DOI:
10.1039/C9RA06353F
(Paper)
RSC Adv., 2019,
9, 30671-30684
Impact of La3+ and Y3+ ion substitutions on structural, magnetic and microwave properties of Ni0.3Cu0.3Zn0.4Fe2O4 nanospinel ferrites synthesized via sonochemical route
Received
14th August 2019
, Accepted 19th September 2019
First published on 26th September 2019
Abstract
In the current study, Ni0.4Cu0.2Zn0.4LaxYxFe2−xO4 (x = 0.00 − 0.10) nanospinel ferrites (NSFs) were fabricated via an ultrasonic irradiation route. The creation of single phase of spinel nanoferrites (NSFs) was investigated by X-ray powder diffractometry (XRD) and selected area diffraction pattern (SAED). The cubic morphology of all samples was confirmed by scanning and transmission electron microscopies (SEM and TEM) respectively. The UV-Vis investigations provided the direct optical energy band gap values in a narrow photon energy interval of 1.87–1.92 eV. The 57Fe Mössbauer spectroscopy analysis explained that the hyperfine magnetic fields of Octahedral (Oh) and Tetrahedral (Td) sites decreased with substitution. The paramagnetic properties of NPs decrease with increase of content of doped ions. Investigations of magnetic properties reveal a superparamagnetic nature at 300 K and soft ferromagnetic trait at 10 K. The Ms (saturation magnetization) and Mr (remanence) decrease and the Hc (coercivity) increases slightly with La3+ and Y3+ substitution. The observed magnetic traits are deeply discussed in relation with the morphology, structure, magnetic moments and cation distributions. The microwave characterization of the prepared NSFs showed that, dissipation (i.e., absorption) of incoming microwave energy occurs at a single frequency, for each sample, lying between 7 and 10.5 GHz. The reflection losses (RL) at these frequencies range from −30 to −40 dB and the mechanism of which is explained in the framework of dipolar relaxation and spin rotation. The best microwave properties were obtained with a LaY concentration of x = 0.08 having an RL of −40 dB @ 10.5 GHz and an absorption bandwidth of 8.4 GHz @ −10 dB. With these high values of RL and absorbing bandwidth, LaY doped NiCuZn NSF products would be promising candidates for radar absorbing materials in the X-band.
1. Introduction
Nanocrystalline spinel ferrites (NSFs) are promising materials in the few last decades because of their unique electrical and magnetic features including high resistivity, high Curie temperature, high saturation magnetization and low eddy current loss. Thus, NSFs have found a considerable amount of use in environmental, biomedical, industrial and technological applications including magnetic resonance imaging microwave absorbers, contrast agents, catalysts, drug delivery agents, magnetic storage devices etc.1–9 Ni–Cu–Zn ferrite is a spinel ferrite mainly used in multi-layer chip inductors (MLCIs).10 MLCIs are mainly used in mobile phones, notebook computers and video cameras. MLCIs are produced with alternating layers of silver electrodes and spinel ferrites. For further miniaturization of these devices, it is important to obtain fewer layers with high efficiency. Therefore, NiCuZn ferrites can be tuned with diverse substitution contents and/or various metal ions to produce a product with high resistivity, low coercivity, high permeability and low dielectric constant.11 In Ni–Cu–Zn spinel lattice, Cu2+ and Ni2+ ions prefer to occupy the Oh sites, Zn2+ ions occupied the Td sites, and Fe3+ ions occupied both Oh (A) and Td (B) sites partially. The A–A, B–B and A–B superexchange interactions are responsible for magnetization in spinel ferrites.12 Consequently, the saturation magnetization of a spinel ferrite can be tuned by the various metal ion substitution.
Rare earth elements present a novel approach for obtaining NSFs desired characteristics for MLCI applications as well as other uses. Rare earth metal ions whose ionic radii are greater than that of the iron(III) ion, micro-strains will be established in the spinel ferrite crystal structure. Micro-strains will trigger a domain wall motion which will result with a deformation in the lattice cell. The domain wall motion and the spin rotational magnetization within the domains are responsible for the permeability. In fact, lower amounts of dopants may stabilize the microstructure while higher concentrations which are above the solubility limit may cause a non-uniform grain size.13 Therefore, the rare earth substitution of NSFs can result in enhanced permeability when the substituting ratio is small. In addition, rare earth metal ions are known to enhance the optical properties of ferrites. For example, the incorporation lanthanum(III) to ZnFe2O4 nanoparticles increased the optical band gap (Eg) from 1.87 eV to 1.97 eV.14 Magnetite nanoparticles doped with Dy, Nd, or La were found the display direct gap values of 3.2–3.55 eV and indirect band gap values of 1.1–1.25 eV when compared to a direct Eg of 2.25 eV and indirect Eg of 0.9 eV in pure magnetite samples.15
Many different synthesis methods have been sued for Ni–Cu–Zn spinel ferrites synthesis. For example, Hu and Yan16 applied a ball-milling technique to synthesize (Ni0.17Zn0.63Cu0.20)Fe1.915O4, a Ni–Zn ferrite with copper (CuO) and vanadium (V2O5) addition. The sintering temperature decreased and the granularity of the particles was reduced by the addition of Cu2+ and V5+ to the ferrite while an enhanced permeability was obtained since the domain wall motion was improved by higher density and bigger grain. Kabbur et al.17 used a glycine-assisted sol–gel combustion for Ni0.25−xMgxCu0.30Zn0.45Fe2O4 synthesis. Although the magnetic moments and the saturation magnetization values had fluctuations as the amount of magnesium ion increased, the substitution of nickel and magnesium resulted in an increase in the electrical resistivity and the sample where the Mg2+ content was x = 0.15 displayed the minimum dielectric loss tangent. In another study by Krishnaveni and co-workers,18 Ni0.53Cu0.12Zn0.35Fe2O4 nanopowders were obtained by microwave-hydrothermal synthesis technique and MLCIs were fabricated with nanoparticles annealed between 600–950 °C temperatures. The sample sintered at 900 °C for 4 h displayed the highest resistance, initial permeability, and inductance. Al-substituted Ni–Cu–Zn ferrites were fabricated by ceramic method in a study done by Eltabey et al.19 As the aluminum ion concentration increased, the initial permeability, magnetization, and the dc resistivity were also found to increase. Finally, Ni0.4Cu0.2Zn0.4Fe2O4 nanoparticles were obtained by a sonochemically-assisted co-precipitation.20 When a high-powered ultrasonic wave is applied to irradiate a liquid, several radially oscillating bubbles with micron sizes are formed.21 This is called as acoustic cavitation. The ultrasonic wave's pressure oscillation causes the bubbles keep expanding and contracting violently. Among various methods, ultrasound irradiation assisted synthesis has many advantages such as low to no agglomeration of particles, the need for less induction time, higher number of crystals at equal supersaturation and the ability to control the particle size.20
In the literature, there are a limited number of reports available on the synthesis of rare-earth substituted Ni–Cu–Zn nanospinel ferrites via sonochemical. No reports have been published on the preparation of Ni–Cu–Zn nanospinel ferrites substituted with lanthanum and yttrium ions simultaneously. Therefore, we reported the synthesis of Ni0.3Cu0.3Zn0.4LaxYxFe2−2xO4 (x = 0.0 − 0.10) NSFs via ultrasonic irradiation. The structure, morphology, magnetic and microwave characteristics were investigated.
2. Experimental details
The Ni0.3Cu0.3Zn0.4LaxYxFe2−2xO4 (x = 0.0 − 0.10) NSFs were synthesized using ultrasonic irradiation procedure. The following grade precursors (sigma Aldrich) of Ni(NO3)2·6H2O, Cu(NO3)2·3H2O, Fe(NO3)3·9H2O, Zn(NO3)2·6H2O, La(NO3)3·6H2O, C6H8O7 and Y2O3 are used as initial materials. Firstly, Y2O3 was solved in 15 ml of concentrated HCl at 150 °C until became a clear solution. The nitrites with citric acid are dissolved in 60 ml of DI water then mixed with dissolved Y2O3 under stirring. A dropwise of 2 M NaOH solution has been used to set the pH at 11. The solution was undergone to ultrasonic irradiation via UZ SONOPULS HD2070 homogenizer (20 kHz and 70 W) for 1 h. Then, the solution containing solid product was washed with DI water. The solid part was separated by means of external magnet and dried at 80 °C.
The phase analysis has been done through Rigaku Benchtop Miniflex X-ray diffraction (XRD) with CuKα radiation. The morphology, size and chemical compositions were performed using FEI Teneo scanning electron microscope (SEM) equipped with an EDX detector and a transmission electron microscopy (TEM; FEI Morgagni 268). UV-visible diffuse reflectance (DR%) spectra were recorded in the range of 200–800 nm. MagLab instrument was used for Mössbauer analysis of samples. The quantum design coupled with VSM was utilized for magnetic characterization of samples.
3. Results and discussion
3.1. Structural analysis
The phase identification of the mixed Ni0.4Cu0.2Zn0.4LaxYxFe2−xO4 (x = 0.00 − 0.10) NSFs was performed in Fig. 1. NiCuZn spinel ferrite phase has been confirmed without presence of any impurities. The diffraction lines (220), (311), (222), (400), (422), (511) and (440) well accord with the single phase of spinel ferrite. Rietveld refinement of XRD experimental data was employed through full proof software to estimate the lattice parameters (Table 1). The average crystallites size was calculated through Debye–Scherrer equation (Table 1). The lattice parameter ‘a’ increased with increasing the La–Y content. This is attributable to the distortion of the crystal structure caused by the occupation of octahedral sites by large ionic radii of La3+ and Y3+ substitution ions. The average crystallites size slightly decreases from about 23 to 12 nm with the increase of La–Y concentration.
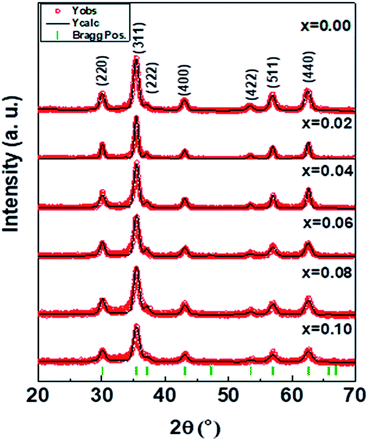 |
| Fig. 1 XRD powder patterns of Ni0.4Cu0.2Zn0.4LaxYxFe2−xO4 (x = 0.00 − 0.10) NSFs. | |
Table 1 Refined structural parameters for Ni0.4Cu0.2Zn0.4LaxYxFe2−xO4 (x = 0.00 − 0.10) NSFs
x
|
a (Å) |
V (Å)3 |
D
XRD (nm) ± 0.04 |
χ
2 (chi2) |
R
Bragg
|
0.00 |
8.397(4) |
592.14 |
22.8 |
1.36 |
2.73 |
0.02 |
8.398(5) |
592.39 |
21.6 |
1.78 |
1.90 |
0.04 |
8.406(7) |
594.12 |
20.6 |
1.56 |
5.96 |
0.06 |
8.408(6) |
594.52 |
17.3 |
1.47 |
5.36 |
0.08 |
8.410(4) |
594.90 |
16.8 |
1.24 |
5.17 |
0.10 |
8.416(0) |
596.09 |
12.4 |
1.25 |
2.41 |
Bertaut method has been employed to estimate the cations distribution of all the prepared samples by analyzing XRD patterns.22,23 As it was given in the literature, M+3 and M+2 cation distributions among the Td and Oh sites were estimated from the intensity ratio of I220/I440 and I422/I400 planes. Therefore, these planes were used in the cation distribution calculations.23 The estimated cations distribution is listed in Table 2. It has been noticed that Fe3+ ions occupy both Td and Oh sites. Rare-earth La3+ ions occupied Oh (B)-site since they have higher ionic radii whereas Y3+ ions occupied Td (A)-site. Zn2+ occupied the Td (A-site) and on the other hand Cu2+ and Ni2+ ions occupied the Oh (B)-site. This is consistent with the literature reports.24,25
Table 2 Cation distribution of Ni0.4Cu0.2Zn0.4LaxYxFe2−xO4 (x = 0.00 − 0.10) NSFs
x
|
Tetrahedral A-site |
Octahedral B-site |
0.00 |
Zn0.4Ni0.05Fe0.55 |
Ni0.35Cu0.2Fe1.45 |
0.02 |
Zn0.4Y0.02Fe0.58 |
Ni0.4Cu0.2La0.02Fe1.38 |
0.04 |
Zn0.4Y0.04Fe0.56 |
Ni0.4Cu0.2La0.04Fe1.36 |
0.06 |
Zn0.4Y0.06Fe0.54 |
Ni0.4Cu0.2La0.06Fe1.34 |
0.08 |
Zn0.4Y0.08Fe0.52 |
Ni0.4Cu0.2La0.08Fe1.32 |
0.10 |
Zn0.4Y0.1Fe0.50 |
Ni0.4Cu0.2La0.1Fe1.30 |
3.2. Morphological investigations
The surface morphology of Ni0.4Cu0.2Zn0.4LaxYxFe2−xO4 (x = 0.00 − 0.10) NSFs is offered in Fig. 2. High magnification SEM images revealed smaller grains with size in the range of few tens nanometer.26 These grains are agglomerated due to the magnetic nature of prepared samples. EDX and elemental mapping results (Fig. 3) did not disclose any preferential segregation of heavy elements, indicating a homogeneous chemical composition. They confirmed the presence of different elements of Ni, Cu, Zn, La, Y, Fe and O and proved the formation of the desired compositions. TEM images, selected area electron diffraction (SAED) pattern and size histograms of the x = 0.02 and 0.06 compositions are presented in Fig. 4.
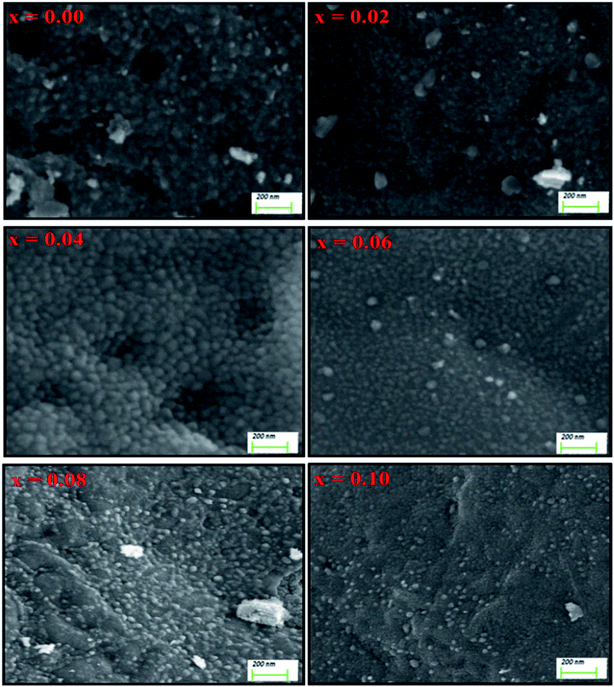 |
| Fig. 2 SEM images of various Ni0.4Cu0.2Zn0.4LaxYxFe2−xO4 (x = 0.00 − 0.10) NSFs. | |
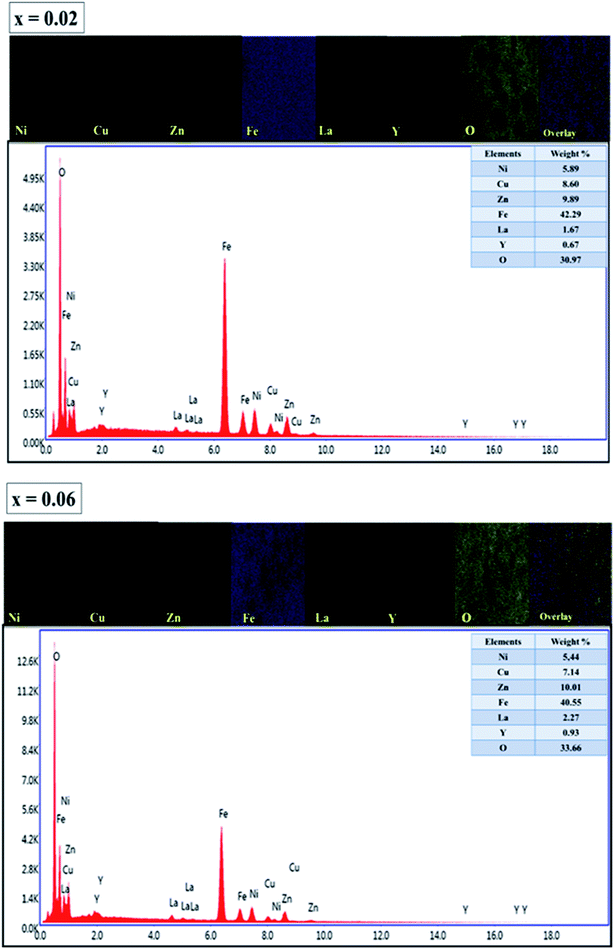 |
| Fig. 3 EDX spectra and elemental mapping of Ni0.4Cu0.2Zn0.4LaxYxFe2−xO4 (x = 0.00 − 0.10) NSFs for x = 0.02 and 0.06 compositions. | |
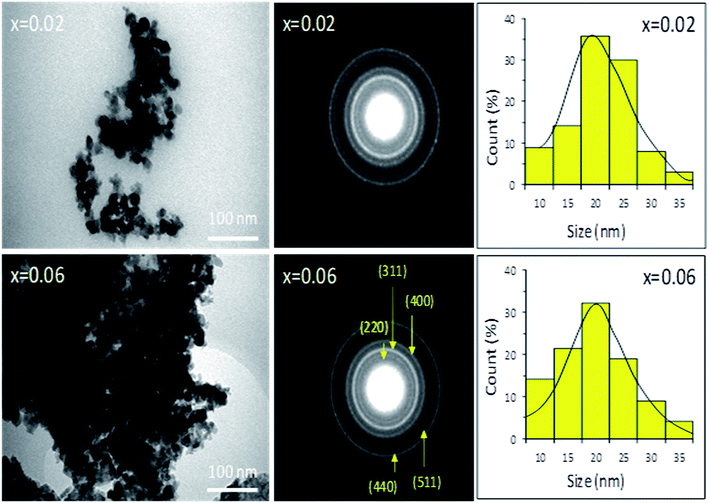 |
| Fig. 4 TEM images, SAED patterns and size histograms of Ni0.4Cu0.2Zn0.4LaxYxFe2−xO4 (x = 0.00 − 0.10) NSFs for x = 0.02 and 0.06 compositions. | |
They indicated a size distribution ranging between 10 and 35 nm, and the average grains size is around 20 nm. The estimated sizes agreed very-well with the crystallites size deduced from XRD. SAED patterns revealed the well crystalline nature of the particles as confirmed by the well-separated continuous rings. The first five rings of the SAED patterns were identified as (220), (311), (400), (511) and (440). The maximum intensity was obtained for (311) reflection which is consistent with the XRD pattern.27
3.3. Optical properties
Optoelectronic properties of Ni0.4Cu0.2Zn0.4LaxYxFe2−xO4 (x = 0.00 − 0.10) NSFs were investigated via percent diffuse reflectance (DR%) measurements in the UV-Vis region of electromagnetic spectrum, Fig. 5. The spectrophotometer swept a photon energy range from 1.55 eV to 6.2 eV with a corresponding wavelength interval from 800 nm to 200 nm. Recorded graphs from all NiCuZn mixed ferrite samples reflect the incident radiation with the magnitudes between 26–31% in a sweep region of 200–500 nm. In the same sweep region, non-ion substituted Ni0.4Cu0.2Zn0.4Fe2O4 nanoparticle sample has reflectance average of 30%. A sharp increase is detected at DR% values at all spectra until maximum 71% in the following sweep range. Scientific facts to use DR% spectra to specify the optical energy band gap of powder samples is correlated with the Kubelka–Munk theory. In the theory, Kubelka–Munk function (KM) is directly proportional to absorption coefficient, α
here, R∞ is absolute remittance, ε is absorptivity, S is twice the scattering coefficient, C is analyte concentration. Optical energy band gap (Eg) values are estimated applying Tauc and Davis-Mott model that provides another equation between α and Eg as below:28–30
where h is Planck's constant, ν is frequency of incident radiation and A is a proportionality constant. Further, power n specifies that electronic transitions are indirect or direct and also in-allowed or allowed. Magnitude of n equals to 1/2 for a direct and allowed transition. Direct Eg values for all Ni0.4Cu0.2Zn0.4LaxYxFe2−xO4 (x = 0.00 − 0.10) were determined by extrapolating the strait line segment of Tauc plots to (αhν)2 = 0 and were given in Fig. 6. Non-ion substituted mixed spinel Ni0.4Cu0.2Zn0.4Fe2O4 has 1.87 eV band gap. Substitution of La3+ and Y3+ ions with equal concentrations of x = 0.02 − 0.10 caused slight increments from this magnitude. All ion-substituted NPs have direct Eg magnitudes between minimum 1.88 eV (x = 0.10) and maximum 1.92 eV (x = 0.04). In the literature, the single reported Eg data from Tm substituted NiCuZn NSFs produced by ultrasound irradiations belongs to our group. Eg magnitudes are around 1.90 eV again.31 However, George et al. also reports very close values just below 2 eV for Ce3+ substituted Ni–Zn mixed ferrite.32
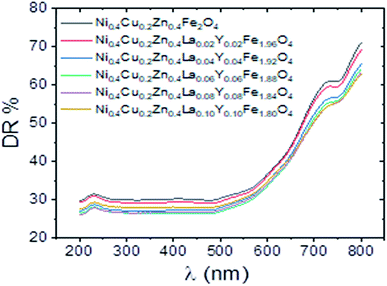 |
| Fig. 5 DR% vs. λ spectra of Ni0.4Cu0.2Zn0.4LaxYxFe2−xO4 (x = 0.00 – 0.10) NPs in 200–800 nm. | |
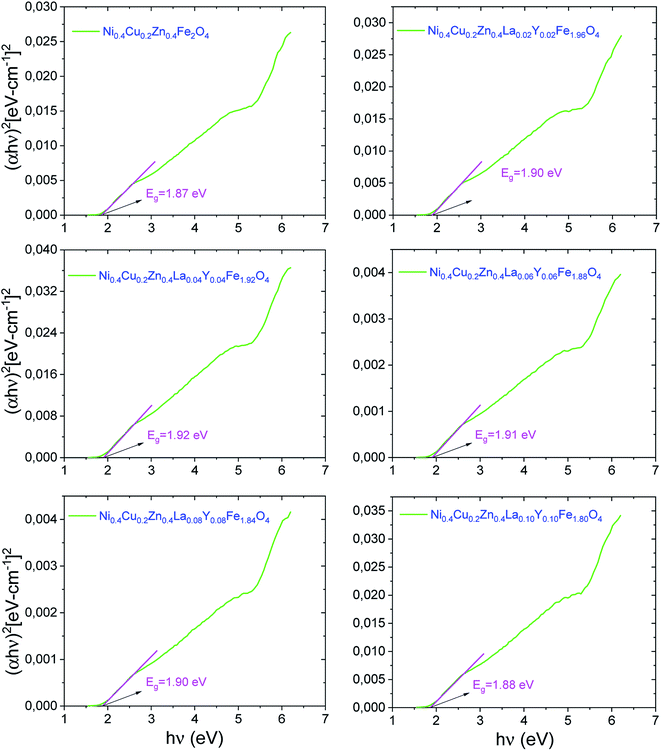 |
| Fig. 6 Tauc plots and extrapolated direct Eg data of Ni0.4Cu0.2Zn0.4LaxYxFe2−xO4 (x = 0.00 − 0.10) NPs. | |
3.4. Mössbauer spectra
Room-temperature Mössbauer spectra of Ni0.4Cu0.2Zn0.4LaxYxFe2−xO4 (x = 0.00 − 0.10) NSFs are depicted in Fig. 7 and the fitted parameters are presented in Table 3. The fitting was performed by means of three sextets, A for Td sites and B1 and B2 for Oh sites for undoped sample. For the doped samples, the paramagnetic doublet derived from superparamagnetic Fe3+ species is additionally formed. The area of this doublet increased from 9.102% to 50.126% as La3+ and Y3+ concentration increases from 0.02 to 0.10. The Fe3+ ions in A-site are characterized by small isomer shift (I.S.) and large hyperfine field (Hf), whereas Fe3+ ions in B-site are represented by smaller Hf.33 The lower covalent behavior of Fe3+–O2− bonds is the reason of greater Hf in A-sites than that in B-sites.34 The values of I.S. are in the 0.277–0.482 mm s−1 characteristics of high spin Fe3+ charge state.35
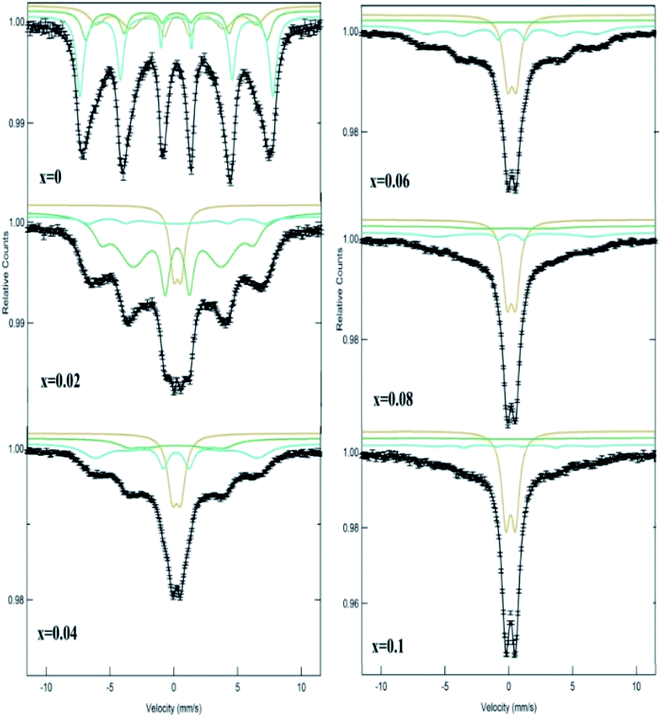 |
| Fig. 7 RT Mössbauer spectra of Ni0.4Cu0.2Zn0.4LaxYxFe2−xO4 (x = 0.00 − 0.10) NSFs. | |
Table 3 Parameters deduced from Mössbauer spectra for Ni0.4Cu0.2Zn0.4LaxYxFe2−xO4 (x = 0.00 − 0.10) NSFsa
x
|
Spectral component |
I.S. (±0.01) (mm s−1) |
Q.S. (±0.02) (mm s−1) |
H
hf (±0.1) (T) |
Area (%) |
(Hhf: hyperfine magnetic field. I.S.: isomer shift. Q.S.: quadrupole splitting. W: line width. RA: relative area).
|
0.00 |
Sx-A: Fe3+ |
0.277 |
0.013 |
45.212 |
19.489 |
Sx-B1: Fe3+ |
0.291 |
−0.09 |
42.486 |
25.434 |
Sx-B2: Fe3+ |
0.318 |
−0.027 |
37.616 |
55.077 |
0.02 |
Sx-A: Fe3+ |
0.293 |
−0.002 |
43.046 |
37.15 |
Sx-B: Fe3+ |
0.344 |
0.024 |
37.071 |
53.748 |
Db: Fe3+ |
0.341 |
0.587 |
— |
9.102 |
0.04 |
Sx-A: Fe3+ |
0.305 |
0.012 |
39.413 |
31.88 |
Sx-B1: Fe3+ |
0.36 |
−0.051 |
23.029 |
42.643 |
Db: Fe3+ |
0.321 |
0.669 |
— |
25.476 |
0.06 |
Sx-A: Fe3+ |
0.294 |
−0.024 |
40.258 |
28.743 |
Sx-B: Fe3+ |
0.312 |
0.072 |
17.227 |
33.107 |
Db: Fe3+ |
0.327 |
0.661 |
— |
38.149 |
0.08 |
Sx-A: Fe3+ |
0.441 |
0.246 |
37.403 |
15.79 |
Sx-B: Fe3+ |
0.478 |
−0.062 |
19.994 |
34.129 |
Db: Fe3+ |
0.326 |
0.673 |
— |
50.081 |
0.10 |
Sx-A: Fe3+ |
0.389 |
0.202 |
37.264 |
7.377 |
Sx-B: Fe3+ |
0.482 |
0.092 |
15.442 |
42.497 |
Db: Fe3+ |
0.327 |
0.738 |
— |
50.126 |
The isomer shift of A and B site continuously increased with increasing La3+ and Y3+ amount. This indicates that the s electron density on both A and B sites decreases with substitution. The quadrupole splitting (Q.S.) occurs because of chemical disorder in the system resulting in an electric field gradient.35 The Q.S. values of A and B sites with respect to substitution are negligible for up to x = 0.06. Q.S. is being highest in A site for x = 0.08 and 0.10 values. This illustrates a reduction of the cubic symmetry around Fe3+ ions with doping. As shown in Table 3, the Hf of iron nuclei at A and B sites decreases with increasing substitutions. This is ascribed to the substitution of magnetic Fe3+ (with magnetic moment of 5 μB) ions by nonmagnetic La3+ and Y3+ ions. This provokes a reduction in the superexchange interaction among different ions.
3.5. Magnetic properties
Magnetic traits of Ni0.4Cu0.2Zn0.4LaxYxFe2−xO4 (x = 0.00 − 0.10) NSFs were evaluated using VSM. The experiments of magnetization against applied magnetic field (M–H) of Ni0.4Cu0.2Zn0.4LaxYxFe2−xO4 (x = 0.00 − 0.10) NSFs were performed at 300 K and 10 K over the field range of ±70 kOe. The results of magnetic hysteresis loops of all products are presented in Fig. 8. From this figure, the magnitudes of Ms, Hc and Mr at both 300 K and 10 K were deduced and listed in Table 4. It is noticed that the various Ni0.4Cu0.2Zn0.4LaxYxFe2−xO4 (x = 0.00 − 0.10) NSFs show superparamagnetic (SPM) behavior at RT (Fig. 8(a) and (b)). However, the magnetization curve at 10 K (Fig. 8(c) and (d)) illustrates typical character of soft ferromagnetic (FM) nanomaterials characterized by closed hysteresis loops with Ms, Mr and Hc. It is noticed that the Ms of all produced nanoparticles are greater at low temperature than that of RT. For pure Ni0.4Cu0.2Zn0.4Fe2O4 (x = 0.00) ferrite, Ms magnitude increases from 55.5 emu g−1 at 300 K to 88.3 emu g−1 at 10 K. The increase in Ms at 10 K compared with RT is ascribed to reduction of the thermal fluctuations and surface spin disorders at the surfaces of the nanoparticles.36–38 Indeed, the surface spins at high temperatures could be subjected in a short time to many disorder states with similar energies that lead to weaken their responses to the applied field and consequently decreases the magnetization in comparison to their bulk counterpart. Nevertheless, the surface spins can freeze to a certain state below the freezing/blocking temperature, which rises the projection of the moments in the direction of H. Moreover, the coercivity values of all the products increased at 10 K when compared with 300 K. The enhancement in Hc is ascribed to reduced thermal fluctuations energy at low temperatures that is less efficient in decreasing the effects of magneto-crystalline anisotropy energy.
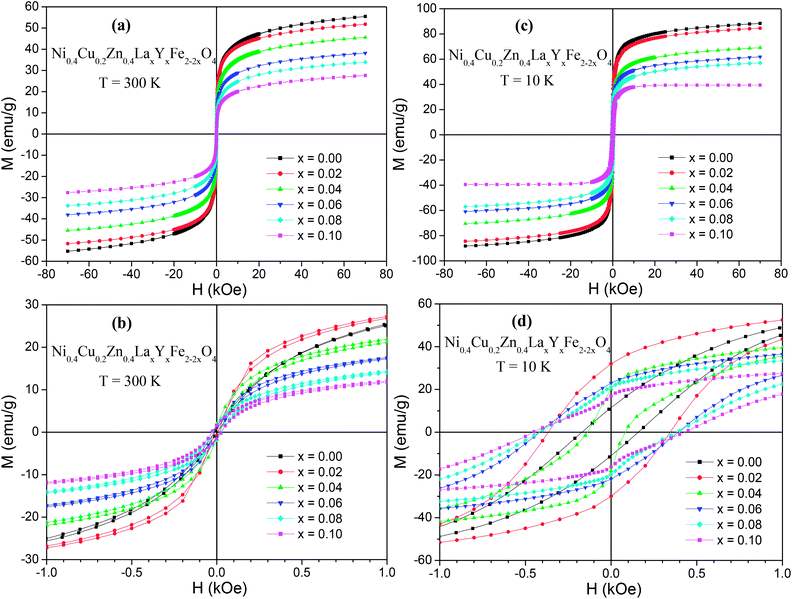 |
| Fig. 8
M–H hysteresis loops of Ni0.4Cu0.2Zn0.4LaxYxFe2−xO4 (x = 0.00 − 0.10) NSFs performed at (a and b) T = 300 and (c and d) T = 10 K. | |
Table 4 Magnetic parameters of Ni0.4Cu0.2Zn0.4LaxYxFe2−xO4 (x = 0.00 − 0.10) NSFs deduced from VSM measurements performed at 300 and 10 K
x
|
M
s (emu g−1) |
M
r (emu g−1) |
R = Mr/Ms |
H
c (Oe) |
n
B (μB) |
300 K |
10 K |
300 K |
10 K |
300 K |
10 K |
300 K |
10 K |
300 K |
10 K |
0.00 |
55.5 |
88.3 |
0.81 |
11.9 |
0.014 |
0.134 |
12.9 |
173.2 |
2.37 |
3.76 |
0.02 |
51.9 |
84.7 |
1.04 |
32.3 |
0.020 |
0.381 |
9.5 |
364.4 |
2.23 |
3.65 |
0.04 |
45.7 |
69.2 |
1.92 |
20.2 |
0.042 |
0.292 |
15.5 |
138.8 |
1.99 |
3.01 |
0.06 |
38.2 |
61.9 |
1.35 |
22.8 |
0.035 |
0.368 |
18.2 |
415.1 |
1.68 |
2.72 |
0.08 |
33.9 |
57.0 |
1.80 |
21.4 |
0.053 |
0.375 |
28.2 |
424.6 |
1.50 |
2.52 |
0.10 |
27.7 |
39.5 |
1.60 |
17.1 |
0.058 |
0.433 |
29.2 |
452.1 |
1.24 |
1.77 |
The pure NiCuZn ferrite displays the highest Ms magnitudes of 55.5 and 88.3 emu g−1 at 300 K and 10 K, respectively. These magnitudes agreed very-well with those reported in NiCuZn nanoferrites prepared via sol–gel auto-combustion process39,40 and in NiCuZn thin films.41 We have observed that there is no improvement in the Ms magnitudes with substitutions. Ms values decrease when the crystallites size reduces with the increase of La3+ and Y3+ substitution levels.42,43 Usually, it is ascribed to the surface effects of the magnetic NPs owing to smaller crystallites size, which could be explained by assuming the occurrence of dead magnetic layers because of surface spins disorders.44 It is anticipated that the number of spins at the surface of different prepared ferrites increases as the crystallites size become smaller. Moreover, the decrease in Ms of NiCuZn ferrites with La3+ and Y3+ substitutions is considered with the zero magnetic moments of La3+ and Y3+ ions and their preferential distributions in the octahedral lattice. It is found that the magnetic properties follow the theoretical decrease tendency owing to the replacement of Fe3+ ions displaying magnetic moments of 5 μB with La3+ and Y3+ ions having zero magnetic moments. Furthermore, Ms could decrease as a result of reduced exchange interactions among Fe3+–O–Fe3+, which are substituted with the weaker Fe3+–O–La3+ and Fe3+–O–Y3+ interactions. Consistent with Néel's sublattice model, spinal ferrites exhibit three kinds of interactions among tetrahedral (A) and octahedral (B) sites, e.g. A–A, B–B and A–B.45,46 A–B sublattice interaction is the strongest among these three interactions.44 The total magnetization of doped NiCuZn ferrites is the difference amongst the magnetization of B and A sites. Because of their larger ionic radii, rare earth ions generally occupy the B sites.47 Hence, the replacement of La3+ and Y3+ ions having zero magnetic moments at the B sites in NiCuZn ferrite lattices reduces the magnetization. The experimental magnetic moments nB per unit formula in units of Bohr magneton were determined via the following expression:48,49
The deduced nB values were listed in the Table 4. A good agreement between nB and Ms variation tendency was observed.
The pure NiCuZn ferrite has the highest Ms and nB values at both RT and 10 K, and then they decrease with increasing the La3+ and Y3+ substitution contents. The decrease in nB is owing to the circumstance that the magnetization of the B sites is weakening and, hence, the A–B super-exchange interaction is weakening with La3+ and Y3+ substitutions, which would then disrupt the structure of collinear spins in the NiCuZn ferrite systems at the B sites.44 These results are in accordance with Mössbauer results that show a reduction in the super-exchange interaction.
On the other hand, it is noticed from Table 4 that the coercivity of Ni0.4Cu0.2Zn0.4LaxYxFe2−xO4 (x = 0.00 − 0.10) NSFs increases with rising x. Pure NiCuZn NSFs exhibit Hc values of 12.9 (at T = 300 K) and 173.2 Oe (at T = 10 K), which increase to the 29.2 (at T = 300 K) and 452.1 Oe (at T = 10 K) for Ni0.4Cu0.2Zn0.4La0.1Y0.1Fe1.8O4 (i.e. x = 0.1). It is well-known that the Hc and Ms are related by the following expression:47
where
μ0 is the permeability of free space and
K is the magneto-crystalline anisotropy constant. Since
Ms was found to decrease with
x, the observed increase in coercivity could be a result of the strengthening of the magneto-crystalline anisotropy with La
3+ and Y
3+ substitutions.
50,51 Moreover, it is known that
Hc and grains size are inversely proportional.
52 Indeed, larger grains offer lesser pinning of domain walls because of the lower volume fractions of grain boundaries.
44 In the present study, it is observed that the crystallites size reduced with La
3+ and Y
3+ substitutions. Therefore, the slow improvement of
Hc with La
3+ and Y
3+ substitutions could be ascribed to the decrease in grains size. The values of squareness ratio “
R” at both measurement temperatures are calculated and listed in
Table 3. Commonly, the nanoparticles are in multi-magnetic domain (MMD) when
R ≥ 0.5 and in single magnetic domain (SMD) when
R < 0.5.
53,54 The achieved
R values reflect the MD nature at both 300 and 10 K in the prepared spinel ferrites nanoparticles.
3.6. Microwave properties
Microwave properties of Ni0.4Cu0.2Zn0.4LaxYxFe2−xO4 (x = 0.00 − 0.10) NSFs were determined by obtaining reflection losses (RL) spectra of the synthesized NPs between 2 and 18 GHz using coaxial airline. Having measured the S-parameters in vector network analyzer, complex permittivity (εr) and permeability (μr) values were calculated using material characterization software in this frequency range. Then, normalized input impedence of our NiCuZn NPs were deduced using the complex permittivity and permeability values via following eqn.
here d is the sample thickness, f is the frequency and c is the speed of light. Finally, the reflection loss spectra of each sample were derived using above equation which was determined via the transmission line theory for a single layer and metal-backed absorber.
Fig. 9 represents the RL spectra of our NSFs in 2–18 GHz interval. There is one RL minima for each sample that is centered between 7 and 10.5 GHz. As amount of doped concentration increases, the frequency at which RL occurs increases slightly. Similarly, microwave absorption capacity enhanced from −28.5 dB to −40 dB. The absorption bandwidth at −10 dB lies between 5 and 8.4 GHz, being wider in highly doped samples (see Table 5). This means that more than 90% of the incoming microwave energy is dissipated within the material in a wide frequency range that is very beneficial feature for good quality absorbers. The optimum doping concentration appeared to be x = 0.08 considering both RL and absorption bandwidth parameters.
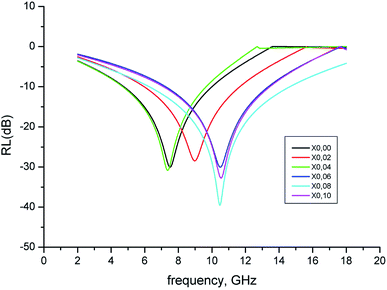 |
| Fig. 9 RL spectra of Ni0.4Cu0.2Zn0.4LaxYxFe2−xO4 (x = 0.00 − 0.10) NSFs. | |
Table 5 The important parameters reflecting the MW properties of Ni0.4Cu0.2Zn0.4LaxYxFe2−xO4 (x = 0.00 − 0.10) NSFs
x
|
RL (dB) |
f (GHz) |
Δf (GHz) |
0.00 |
29.9 |
7.5 |
5.3 |
0.02 |
28.5 |
9.0 |
5.8 |
0.04 |
30.8 |
7.4 |
5.0 |
0.06 |
30.0 |
10.5 |
6.2 |
0.08 |
39.5 |
10.5 |
8.4 |
0.10 |
32.6 |
10.5 |
6.5 |
Dielectric (tan
δε) and magnetic (tan
δμ) loss Ni0.4Cu0.2Zn0.4LaxYxFe2−xO4 (x = 0.00 − 0.10) NSFs (Fig. 10). It implies that at low frequencies up to 4 GHz both dielectric and magnetic loss tangents are effective, while at high frequencies between 15 and 17 GHz, only dielectric loss plays role in dissipation. However, in RL spectra, there are no reflection minima at these frequencies meaning that main microwave absorption mechanism was not loss tangents. The possible dissipation mechanism can be explained by dipolar relaxation and spin rotation as follows. The existence of cations with dissimilar valences (Zn2+, Ni2+, Fe2+/Fe3+ together with O2−) generates dipoles with diverse strength in the unit cells of LaY doped NiCuZn ferrite NSFs. The conductance loss and dielectric loss are two dominant kinds of energy dissipation as expressed here:
where
ε0 is the permittivity of free space,
ω is the pulsation,
σ is the conductivity, and

is the relaxation polarization. In our case, where the magnetic materials are poor conductors, the crucial mechanism of dielectric loss in absorbing the microwave energy come to be the Debye dipolar relaxation.
55,56 Generally, polycrystalline ferrites have a permeability correlated with two magnetizing mechanisms,
i.e. domain wall motion and spin rotation.
57,58 The first one is influenced by the size of grains wherein the increase in the size of domain walls will contribute to increase the magnetization. The contribution of the domain walls to the permeability consists of resonance and influenced to frequency.
59 It is effective at lower frequencies below 100 MHz.
56 Instead, the contribution of spin rotation is of resonance that occurs at high frequencies.
60 Therefore, the observed RL minimum around 10 GHz can also be explained dissipation due to the spin rotation. According to the obtained results, one can claim that the Ni
0.4Cu
0.2Zn
0.4La
xY
xFe
2−xO
4 (
x = 0.00 − 0.10) NSFs could be utilized as radar absorbing materials in X-band due to the high RL and large absorbing bandwidth.
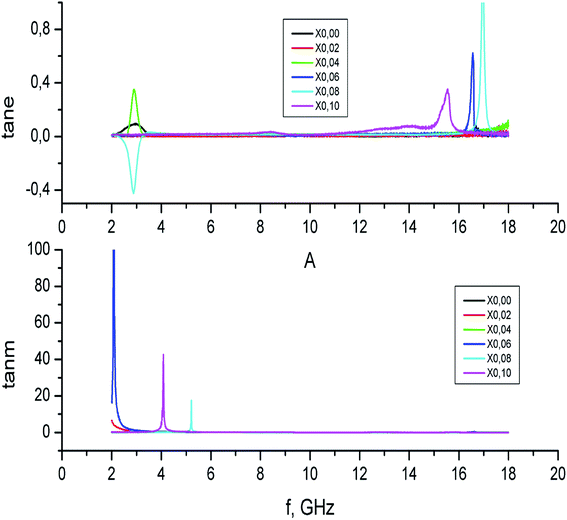 |
| Fig. 10 Dielectric (above) and magnetic (below) loss tangents of Ni0.4Cu0.2Zn0.4LaxYxFe2−xO4 (x = 0.00 − 0.10) NSFs. | |
4. Conclusion
The influence of La–Y substitution on the structure, optical, magnetic and microwave properties of NiCuZn NSFs on were investigated in detail. With raising the amount of La–Y, the lattice parameters and crystallite size increase due the enlargement of crystal. Tauc plots assign direct and allowed electronic transitions for all NiCuZn mixed nanoferrite samples. Eg values in a range of 1.87 eV–1.92 eV also reveal the semiconducting nature of nanoferrite samples. La3+ and Y3+ ions substitution process with x = 0.02 − 0.10 concentrations slightly increased the order of Eg data. The Mössbauer results revealed that the hyperfine field at A and B sites decreases with substitution of La3+ and Y3+ in Fe3+ because of the replacement of magnetic Fe3+ (with magnetic moment of 5 μB) ions by nonmagnetic La3+ and Y3+ ions. The magnetic properties of various nanoparticles were analyzed at T = 300 K and 10 K. All prepared nanoparticles exhibit superparamagnetic behavior at RT and soft ferromagnetic trait at 10 K. It is noticed that the deduced magnetic parameters of all the produced nanoparticles are higher at low temperature than that of RT, owing to the reduced thermal fluctuations and surface spin disorders at the surfaces of the nanoparticles. It is observed that Ms and Mr decrease and Hc increases slightly with La3+ and Y3+ substitutions. The reduction in magnetization is attributed to reduction in crystallites size, zero magnetic moments of La3+ and Y3+ ions substituting magnetic. Microwave characterization of Ni0.4Cu0.2Zn0.4LaxYxFe2−xO4 (x = 0.00 − 0.10) NSFs showed that RL occurs at different frequencies between 7.5 and 10.5 GHz. The LaY doping shifted the RL minimum to higher frequencies. Besides, absorption bandwidth increased with LaY amount reaching to 8.4 GHz @ 10 GHz in x = 0.08 sample. Also, this product has the better microwave capability with RL of −40 dB @ 10.5 GHz. Therefore, the Ni0.4Cu0.2Zn0.4LaxYxFe2−xO4 (x = 0.00 − 0.10) NSFs could be utilized as radar absorbing materials in X-band due to the high RL and large absorbing bandwidth.
Conflicts of interest
There are no conflicts to declare.
References
- G. Gan, H. Zhang, Q. Li, J. Li, X. Huang and F. Xie,
et al., Low loss, enhanced magneto-dielectric properties of Bi2O3 doped Mg–Cd ferrites for high frequency antennas, J. Alloys Compd., 2018, 735, 2634 CrossRef CAS.
- K. K. Kefeni, T. A. Msagati and B. B. Mamba, Ferrite nanoparticles: synthesis, characterisation and applications in electronic device, Mater. Sci. Eng., B, 2017, 215, 37 CrossRef CAS.
- D.-H. Kim, H. Zeng, T. C. Ng and C. S. Brazel, T1 and T2 relaxivities of succimer-coated MFe2O4 (M = Mn2+, Fe2+ and Co2+) inverse spinel ferrites for potential use as phase-contrast agents in medical MRI, J. Magn. Magn. Mater., 2009, 321, 3899 CrossRef CAS.
- J. Lu, S. Ma, J. Sun, C. Xia, C. Liu and Z. Wang,
et al., Manganese ferrite nanoparticle micellar nanocomposites as MRI contrast agent for liver imaging, Biomaterials, 2009, 30, 2919 CrossRef CAS.
- V. Pilati, R. Cabreira Gomes, G. Gomide, P. Coppola, F. G. Silva and FbL. Paula,
et al., Core/shell nanoparticles of non-stoichiometric Zn–Mn and Zn–Co ferrites as thermosensitive heat sources for magnetic fluid hyperthermia, J. Phys. Chem. C, 2018, 122, 3028 CrossRef CAS.
- N. Sanpo, C. C. Berndt, C. Wen and J. Wang, Transition metal-substituted cobalt ferrite nanoparticles for biomedical applications, Acta Biomater., 2013, 9, 5830 CrossRef CAS.
- I. Sharifi, H. Shokrollahi and S. Amiri, Ferrite-based magnetic nanofluids used in hyperthermia applications, J. Magn. Magn. Mater., 2012, 324, 903 CrossRef CAS.
- M. D. Shultz, S. Calvin, P. P. Fatouros, S. A. Morrison and E. E. Carpenter, Enhanced ferrite nanoparticles as MRI contrast agents, J. Magn. Magn. Mater., 2007, 311, 464 CrossRef CAS.
- C. Singh, A. Goyal and S. Singhal, Nickel-doped cobalt ferrite nanoparticles: efficient catalysts for the reduction of nitroaromatic compounds and photo-oxidative degradation of toxic dyes, Nanoscale, 2014, 6, 7959 RSC.
- K. Ramakrishna, C. Srinivas, B. Tirupanyam, P. Ramesh, S. Meena and D. Potukuchi,
et al., Effect of Cu2+ substitution on the magnetic properties of co-precipitated Ni–Cu–Zn ferrite nanoparticles, AIP Conf. Proc., 2017, 1832, 050154 CrossRef.
- M. P. Reddy, W. Madhuri, N. R. Reddy, K. V. S. Kumar, V. R. K. Murthy and R. R. Reddy, Influence of copper substitution on magnetic and electrical properties of MgCuZn ferrite prepared by microwave sintering method, Mater. Sci. Eng., C, 2010, 30, 1094 CrossRef CAS.
- S. More, R. Kadam, A. Kadam, D. Mane and G. Bichile, Structural properties and magnetic interactions in Al3+ and Cr3+ co-substituted CoFe2O4 ferrite, Open Chem., 2010, 8, 419 CAS.
- X. C. Zhong, X. J. Guo, S. Y. Zou, H. Y. Yu, Z. W. Liu and Y. F. Zhang,
et al., Improving soft magnetic properties of Mn–Zn ferrite by rare earth ions doping, AIP Adv., 2017, 8, 047807 CrossRef.
- R. Tholkappiyan and K. Vishista, Influence of lanthanum on the optomagnetic properties of zinc ferrite prepared by combustion method, Phys. B, 2014, 448, 177 CrossRef CAS.
- R. Kershi, F. Ali and M. Sayed, Influence of rare earth ion substitutions on the structural, optical, transport, dielectric, and magnetic properties of superparamagnetic iron oxide nanoparticles, J. Adv. Ceram., 2018, 7, 218 CrossRef CAS.
- J. Hu and M. Yan, Preparation of high-permeability NiCuZn ferrite, J. Zhejiang Univ., Sci., B, 2005, 6, 580 CrossRef.
- S. M. Kabbur, U. R. Ghodake, R. C. Kambale, S. D. Sartale, L. P. Chikhale and S. S. Suryavanshi, Magnetic, Electric and Optical Properties of Mg-Substituted Ni–Cu–Zn Ferrites, J. Electron. Mater., 2017, 46, 5693 CrossRef CAS.
- T. Krishnaveni, B. R. Kanth, V. S. R. Raju and S. R. Murthy, Fabrication of multilayer chip inductors using Ni–Cu–Zn ferrites, J. Alloys Compd., 2006, 414, 282 CrossRef CAS.
- M. M. Eltabey, K. M. El-Shokrofy and S. A. Gharbia, Enhancement of the magnetic properties of Ni–Cu–Zn ferrites by the non-magnetic Al3+-ions substitution, J. Alloys Compd., 2011, 509, 2473 CrossRef CAS.
- H. Harzali, F. Saida, A. Marzouki, A. Megriche, F. Baillon and F. Espitalier,
et al., Structural and magnetic properties of nano-sized NiCuZn ferrites synthesized by co-precipitation method with ultrasound irradiation, J. Magn. Magn. Mater, 2016, 419, 50 CrossRef CAS.
- H. Harzali, F. Baillon, O. Louisnard, F. Espitalier and A. Mgaidi, Sono-crystallization of ZnSO4·7H2O with variation of solution heights, Chem. Eng. J., 2012, 195, 332 CrossRef.
- M. A. Almessiere, Y. Slimani, A. D. Korkmaz, H. Gungunes, M. Nawaz, S. E. Shirsath, A. Baykal and I. Ercan, Ca2+/Mg2+ co-substituted strontium nanohexaferrites: magnetic investigation and Mossbauer analysis, J. Sol-Gel Sci. Technol., 2019, 92, 239–251 CrossRef CAS.
- A. Sadaqat, M. Almessiere, Y. Slimani, S. Guner, M. Sertkol, H. Albetran, A. Baykal, S. E. Shirsath, B. Ozcelik and I. Ercan, Structural, optical and magnetic properties of Tb3+ substituted Co nanoferrites prepared via sonochemical approach, Ceram. Int., 2019 DOI:10.1016/j.ceramint.2019.07.280.
-
S. E. Shirsath, D. Wang, S. S. Jadhav, M. L. Mane, and S. Li, Ferrites obtained by sol–gel method, ed. L. Klein, M. Aparicio and A. Jitianu, Handbook of Sol–Gel Science and Technology, Springer, Cham, 2018, pp. 695–735 Search PubMed.
- W. Wei, W. Ye, J. Wang, C. Huang, J.-B. Xiong, H. Qiao, S. Cui and L. Mi, Hydrangea-like α-Ni1/3Co2/3(OH)2 Reinforced by Ethyl Carbamate“Rivet” for All-Solid-State Supercapacitors with Outstanding Comprehensive Performance, ACS Appl. Mater. Interfaces, 2019, 11(35), 32269–32281 CrossRef CAS.
- W. Wei, W. Chen, L. Ding, S. Cui and L. Mi, Construction of hierarchical three-dimensional interspersed flower-like nickel hydroxide for asymmetric super capacitors, Nano Res., 2017, 10(11), 3726–3742 CrossRef CAS.
- W. Wei, J. Wu, S. Cui, Y. Zhao, W. Chen and L. Mi, α-Ni(OH)2/NiS1.97 heterojunction composites with excellent ion and electron transport properties for advanced super capacitors, Nanoscale, 2019, 11, 6243–6253 RSC.
- J. Tauc, R. Grigorovici and A. Vancu, Optical properties and electronic structure of amorphous germanium, Phys. Status Solidi, 1966, 15, 627 CrossRef CAS.
- A. D. Korkmaz, S. Güner, Y. Slimani, H. Gungunes, Md. Amir, A. Manikandan and A. Baykal, Microstructural, optical, and magnetic properties of vanadium-substituted nickel spinel nanoferrites, J. Supercond. Novel Magn., 2019, 32, 1057 CrossRef CAS.
- M. A. Almessiere, Y. Slimani, A. D. Korkmaz, S. Guner, M. Sertkol, S. E. Shirsath and A. Baykal, Structural, optical and magnetic properties of Tm3+ substituted cobalt spinel ferrites synthesized via sonochemical approach, Ultrason. Sonochem., 2019, 54, 1 CrossRef CAS PubMed.
- Y. Slimani, M. A. Almessiere, M. Nawaz, A. Baykal, S. Akhtar, I. Ercan and I. Belenli, Effect of bimetallic (Ca, Mg) substitution on magneto-optical properties of NiFe2O4 nanoparticles, Ceram. Int., 2019, 45, 6021 CrossRef CAS.
- Y. Slimani, M. A. Almessiere, M. Sertkol, S. E. Shirsath, A. Baykal, M. Nawaz, S. Akhtar, B. Ozcelik and I. Ercan, Structural, magnetic, optical properties and cation distribution of nanosized Ni0.3Cu0.3Zn0.4TmxFe2−xO4 (0.0 ≤ x ≤ 0.10) spinel ferrites synthesized by ultrasound irradiation, Ultrason. Sonochem., 2019, 57, 203 CrossRef CAS.
- L. George, C. Viji, H. Mathew and E. M. Mohammed, Structural, Dielectric, Magnetic and Optical Properties of Cerium Substituted Ni–Zn Mixed Ferrite, Mater. Sci. Res. India, 2017, 14, 133 CAS.
- R. J. Joseyphus, A. Narayanasamy, K. Shinoda, B. Jeyadevan and K. Tohji, Synthesis and magnetic properties of the size-controlled Mn–Zn ferrite nanoparticles by oxidation method, J. Phys. Chem. Solids, 2006, 67, 1510 CrossRef CAS.
- A. Lakshman, P. S. V. Subba Rao and K. H. Rao, Mössbauer spectroscopic analyses of Mg0.9Cu0.1Mn0.05CrxFe1.95−xO4 spinel ferrites, Mater. Lett., 2006, 60, 7 CrossRef CAS.
- H. Kumar, R. C. Srivastava, j. P. Singh, P. Negi, H. M. Agrawal, D. Das and K. H. Chae, Structural and magnetic study of dysprosium substituted cobalt ferrite nanoparticles, J. Magn. Magn. Mater., 2016, 401, 16 CrossRef CAS.
- M. A. Almessiere, Y. Slimani, S. Güner, J. van Leusen, A. Baykal and P. Kögerler, Effect of Nb3+ ion substitution on the magnetic properties of SrFe12O19 hexaferrites, J. Mater. Sci.: Mater. Electron., 2019, 30, 11181 CrossRef CAS.
- M. A. Almessiere, Y. Slimani, H. Gungunes, A. Manikandan and A. Baykal, Investigation of the effects of Tm3+ on the structural, microstructural, optical, and magnetic properties of Sr hexaferrites, Results Phys., 2019, 13, 102166 CrossRef.
- M. A. Almessiere, Y. Slimani, H. S. El Sayed and A. Baykal, Morphology and magnetic traits of strontium nanohexaferrites: effects of manganese/yttrium co-substitution, J. Rare Earths, 2019, 37, 732–740 CrossRef CAS.
- V. Chaudhari, S. E. Shirsath, M. L. Mane, R. H. Kadam, S. B. Shelke and D. R. Mane, Crystallographic, magnetic and electrical properties of Ni0.5Cu0.25Zn0.25LaxFe2−xO4 nanoparticles fabricated by sol–gel method, J. Alloys Compd., 2013, 549, 213 CrossRef CAS.
- P. K. Roy and J. Bera, Electromagnetic properties of samarium-substituted NiCuZn ferrite prepared by auto-combustion method, J. Magn. Magn. Mater., 2009, 321, 247 CrossRef CAS.
- F. Liu, C. Yang, T. Ren, A. Z. Wang, J. Yu and L. Liu, NiCuZn ferrite thin films grown by a sol–gel method and rapid thermal annealing, J. Magn. Magn. Mater., 2007, 309, 75 CrossRef CAS.
- M. A. Almessiere, Y. Slimani, S. Güner, A. Baykal and I. Ercan, Effect of dysprosium substitution on magnetic and structural properties of NiFe2O4 nanoparticles, J. Rare Earths, 2019, 37, 871 CrossRef CAS.
- M. A. Almessiere, Y. Slimani, S. Güner, M. Nawaz, A. Baykal, F. Aldakheel, S. Akhtar, I. Ercan, İ. Belenli and B. Ozçelik, Magnetic and structural characterization of Nb3+-substituted CoFe2O4 nanoparticles, Ceram. Int., 2019, 45, 8222 CrossRef CAS.
- C. Murugesan and G. Chandrasekaran, Impact of Gd3+ substitution on the structural, magnetic and electrical properties of cobalt ferrite nanoparticles, RSC Adv., 2015, 5, 73714 RSC.
- M. A. Almessiere, Y. Slimani, S. Guner, M. Nawaz, A. Baykal, F. Aldakheel, A. Sadaqat and I. Ercan, Effect of Nb substitution on magneto-optical properties of Co0.5Mn0.5Fe2O4 nanoparticles, J. Mol. Struct., 2019, 1195, 269 CrossRef CAS.
- M. A. Almessiere, Y. Slimani, M. Sertkol, F. A. Khan, M. Nawaz, H. Tombuloglu, E. A. Al-Suhaimi and A. Baykal, Ce–Nd Co-substituted nanospinel cobalt ferrites: an investigation of their structural, magnetic, optical, and apoptotic properties, Ceram. Int., 2019, 45, 16147 CrossRef CAS.
- M. A. Almessiere, A. D. Korkmaz, Y. Slimani, M. Nawaz, S. Ali and A. Baykal, Magneto-optical properties of Rare Earth metals substituted Co–Zn spinel nanoferrites, Ceram. Int., 2019, 45, 3449 CrossRef CAS.
- M. A. Almessiere, Y. Slimani, U. Kurtan, S. Guner, M. Sertkol, S. E. Shirsath, S. Akhtar, A. Baykal and I. Ercan, Structural, magnetic, optical properties and cation distribution of nanosized Co0.7Zn0.3TmxFe2−xO4 (0.0 ≤ x ≤ 0.04) spinel ferrites synthesized by ultrasonic irradiation, Ultrason. Sonochem., 2019, 58, 104638 CrossRef CAS.
- M. A. Almessiere, Y. Slimani, A. D. Korkmaz, N. Taskhandi, M. Sertkol, A. Baykal, S. E. Shirsath, İ. Ercan and B. Ozçelik, Sonochemical synthesis of Eu3+ substituted CoFe2O4 nanoparticles and their structural, optical and magnetic properties, Ultrason. Sonochem., 2019, 5, 104621 CrossRef.
- M. A. Almessiere, Y. Slimani, M. Sertkol, M. Nawaz, A. Sadaqat, A. Baykal, I. Ercan, B. Ozçelik and B. Ozçelik, Effect of Nb3+ Substitution on the Structural, Magnetic, and Optical Properties of Co0.5Ni0.5Fe2O4 Nanoparticles, Nanomaterials, 2019, 9, 430 CrossRef CAS.
- M. A. Almessiere, Y. Slimani, A. Demir Korkmaz, M. Sertkol, A. Baykal, I. Ercan and B. Özçelik, Sonochemical Synthesis of CoFe2−xNdxO4 Nanoparticles: Structural, Optical, and Magnetic Investigation, J. Supercond. Novel Magn., 2019, 1–8 CrossRef CAS.
- Y. Slimani, M. A. Almessiere, S. Güner, N. A. Tashkandi, A. Baykal, M. F. Sarac, M. Nawaz and I. Ercan, Calcination effect on the magneto-optical properties of vanadium substituted NiFe2O4 nanoferrites, J. Mater. Sci.: Mater. Electron., 2019, 30, 9143 CrossRef CAS.
- M. A. Almessiere, Y. Slimani, S. Guner, M. Sertkol, A. Demir Korkmaz, S. E. Shirsath and A. Baykal, Sonochemical Synthesis and Physical Properties of Co0.3Ni0.5Mn0.2EuxFe2−xO4 nano-spinel ferrites, Ultrason. Sonochem., 2019, 58, 104654 CrossRef CAS.
- S. He, G.-S. Wang, C. Lu, J. Liu, B. Wen, H. Liu, L. Guo and M.-S. Cao, Enhanced wave absorption of nanocomposites based on the synthesized complex symmetrical CuS nanostructure and poly(vinylidene fluoride), J. Mater. Chem. A, 2013, 1, 4685 RSC.
- D. Chen, H. Quan, G. S. Wang and L. Guo, Hollow α-MnS Spheres and Their Hybrids with Reduced Graphene Oxide: Synthesis, Microwave Absorption, and Lithium Storage Properties, ChemPlusChem, 2013, 78, 843 CrossRef CAS.
-
S. Chikazumi, Physics of Ferromagnetism (International Series of Monographs on Physics, Book 94), Oxford University Press, 2nd edn, 2009, ISBN-10:0199564817 Search PubMed.
- D. Stoppels, Developments in soft magnetic power ferrites, J. Magn. Magn. Mater., 1996, 160, 323 CrossRef CAS.
- T. Nakamura, T. Miyamoto and Y. Yamada, Complex permeability spectra of polycrystalline Li–Zn ferrite and application to EM-wave absorber, J. Magn. Magn. Mater., 2003, 256, 340 CrossRef CAS.
- G. T. Rado, R. W. Wright and W. H. Emerson, Ferromagnetism at very high frequencies. III. Two mechanisms of dispersion in a ferrite, Phys. Rev., 1950, 80, 273 CrossRef.
|
This journal is © The Royal Society of Chemistry 2019 |