DOI:
10.1039/C9RA06869D
(Paper)
RSC Adv., 2019,
9, 33755-33760
Effect of salts formed by neutralization for the enzymatic hydrolysis of cellulose and acetone–butanol–ethanol fermentation
Received
29th August 2019
, Accepted 16th October 2019
First published on 21st October 2019
Abstract
Neutralization is essential to maintain the pH for enzymatic hydrolysis of cellulose followed by fermentation of biofuels. This study investigated the effect of salts formed during the neutralization on the enzymatic hydrolysis of cellulosic materials and acetone–butanol–ethanol (ABE) fermentation. The results showed that the formed Ca-citrate salt considerably decreased the glucose release by 26.9% and 26.1% from Avicel and sulfuric acid-pretreated hybrid Pennisetum, respectively, which was probably due to the unproductive adsorption of cellulases by Ca-citrate solids. On the other hand, the formed soluble Na and Ca salts severely inhibited ABE fermentation, thereby decreasing the ABE concentration from 12.8 g L−1 to 0–10.7 g L−1 in different degrees, but no or slight inhibition was observed when the Ca salts formed as precipitates. In particular, Ca-sulfate did not show apparent inhibition of both hydrolysis and fermentation. Therefore, the selection of suitable pretreatment and neutralizing reagents is an alternative way to avoid process inhibition in biofuel production from lignocellulosic materials.
Introduction
Economic growth has naturally led to a rapid rise in energy consumption worldwide. The increasing demand for biofuels has been the impetus for research to produce alcohol biofuels from renewable resources.1,2 Biobutanol with superior fuel properties is considered as an alternative biofuel.3,4 It could be produced through acetone, butanol, and ethanol (ABE) fermentation by Clostridium strains from lignocellulosic materials such as agricultural and forest residues, and energy crops.5–8 Lignocellulose mainly consists of cellulose, hemicellulose, and lignin, which form a complex structure to resist the chemical or microbial degradation. Thus, the conversion of lignocellulosic materials to sugars for ABE fermentation is one of the main technical and economic challenges.
Enzymatic hydrolysis represents the most-efficient method for conversion of lignocellulosic materials to fermentable sugars.9 Pretreatment necessarily disrupts the heterogeneous structure of lignocellulosic materials, removes hemicelluloses and/or lignin, and increases the surface area and porosity of biomass.10,11 Therefore, pretreatment enhances the enzymatic hydrolysis of cellulose for biofuels fermentation.12 To date, a variety of pretreatment technologies have been developed. Dilute acid and alkali pretreatments are the most widely used pretreatment processes, which yield higher amounts of digestible substrates for successful enzymatic hydrolysis.13
After dilute acid or alkali pretreatment, a neutralization step is essential to maintain the required pH for the enzymatic hydrolysis of cellulosic materials followed by fermentation to biofuels. Generally, alkalis (i.e., sodium hydroxide, calcium hydroxide, ammonia, and calcium carbonate) or acids (i.e., sulfuric, acetic, and hydrochloric acids) are used to neutralize the pretreated hydrolysate to pH 5.0 and 6.5 for enzymatic hydrolysis and fermentation, respectively. However, salts, such as Na and Ca salts, formed during the neutralization process, might affect the efficiency of hydrolysis and fermentation. Several studies have shown that soluble salts, including sodium sulfate, sodium acetate, and sodium chloride, were toxic to butanol-producing cultures.14,15 The presence of soluble salts in the fermentation medium inhibits the cell growth of fermentation microbes.16
To the best of our knowledge, few studies discussed the effect of salts formed after neutralization on enzymatic hydrolysis of cellulosic materials. It is also unclear whether insoluble salts have inhibitory effects on ABE fermentation. This study simulated the formation process of salts after sulfuric, acetic, and citric acid pretreatments to investigate the effect of these salts on enzymatic hydrolysis of cellulosic materials and acetone–butanol–ethanol (ABE) fermentation, respectively. The study will provide a scientific basis for adjusting biomass processing conditions to increase the feasibility of biofuel production from lignocellulosic materials.
Experimental
Raw materials
The hybrid Pennisetum used as a lignocellulosic material was provided by the Beijing Research and Development Center for Grass and Environment, Beijing Academy of Agriculture and Forestry Sciences, Beijing, China. The raw materials were milled and sieved through a 80 mesh screen scale. The milled sample was pretreated with 1% (w/w) sulfuric acid with a solid to liquid ratio of 1
:
10 at 121 °C for 1 h in an autoclave (YXQ-LS-50A, Boxun, Shanghai). After pretreatment, the supernatant and solid were separated by centrifugation at 10
000g for 10 min. The pretreated solids were washed and oven-dried at 105 °C for 12 h, and then were used for enzymatic hydrolysis. Microcrystalline cellulose (Avicel PH-101) was purchased from Sigma Chemical Co. (St. Louis, MO, USA). The Avicel and pretreated Pennisetum contained 91.3% and 51.7% cellulose, respectively. The commercial enzymes Celluclast 1.5 L and Novozyme 188 (Novozymes A/S, Bagsværd, Denmark) were used as the cellulase preparations (CEL). Clostridium acetobutylicum DSM 1731 was obtained from DSMZ, Braunschweig Germany (German Collection of Microorganisms and Cell Cultures).
Enzymatic hydrolysis
Hydrolysis with 2% (w/v) Avicel and sulfuric acid-pretreated hybrid Pennisetum was performed in tubes with 3 mL buffer solution in a shaker with stirring at 200 rpm, 50 °C for 48 h. The sodium citrate buffer (50 mM, pH 5.0) was used as control. The citric acid, acetic acid, and sulfuric acid solutions, which were neutralized with NaOH and Ca(OH)2 to pH 5.0, respectively, were also used as buffer for the hydrolysis. Dilute acid pretreatments are usually performed over a temperature range of 120 °C to 210 °C, with acid concentration typically less than 4% (w/w) and residence time from a few seconds to an hour in different types of reactors.13 Thus, the acids loading, 90 mM and 120 mM, were applied according to the studies, in which different dilute organic acids were used for pretreatments.17–19 Prior to the hydrolysis, 22.9 mg protein per g biomass of Celluclast 1.5 L (169.6 mg protein per mL), 18.3 mg protein per g biomass of Novozyme 188 (187.9 mg protein per mL) were added to the slurry for enzymatic hydrolysis. After the hydrolysis, the samples were boiled for 10 min to stop the enzymatic hydrolysis and then centrifuged for 10 min at 10
000g. The supernatant of each sample was collected for glucose analysis.
Enzyme adsorption
The enzyme adsorption experiments were carried out in sodium citrate buffer (50 mM, pH 5.0) with 1% (w/v) consistency of Ca-citrate or Ca-sulfate salts. The samples were incubated with 10–400 mg protein of enzymes per g dry matter for 60 min at 4 °C with magnetic stirring. After adsorption, the solids and liquids were separated by centrifugation at 10
000g for 10 min. The protein concentration was measured with a BCA protein assay kit (Beyotime biotechnology, China). The protein adsorbed was measured by subtracting the protein in supernatant from the total loaded protein.
Fermentation
Freeze-stored culture of Clostridium acetobutylicum DSM 1731 was inoculated to 50 mL of Reinforced Clostridial Medium20 for 14–16 h. Then, 1 mL of active growing cells was inoculated into 50 mL of sterilized pre-fermentation P2 media prepared in a 125 mL screw-capped bottle. The pre-fermentation P2 medium contained glucose 30 g L−1 and yeast extract 1 g L−1. Before inoculation, 0.5 mL each of the filter-sterilized stock solution (buffer: KH2PO4 50 g L−1, K2HPO4 50 g L−1, ammonium acetate 220 g L; mineral: MgSO4·7H2O 20 g L−1, MnSO4·H2O 1 g L−1, FeSO4·7H2O 1 g L−1, NaCl 1 g L; and vitamin: para-aminobenzoic acid 0.1 g L−1, thiamine 0.1 g L−1, biotin 0.001 g L−1) was added into the P2 media. The culture was allowed to grow for approximately 16 h at 37 °C before inoculation into the ABE fermentation media.
ABE fermentation was conducted in a 125 mL screw-capped bottle containing 50 mL glucose medium, in which the original glucose concentration was 50 g L−1. The media containing 90 and 120 mM citric acid, acetic acid, and sulfuric acid were neutralized with NaOH and Ca(OH)2 to pH 6.5, respectively. The media were boiled for 10 min in the water bath and purged with N2 for 2 min to maintain their anaerobic condition, and then sterilized at 121 °C for 15 min. Fermentation started at 37 °C when the media were inoculated into the C. acetobutylicum DSM 1731 culture (10%, v/v). Each of the filter-sterilized stock solutions (buffer, mineral and vitamin) was added to the media before the inoculation. All fermentations were conducted at least twice to ensure the reproducibility.
Chemical analysis
The hydrolysis and fermentation samples were analyzed using nuclear magnetic resonance (NMR). The NMR spectra for quantification of glucose, butanol, acetone and ethanol were recorded on an AVANCE 500 DRX NMR spectrometer equipped with a 5 mm QNP SB probe (Bruker, Billerica, MA, USA). The above-mentioned compounds were identified from routine two-dimensional proton–proton and proton–carbon correlated spectra. Quantitative 1H NMR spectra were collected with water presaturation (zgcppr) using a 90° pulse angle, 48 dB presaturation power, 40 s relaxation delay, and 16 scans at 300 K. Prior to the NMR measurements, 200 L of sample liquid was transferred to a 5 mm NMR tube followed by addition of deuterium oxide (D2O, 275 L) and 3-(trimethylsilyl)-propionic-d4 acid (25 L, 20 mM) in D2O as an internal standard of known concentration.
Calculations
Glucose yields were calculated based on the chemical composition of Avicel and pretreated HP according to eqn (1). Butanol and ABE yields were calculated based on the fermentation products, and the original and residual glucose concentrations in fermentation media according to eqn (2). |
 | (1) |
|
 | (2) |
Results and discussion
Effect of salts on enzymatic hydrolysis
The hydrolysis of Avicel and sulfuric acid-pretreated hybrid Pennisetum in Na-citrate (50 mM) buffer as control and in pure water was conducted (Fig. 1). There were no apparent differences in glucose concentrations after Avicel hydrolysis between Na-citrate buffer and water. However, the glucose concentration with water for hybrid Pennisetum was slightly lower. When the original citric acid concentration was 90 or 120 mM, the Na-citrate formed after neutralization by NaOH did not affect glucose release after hydrolysis. However, Ca-citrate that formed after neutralization by Ca(OH)2 decreased the glucose yields of Avicel and sulfuric acid-pretreated hybrid Pennisetum from 30.6% and 31.8% to 3.7% and 5.7%, respectively (Fig. 1). The glucose yields of these two materials were inhibited by 26.9% and 26.1%, respectively, according to the calculation of decreased percentage of glucose yields compared to control. The concentrations of salts did not make the differences in glucose yields. When sulfuric acid and acetic acid were present, the formed Na-sulfate, Ca-sulfate, Na-acetate, and Ca-acetate salts did not affect the glucose yields after hydrolysis of both Avicel and sulfuric acid-pretreated hybrid Pennisetum (Table 1).
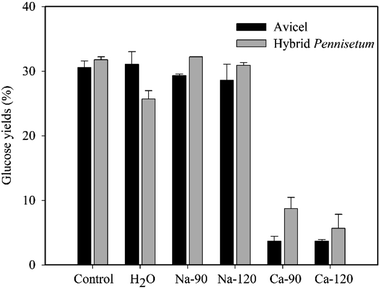 |
| Fig. 1 Glucose concentrations after enzymatic hydrolysis of Avicel and sulfuric acid-pretreated hybrid Pennisetum when Na-citrate and Ca-citrate were present. The 90 and 120 mM represents the concentration of citric acid originally in the hydrolysis solution. | |
Table 1 Glucose concentrations after enzymatic hydrolysis of Avicel and sulfuric acid-pretreated hybrid Pennisetum when Na and Ca salts were present
Salts |
Concentrations (mM) |
Glucose yields (%) |
Avicel |
Hybrid Pennisetum |
H2O |
— |
31.1 ± 2.0 |
25.7 ± 1.3 |
Na-citrate buffer |
50 |
30.6 ± 1.0 |
31.8 ± 0.4 |
Na-sulfate |
90 |
31.8 ± 1.7 |
32.2 ± 0.0 |
120 |
27.9 ± 0.7 |
30.9 ± 0.4 |
Ca-sulfate |
90 |
29.3 ± 0.3 |
32.2 ± 0.0 |
120 |
29.1 ± 1.5 |
31.3 ± 0.0 |
Na-acetate |
90 |
29.1 ± 1.5 |
31.8 ± 1.3 |
120 |
26.4 ± 1.7 |
25.2 ± 3.5 |
Ca-acetate |
90 |
27.6 ± 1.5 |
31.3 ± 0.8 |
120 |
29.3 ± 0.7 |
30.9 ± 0.4 |
Previously, it was reported that in the presence of Na2SO4, K2SO4, CaSO4, and (NH4)2SO4 salts, the enzymatic hydrolysis yields of washed acid-catalyzed steam-exploded corn stover gradually decreased with increasing salts concentrations.21 The inhibition degree of CaSO4 was the highest at 0.06 M, whereas that of (NH4)2SO4 was the lowest.11 The reason was probably due to that the combination of Ca2+ and –SH of the cystine residue in cellulase activity centre inhibited the cellulase activity; moreover, Ca2+, as a divalent metal ion, could have more effect on the conformation deformation of the cellulase molecular structure, resulting in inhibition effect on its activity.22 In an another study, Yu et al. (2010) found that the common cations of ash (K+, Mg2+, Ca2+, Al3+, Mn2+, Fe3+, Cu2+, and Zn2+) all showed inhibitory effects on cellulase at different levels, except for the stimulative effects of Ca2+ and Mg2+ on β-glucosidase.23 The results in this study showed that only Ca-citrate had an inhibitory effect on the enzymatic hydrolysis of cellulosic materials. This suggested that the Na+ and Ca2+ was probably not the inhibitors for enzymatic hydrolysis of cellulosic materials by cellulase.
The inhibition of Ca-citrate was probably due to the unproductive adsorption of enzymes on the Ca-citrate solid particles. As can be seen, the Ca-citrate decreased the amount of free enzymes in solution by adsorption (Fig. 2). This result was in agreement with the study of interactions of enzymes and CaCO3.24 The enzymes' adsorption capacity of Ca-sulfate was apparently lower than for Ca-citrate, which was probably because of its slight solubility. The enzyme-binding mechanism for Ca-citrate has not been studied previously. A clue to its operative mechanism can be deduced from analogous mechanistic studies of enzyme–lignin interactions.25 This unproductive adsorption could be due to hydrophobic, hydrogen bond, and electrostatic interactions.24 The enzyme–Ca-citrate interaction mechanisms could be explained by electrostatic and hydrogen bond interactions because Ca-citrate is generally considered to present a hydrophilic surface.
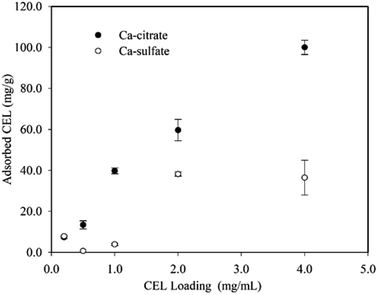 |
| Fig. 2 Adsorption of cellulase preparation (CEL) by Ca-citrate and Ca-sulfate. | |
Effect of salts on ABE fermentation
When the glucose medium contained 90 mM citric acid originally, the fermentation after neutralization by Ca(OH)2 produced 13.2 g L−1 ABE in 120 h, in which 8.3 g L−1 was butanol (Fig. 3A). The butanol and ABE yields were 0.21 and 0.33, respectively (Table 2). The concentrations of acetone, butanol and ethanol, and yields of these solvents were comparable to the control experiment without any salts. The solvents yields were also in agreement with previous studies.26–28 The increased concentration (120 mM) of citric acid did not affect the ABE fermentation. However, C. acetobutylicum DSM1731 did not grow when Na-citrate formed after neutralization by NaOH, which suggested that Na-citrate inhibited the fermentation. When Na-acetate and Na-sulfate formed, the butanol and ABE concentrations and yields after fermentation were apparently lower than control (Fig. 3B and C and Table 2).
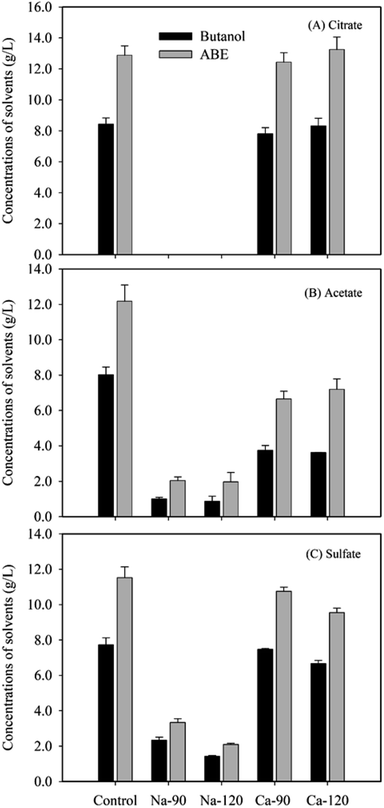 |
| Fig. 3 Concentrations of butanol and ABE after glucose fermentation by C. acetobutylicum DSM 1731 when (A) Na-citrate and Ca-citrate, (B) Na-acetate and Ca-acetate, and (C) Na-sulfate and Ca-sulfate were present in the medium. The 90 and 120 mM represents the concentration of citric, acetic, and sulfuric acid originally in the fermentation media. | |
Table 2 Concentrations of acetone, butanol and ethanol, and yields of butanol and ABE after glucose fermentation by C. acetobutylicum DSM 1731 when Na and Ca salts were present in the media. The 90 and 120 mM represents the concentration of citric, acetic, and sulfuric acid originally in the fermentation media
Salts |
Concentration (mM) |
Products (g L−1) |
Glucose (g L−1) |
Yields (g g−1) |
Acetone |
Butanol |
Ethanol |
ABE |
Original |
Residual |
Butanol |
ABE |
Control |
0 |
3.4 ± 0.2 |
8.4 ± 0.4 |
1.1 ± 0.1 |
12.8 ± 0.6 |
49.9 ± 0.4 |
7.7 ± 1.7 |
0.20 |
0.30 |
Ca-citrate |
90 |
3.7 ± 0.2 |
7.8 ± 0.4 |
1.0 ± 0.1 |
12.4 ± 0.6 |
43.9 ± 0.0 |
6.3 ± 1.7 |
0.21 |
0.33 |
120 |
3.9 ± 0.3 |
8.3 ± 0.5 |
1.0 ± 0.0 |
13.2 ± 0.8 |
45.6 ± 1.0 |
3.3 ± 3.0 |
0.20 |
0.31 |
Na-acetate |
90 |
0.5 ± 0.0 |
1.0 ± 0.1 |
0.2 ± 0.0 |
2.0 ± 0.2 |
49.0 ± 0.0 |
30.3 ± 2.2 |
0.05 |
0.11 |
120 |
0.8 ± 0.2 |
0.9 ± 0.3 |
0.3 ± 0.1 |
1.9 ± 0.5 |
48.0 ± 0.0 |
30.6 ± 3.9 |
0.05 |
0.11 |
Ca-acetate |
90 |
2.4 ± 0.1 |
3.7 ± 0.3 |
0.5 ± 0.0 |
6.7 ± 0.4 |
46.0 ± 0.0 |
19.1 ± 0.1 |
0.14 |
0.25 |
120 |
3.0 ± 0.6 |
3.6 ± 0.0 |
0.5 ± 0.0 |
7.2 ± 0.6 |
47.0 ± 0.0 |
18.6 ± 5.4 |
0.13 |
0.25 |
Na-sulfate |
90 |
0.7 ± 0.0 |
2.3 ± 0.2 |
0.3 ± 0.0 |
3.3 ± 0.2 |
50.7 ± 0.4 |
32.0 ± 0.9 |
0.12 |
0.18 |
120 |
0.5 ± 0.0 |
1.4 ± 0.0 |
0.2 ± 0.0 |
2.1 ± 0.1 |
49.1 ± 1.5 |
35.0 ± 0.6 |
0.10 |
0.15 |
Ca-sulfate |
90 |
2.5 ± 0.2 |
7.4 ± 0.1 |
0.8 ± 0.0 |
10.7 ± 0.2 |
47.4 ± 0.2 |
8.0 ± 0.9 |
0.19 |
0.27 |
120 |
2.2 ± 0.0 |
6.6 ± 0.2 |
0.7 ± 0.0 |
9.5 ± 0.2 |
42.2 ± 1.5 |
7.3 ± 1.4 |
0.19 |
0.27 |
The results suggested that the Na salts had a severe inhibitory effect on ABE fermentation by C. acetobutylicum DSM1731 and the order of the inhibitory effect was Na-citrate > Na-acetate > Na-sulfate. The inhibition of soluble Na salts was in accordance with the previous studies. Ezeji et al. (2007) reported that ABE concentrations decreased by 4.5 g L−1 and 6.5 g L−1 when Na-acetate (13.3 g L−1) and an additional Na-sulfate (8.9 g L−1) were present in the fermentation medium.14 Thus, a mixture of salts could be more toxic than a single salt. As reported previously, C. beijerinckii P260 produced less than 2.59 g L−1 ABE from alkaline peroxide-treated wheat straw hydrolysate without detoxification.29 After the removal of salts using electrodialysis, however, the ABE concentration increased to 22.17 g L−1.29 The presence of these inhibitors might affect microbial cell growth and inhibit the glycolytic and fermentative enzymes, which are essential to central metabolic pathways.30
When Ca-acetate and Ca-sulfate formed after neutralization by Ca(OH)2, increased concentrations and yields of solvents were obtained after fermentation compared to that with Na salts (Fig. 2B and C and Table 2). Similarly to Ca-citrate, Ca-sulfate produced comparable concentrations and yields of solvents to the control when the original sulfuric acid concentration was 90 mM (Fig. 3C and Table 2). The Ca-sulfate had slightly inhibitory effect when the original sulfuric acid concentration was 120 mM. However, Ca-acetate caused a decrease in the concentrations and yields (Fig. 3B and Table 2). The results suggested that Ca-acetate inhibited ABE fermentation. The different responses between the Ca salts were possibly due to their different solubility: i.e., Ca-citrate, Ca-sulfate and Ca-acetate salts were insoluble, slightly soluble, and soluble, respectively. Thus, most Ca-citrate and Ca-sulfate salts were precipitates on the bottom of the broth, which could not inhibit the ABE fermentation. Together with the results of enzymatic hydrolysis, we concluded that the completely insoluble salts formed during the neutralization process would inhibit the enzymatic hydrolysis of cellulosic materials; however, the soluble salts were inhibitors for the following ABE fermentation.
Process conditioning
The inhibitors for enzymatic hydrolysis of cellulosic materials and the following ABE fermentation can be broadly divided into two groups: (1) process inhibitors, which include different sugar and lignin degradation compounds, and the salts formed by acid–base reactions during pretreatment and neutralization processes; and (2) inherent inhibitors, which include monosaccharides and oligosaccharides, and butanol.14,30,31 Different detoxification methods, including lime, evaporation, peroxidase, adsorption using ion exchange resins, activated charcoal, and biological, have been used previously to reduce the process inhibitors associated with pretreatment and neutralization.19 However, process conditioning, e.g., selecting suitable pretreatment and neutralizing reagents and their proper concentrations, is a relatively simple method to avoid inhibition with salts in successful biofuel production from lignocellulosic materials.
A variety of acids have been investigated for pretreatment, such as sulfuric, oxalic, citric, acetic, and tartaric acids.17–19 The pretreated solids are usually separated from the liquid for the following hydrolysis using the buffer solutions. However, the separation and addition of buffer solution would increase the processing cost. Thus, direct neutralization for enzymatic hydrolysis and fermentation could improve this problem, and correspondingly, the inhibitory effect of formed salts must be considered. In this study, it seemed that the salts formed during the neutralization could be also used as the buffer solution for the enzymes, and some of the salts did not show inhibition on ABE fermentation. Sulfuric acid has been most widely used because it is inexpensive and effective. As shown in this study, the Ca-sulfate formed during sulfuric acid neutralization by using Ca(OH)2 as the neutralizing reagent did not inhibit the enzymatic hydrolysis of lignocellulosic materials, and also it had little inhibitory effect for ABE fermentation. Thus, the sulfuric acid as the pretreatment reagent and the Ca(OH)2 as the neutralizing reagent would be good option for process conditioning of biobutanol production from lignocellulosic materials. Certainly, it would be of interest to further investigate the neutralization process after pretreatment with other acids using different alkalis.
Conclusions
The Ca-citrate salt formed during neutralization had a severely inhibitory effect on cellulose hydrolysis, which was probably because of the adsorption interaction of cellulases on Ca-citrate solids. The soluble Na-citrate, -acetate and -sulfate inhibited ABE fermentation, but the insoluble Ca-citrate or slightly soluble Ca-sulfate did not. Interestingly, the Ca-sulfate did not show apparent inhibition on both hydrolysis and fermentation. The different inhibition capacities of the formed salts were probably due to their different solubilities. Thus, selecting suitable pretreatment and neutralization reagents is an alternative way to reduce inhibition with salts during the process of biofuel production from lignocellulosic materials.
Conflicts of interest
There are no conflicts to declare.
Acknowledgements
This work was supported by Start-up Fund for Introduced Talents of Hebei Agricultural University, China (YJ201910), the Fundamental Research Funds for the Central Universities of China (Z109021701). Financial support also came from the projects: “Sustainable production concepts in the integrated biorefining industry (SusBioRef)” funded by the Academy of Finland, “New value chains for biorefining (A71029)” funded by the European Regional Development Fund, and “Development of efficient biomass conversion routes for biofuel production and utilization (CONVER-B)” funded by INNO-INDIGO/Academy of Finland.
References
- H. G. Qiu, L. X. Sun, J. K. Huang and S. Eozelle, Renewable Sustainable Energy Rev., 2012, 16, 3095–3104 CrossRef CAS
. - C. W. Zhang, H. Wen, C. J. Chen, D. Cai, C. H. Fu, P. Li, P. Y. Qin and T. W. Tan, Renewable Energy, 2019, 134, 44–53 CrossRef CAS
. - D. T. Jones and D. R. Woods, Microbiol. Rev., 1986, 50(4), 484–524 CAS
. - S. B. Bankar, S. A. Survase, H. Ojamo and T. Granström, RSC Adv., 2013, 3, 24734–24757 RSC
. - S. Banerjee, S. Mudliar, R. Sen, B. Giri, D. Satpute, T. Chakrabarti and R. A. Pandey, Biofuels, Bioprod. Biorefin., 2010, 4, 77–93 CrossRef CAS
. - G. Jurgens, S. Survase, O. Berezina, E. Sklavounos, J. Linnekoski, A. Kurkijärvi, M. Väkevä, A. van Heiningen and T. Granström, Biotechnol. Lett., 2012, 34(8), 1415–1434 CrossRef CAS
. - C. W. Zhang, S. Y. Pang, M. Lv, X. Y. Wang, C. S. Su, W. X. Gao, H. D. Chen, D. Cai, P. Y. Qin and T. W. Tan, Energy Fuels, 2019, 33, 1210–1218 CrossRef CAS
. - H. Wen, H. D. Chen, D. Cai, P. W. Gong, T. Zhang, Z. C. Wu, H. T. Gao, Z. Z. Li, P. Y. Qin and T. W. Tan, Biotechnol. Biofuels, 2018, 11, 134 CrossRef
. - B. Yang, Z. Y. Dai, S. Y. Ding and C. E. Wyman, Biofuels, 2011, 2(4), 421–450 CrossRef CAS
. - M. Shafiei, R. Kumar and K. Karimi, in Lignocellulose-Based Bioproducts, ed. K. Karimi, Springer International Publishing, 2015, pp. 85–154 Search PubMed
. - C. E. Wyman, B. E. Dale, V. Balan, R. T. Elander, M. T. Holtzapple, R. S. Ramirez, M. R. Ladisch, N. S. Mosier, Y. Y. Lee, R. Gupta, S. R. Thomas, B. R. Hames, R. Warner and R. Kumar, in Aqueous Pretreatment of Plant Biomass for Biological and Chemical Conversion to Fuels and Chemicals, ed. C. E. Wyman, John Wiley & Sons, Ltd, 2013, pp. 239–259 Search PubMed
. - N. Mosier, C. Wyman, B. Dale, R. Elander, Y. Y. Lee, M. Holtzapple and M. Ladisch, Bioresour. Technol., 2005, 96(6), 673–686 CrossRef CAS
. - F. Hu and A. Ragauskas, BioEnergy Res., 2012, 5, 1043–1066 CrossRef CAS
. - T. Ezeji, N. Qureshi and H. P. Blaschek, Biotechnol. Bioeng., 2007, 97, 1460–1469 CrossRef CAS
. - N. Qureshi, T. C. Ezeji, J. Ebener, B. S. Dien, M. A. Cotta and H. P. Blaschek, Bioresour. Technol., 2008a, 99, 5915–5922 Search PubMed
. - I. S. Maddox, E. Steiner, S. Hirsch, S. Wessner, N. A. Gutierrez, J. R. Gapes and K. C. Schuster, J. Mol. Microbiol. Biotechnol., 2000, 2, 95–100 CAS
. - L. Qin, Z. H. Liu, N. Z. Li, B. E. Dale and Y. J. Yuan, Bioresour. Technol., 2012, 112, 319–326 CrossRef CAS
. - O. M. Kwon, D. H. Kim, S. K. Kim and G. T. Jeong, Algal Res., 2016, 13, 293–297 CrossRef
. - T. A. L. Silva, H. D. Z. Zamora, L. H. R. Varão, N. S. Prado, M. A. Baffi and D. Pasquini, Waste Biomass Valorization, 2018, 9, 2191 CrossRef CAS
. - A. Hirsch and E. Grinsted, J. Dairy Res., 1954, 21, 101–110 CrossRef
. - J. Zhu, L. Shi, L. Zhang, Y. Xu, Q. Yong, J. Ouyang and S. Yu, Bioprocess Biosyst. Eng., 2016, 39(10), 1619–1626 CrossRef CAS
. - H. Zhang, J. Zhao, Z. Lin and H. Huang, Chin. J. Bioprocess Eng., 2011, 9(1), 1–4 CrossRef CAS
. - B. Yu and H. Chen, Bioresour. Technol., 2010, 101, 9114–9119 CrossRef
. - B. C. Min and B. V. Ramarao, Bioprocess Biosyst. Eng., 2017, 40(6), 799–806 CrossRef CAS
. - H. Liu, J. Sun, S. Y. Leu and S. Chen, Biofuels, Bioprod. Biorefin., 2016, 10(5), 648–663 CrossRef CAS
. - S. Y. Li, R. Srivastava, S. L. Suib, Y. Li and R. S. Parnas, Bioresour. Technol., 2011, 102(5), 4241–4250 CrossRef CAS
. - M. Yang, S. Kuittinen, J. Vepsäläinen, J. Zhang and A. Pappinen, Bioresour. Technol., 2017, 243, 126–134 CrossRef CAS
. - C. W. Zhang, H. D. Chen, N. An, C. S. Su, M. Lv, S. Y. Pang, X. Y. Wang, D. Cai and P. Y. Qin, Ind. Crops Prod., 2019, 139, 111500 CrossRef CAS
. - N. Qureshi, B. C. Saha, R. E. Hector and M. A. Cotta, Biomass Bioenergy, 2008b, 32, 1353–1358 Search PubMed
. - N. R. Baral and A. Shah, Appl. Microbiol. Biotechnol., 2014, 98, 9151–9172 CrossRef CAS
. - H. B. Klinke, A. B. Thomsen and B. K. Ahring, Appl. Microbiol. Biotechnol., 2004, 66, 10–26 CrossRef CAS
.
Footnote |
† Equally contributed by both authors in this article. |
|
This journal is © The Royal Society of Chemistry 2019 |