment of Mg2+ on GO sheets based on the strong cation–π interaction, can promoted reasonable cross-linking and improved the water channels of membrane in terms of flatness and low mass transfer resistance. As a result, the GOHDA–Mg2+ membrane has ultrahigh water flux, while keeping high dyes rejection.
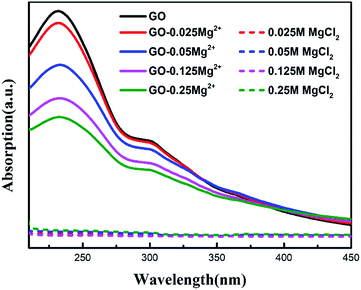 |
| Fig. 5 UV absorption spectra of GO suspension (100 mg L−1) 1 : 1 mixed with 0.025 M, 0.125 M, 0.05 M and 0.25 M Mg2+ solutions. | |
Conclusions
In summary, we successfully enhanced the permeance of the cross-linking GO membrane by using the cation–π interaction between Mg2+ and aromatic ring structure. The enhancement is positively correlated with the concentration of Mg2+. It not only overcomes the swelling of GO membrane and enhances the stability of membrane, but also solves limitation of low water flux. This is attributed to the presence of Mg2+, which can prevent excessive chemical cross-linking and improves the water channels of membrane in terms of flatness and low mass transfer resistance surface during the cross-linking reaction. Therefore, the GOHDA–0.25Mg2+ exhibits ultrahigh water flux (143.2 L m−2 h−1 bar−1) and high reject rate for dyes in the filtration experiment. The GOHDA–Mg2+ membranes also have an outstanding stability in filtration process, which is attributed to the C–N bond formed between GO and HDA. This study suggests that other ions that have strong cation–π interaction, could have a similar effect like Mg2+, may opens a new door for the chemical cross-linking mode of GO membrane combined with ions.
Conflicts of interest
There are no conflicts to declare.
Acknowledgements
This work was supported by the National Natural Science Foundation of China (No. U1832150, 11875236), the Scientific Research and Developed Fund of Zhejiang A & F University (No. 2017FR032).
Notes and references
- V. V. Neklyudov, N. R. Khafizov, I. A. Sedov and A. M. Dimiev, Phys. Chem. Chem. Phys., 2017, 19, 17000–17008 RSC.
- P. Sun, K. Wang and H. Zhu, Adv. Mater., 2016, 28, 2287–2310 CrossRef CAS PubMed.
- N. V. Medhekar, A. Ramasubramaniam, R. S. Ruoff and V. B. Shenoy, ACS Nano, 2010, 4, 2300–2306 CrossRef CAS PubMed.
- Y. Tu, M. Lv, P. Xiu, T. Huynh, M. Zhang, M. Castelli, Z. Liu, Q. Huang, C. Fan, H. Fang and R. Zhou, Nat. Nanotechnol., 2013, 8, 594 CrossRef CAS PubMed.
- B. Mi, Science, 2014, 343, 740 CrossRef CAS PubMed.
- J. Ran, C. Chu, T. Pan, L. Ding, P. Cui, C.-F. Fu, C.-L. Zhang and T. Xu, J. Mater. Chem. A, 2019, 7, 8085–8091 RSC.
- K. H. Thebo, X. Qian, Q. Zhang, L. Chen, H.-M. Cheng and W. Ren, Nat. Commun., 2018, 9, 1486 CrossRef PubMed.
- S. Zheng, Q. Tu, J. J. Urban, S. Li and B. Mi, ACS Nano, 2017, 11, 6440–6450 CrossRef CAS PubMed.
- W.-S. Hung, C.-H. Tsou, M. De Guzman, Q.-F. An, Y.-L. Liu, Y.-M. Zhang, C.-C. Hu, K.-R. Lee and J.-Y. Lai, Chem. Mater., 2014, 26, 2983–2990 CrossRef CAS.
- S. Xia, M. Ni, T. Zhu, Y. Zhao and N. Li, Desalination, 2015, 371, 78–87 CrossRef CAS.
- L. Chen, G. Shi, J. Shen, B. Peng, B. Zhang, Y. Wang, F. Bian, J. Wang, D. Li, Z. Qian, G. Xu, G. Liu, J. Zeng, L. Zhang, Y. Yang, G. Zhou, M. Wu, W. Jin, J. Li and H. Fang, Nature, 2017, 550, 380 CrossRef CAS PubMed.
- Y. Han, Z. Xu and C. Gao, Adv. Funct. Mater., 2013, 23, 3693–3700 CrossRef CAS.
- W. Chen, S. Chen, T. Liang, Q. Zhang, Z. Fan, H. Yin, K.-W. Huang, X. Zhang, Z. Lai and P. Sheng, Nat. Nanotechnol., 2018, 13, 345–350 CrossRef CAS PubMed.
- M. Hu and B. Mi, Environ. Sci. Technol., 2013, 47, 3715–3723 CrossRef CAS PubMed.
- J. Shen, G. Liu, K. Huang, W. Jin, K.-R. Lee and N. Xu, Angew. Chem., 2015, 127, 588–592 CrossRef.
- G. Shi, J. Liu, C. Wang, B. Song, Y. Tu, J. Hu and H. Fang, Sci. Rep., 2013, 3, 3436 CrossRef PubMed.
- W. S. Hummers and R. E. Offeman, J. Am. Chem. Soc., 1958, 80, 1339 CrossRef CAS.
- C. Zhang, D. M. Dabbs, L.-M. Liu, I. A. Aksay, R. Car and A. Selloni, J. Phys. Chem. C, 2015, 119, 18167–18176 CrossRef CAS.
- E. Fuente, J. A. Menéndez, M. A. Díez, D. Suárez and M. A. Montes-Morán, J. Phys. Chem. B, 2003, 107, 6350–6359 CrossRef CAS.
- A. Bagri, C. Mattevi, M. Acik, Y. J. Chabal, M. Chhowalla and V. B. Shenoy, Nat. Chem., 2010, 2, 581 CrossRef CAS PubMed.
- Y. Shen, H.-B. Zhang, H. Zhang, W. Ren, A. Dasari, G.-S. Tang and Z.-Z. Yu, Carbon, 2013, 56, 132–138 CrossRef CAS.
- J. Cai, J. Chen, P. Zeng, Z. Pang and X. Kong, Chem. Mater., 2019, 31, 3729–3735 CrossRef CAS.
- Y. Yang, J. Wang, J. Zhang, J. Liu, X. Yang and H. Zhao, Langmuir, 2009, 25, 11808–11814 CrossRef CAS PubMed.
- N. I. Kovtyukhova, P. J. Ollivier, B. R. Martin, T. E. Mallouk, S. A. Chizhik, E. V. Buzaneva and A. D. Gorchinskiy, Chem. Mater., 1999, 11, 771–778 CrossRef CAS.
- S. H. Shim, K. T. Kim, J. U. Lee and W. H. Jo, ACS Appl. Mater. Interfaces, 2012, 4, 4184–4191 CrossRef CAS PubMed.
- H.-K. Jeong, Y. P. Lee, R. J. W. E. Lahaye, M.-H. Park, K. H. An, I. J. Kim, C.-W. Yang, C. Y. Park, R. S. Ruoff and Y. H. Lee, J. Am. Chem. Soc., 2008, 130, 1362–1366 CrossRef CAS PubMed.
- N. Wang, S. Ji, G. Zhang, J. Li and L. Wang, Chem. Eng. J., 2012, 213, 318–329 CrossRef CAS.
- K. Goh, W. Jiang, H. E. Karahan, S. Zhai, L. Wei, D. Yu, A. G. Fane, R. Wang and Y. Chen, Adv. Funct. Mater., 2015, 25, 7348–7359 CrossRef CAS.
- H. Huang, Z. Song, N. Wei, L. Shi, Y. Mao, Y. Ying, L. Sun, Z. Xu and X. Peng, Nat. Commun., 2013, 4, 2979 CrossRef PubMed.
- L. Chen, J.-H. Moon, X. Ma, L. Zhang, Q. Chen, L. Chen, R. Peng, P. Si, J. Feng, Y. Li, J. Lou and L. Ci, Carbon, 2018, 130, 487–494 CrossRef CAS.
- H. Yorita, K. Otomo, H. Hiramatsu, A. Toyama, T. Miura and H. Takeuchi, J. Am. Chem. Soc., 2008, 130, 15266–15267 CrossRef CAS PubMed.
Footnote |
† Electronic supplementary information (ESI) available. See DOI: 10.1039/c9ra07109a |
|
This journal is © The Royal Society of Chemistry 2019 |
Click here to see how this site uses Cookies. View our privacy policy here.