DOI:
10.1039/C9RA05923G
(Paper)
RSC Adv., 2019,
9, 34559-34566
TiBALDH as a precursor for biomimetic TiO2 synthesis: stability aspects in aqueous media†
Received
30th July 2019
, Accepted 17th October 2019
First published on 28th October 2019
Abstract
Titanium(IV) bis(ammonium lactate)dihydroxide (TiBALDH) is a commercially available reagent frequently used to synthesize TiO2. Particularly, for the biomimetic synthesis of TiO2, TiBALDH is the preferred precursor because it can be mixed in aqueous solutions with no apparent hydrolysis or condensation reactions. Thus, proteins or other biomolecules can be used as a template in aqueous systems for the synthesis of TiO2 from TiBALDH. Nevertheless, there is evidence that TiBALDH is in equilibrium with TiO2, and even, the principal structure of the complex has been suggested as [Ti4O4(lactate)8]8−. Since that chemical equilibrium depends on the polarity of the solvent, in this work we explored a diversity of media to test the chemical stability of TiBALDH and its equilibrium with TiO2 at room temperature. TiBALDH (2.078 M) contains particles of 18.6 ± 7.3 nm in size, if it is diluted with deionized water, the particles reach a size of 5.2 ± 1.7 nm, which suggest that intermolecular interactions form polymers of titanium lactate complexes reversibly, reaching equilibrium after 10 hours. Typical buffer systems were tested; TiBALDH reacted rapidly only with phosphate groups, even if the source came from DNA. Therefore, phosphate buffer must be avoided in biomineralization TiO2 synthesis. In solutions of TiBALDH at basic pH, condensation reactions are promoted to form a gel containing anatase nanoparticles, but if the solutions are acidic, monodisperse anatase nanoparticles of ∼5 nm were observed. The results show that the commercial reagent TiBALDH contains many species of titanium lactate complexes in equilibrium with TiO2, and it is affected by the concentration, time, pH, and several ions. This peculiar behavior must be taken into account when this precursor is used and it could be useful to develop novel synthesis routes of macrostructures with biomolecules in aqueous systems.
Introduction
Biological organisms over millions of years of evolution, have been able to generate and adapt inorganic materials according to their requirements, achieving remarkable control in their size, shape, and composition. This process, known as biomineralization, is performed at ambient temperature and pressure, in aqueous media.1 The mild conditions for this process are why this “natural or biological” method is being replicated in the laboratory. Thus, biomolecules are an astonishing source of inspiration because they have well defined sizes, sophisticated shapes and purposeful activities. Recently, biomimetic syntheses of metallic nanoparticles,2–5 and semiconductors,6,7 have been successfully achieved using a variety of biomolecules such as proteins,8 DNA,9 and macrostructures.10,11 Importantly, this technique has the advantage of avoiding organic solvents that can alter the chemical and structural stability of biomolecules.12
Being titanium oxide a material with interesting properties and technological applications in fields such as pigments industries, (photo)catalysis,13 energy production and storage,14,15 among others, its synthesis by biomimetic techniques has been explored.16 The major research in this field has used TiO2 as target, to identify peptide sequences that have affinity to this material and subsequent use as template to guide its biomineralization. However, most activity rest in the study of the peptide's characteristics, such as sequence order, existence of basic residues (charges) or configuration,17–20 minimizing the chemical properties of the Ti precursor in the final material. Although some efforts for biomineralize TiO2 from Ti alkoxides precursors have been reported,21,22 groups around the world routinely employed titanium(IV) bis(ammonium lactate)dihydroxide (TiBALDH) as source of Ti. This popularity obeys that TiBALDH is reported as water-soluble, stable a neutral pH and ambient conditions.23–28
It is clear that its chemical properties make the TiBALDH a good candidate for (TiO2) biomineralization experiments. However, several aspects of its chemistry must be considered before adopting its use systematically. The majority of works assume a molecular structure [Ti[(lactate)OH]2]2− and propose mechanism of reactions based on this structure,24 but evidence indicates that species of [Ti4O4(lactate)8]8− coexist with [Ti(lactate)3]2− in TiBALDH solutions, which suggest that a chemical equilibrium occur.29,30 In addition, TiO2 is also present in the equilibrium.31 Then, TiBALDH is a complex “mixture” of different species that must be considered in biomineralization experiments. On the one hand to assure the reproducibility of the method and on the other one to propose a more realistic reaction mechanism.
Some efforts to understand how several factors affect the chemical equilibrium have been studied. Kessler et al. studied the stability of TiBALDH,30 as a function of solvent polarity, demonstrating that the equilibrium can be shifted by the addition of polar solvents to form TiO2 nanoparticles reversibly (whose results have been reproduced in our laboratory). More recently, factors as pH and time were explored and a kinetic model proposed for the formation of TiO2 particles.31 Then, being the TiBALDH the preferred precursor for TiO2 biomineralization experiments, its (pre)existence in the equilibrium and the lack of systematic studies about it, inspire us this work. Thus, our main aim was explore several factors that affect the equilibrium and should be taken into account carefully when we use TiBALDH in biomineralization reactions. Between the main experimental issues, we considered buffer system (composition and concentration), pH value and ions (chemical species) used to adjust it and TiBALDH concentration itself. Moreover, these experiments should be made without proteins and peptides to determine their effect in the mineralization process. Then, we found that phosphate ions are appropriate only if they are integrated into biomolecules, for example DNA, to template TiO2 nanostructures.
Experimental section
Stability of TiBALDH in aqueous media
TiBALDH (titanium(IV) bis(ammonium lactate)dihydroxide) was purchased from Sigma-Aldrich, it comes to 50% in water and we used two distinct lots (Lot. MKBP1038V and MKCC1000, stored at 4 °C) to ensure the reliability of this reagent. All experiments were conducted at room temperature unless otherwise specified. We prepared aqueous solutions of TiBALDH as follows:
(a) TiBALDH-water: the effect of the concentration was studied by preparing solutions of TiBALDH at concentrations between 1.0 M and 1.0 × 10−3 M, deionized water (≥18.2 MΩ) was used as the solvent.
(b) TiBALDH-ions: the stability of TiBALDH (50 mM) was studied in the following systems: water only (negative control, NC), water pH 4, water pH 8.5, sodium acetate buffer (AB) (100 mM, pH 4.5), sodium citrate buffer (CB) (100 mM, pH 5.5), 2-(N-morpholino)ethanesulfonic acid (MES) buffer (MB) (100 mM, pH 6.0), sodium phosphate buffer (PB) (100 mM, pH 7.0), tris(hydroxymethyl)aminomethane buffer (TB) (100 mM, pH 8.0), sodium sulfate (100 mM), sodium nitrate (100 mM), and sodium chloride (100 mM).
(c) TiBALDH-PB: to measure the changes in the particle size in the presence of phosphate ions, TiBALDH (2.078 M) was added to PB buffer at different concentrations. Also, phosphoric acid and circular double strained DNA were used as a phosphate source. The DNA was obtained from vector M13mp18 (New England Biolabs). The vector was transformed in E. coli XL1-blue, purified by miniprep and dissolved in deionized water. The concentration of DNA was measured by spectroscopic absorption at 260 nm.
(c) TiBALDH-pH: the influence of pH in the TiBALDH equilibrium was studied preparing aqueous solutions of TiBALDH at different concentrations; the pH was adjusted with NaOH or HCl (1.0 M or 5.0 M, depending on the concentration of TiBALDH).
Characterization
The particle size of all solutions was measured by dynamic light scattering (ZetaSizer ZSP, Malvern Instruments) at 25 °C using the measurement position of 4.65 mm with automatic attenuation and the “general purpose” mode, 12 runs of 10 seconds each were performed. All mean particle sizes were taken from the intensity results. The amount of soluble Ti(IV) was measured by a colorimetric method with Tiron (4,5-dihydroxy-1,3-benzenedisulfonic acid) 5.0 mM in acetate buffer pH 4.5.32 The relative content of Ti(IV) was obtained by comparing with the content of soluble Ti(IV) obtained in the solution of TiBALDH in water only. The crystallinity and morphology of TiO2 were observed by transmission electron microscopy (TEM) using a JEOL 2010 instrument operated at 200 kV. Two microliters of the sample were deposited in a copper grid covered with a holey carbon film (300 mesh, Agar Scientific), and dried at room temperature.
Note: all prepared solutions of TiBALDH (TiBALDH-water, TiBALDH-ions, TiBALDH-TiBALDH-PB, TiBALDH-Tiron, TiBALDH-pH) were capped and incubated at least for 24 h at room temperature and made by triplicate.
Results and discussions
TiBALDH originally was reported as stable in water, but now we know that the principal molecular form of TiBALDH in aqueous solution is [Ti4O4(lactate)8]8− which is stabilized by ammonium ions, in equilibrium with TiO2 according to eqn (1).30 |
3[Ti4O4(lactate)8]8− + 8NH4+ ⇄ 8[Ti(lactate)3]2− + 4TiO2 + 8NH3 + 4H2O
| (1) |
Then, factors that affect this equilibrium, such as concentration, ions, and pH should be studied to improve the design of biomineralization synthesis from TiBALDH.
Concentration-effect
We studied the progress of the particles in TiBALDH aqueous solutions by dynamic light scattering (DLS) measurements. First, we studied the effect in size as a function of TiBALDH concentration using water only. The commercial solution as received (2.078 M), contains principally nanoparticles of 18.6 ± 7.3 nm in size (Fig. 1). A decrease of the concentration is accompanied by a reduction in the size particle as small as 5.2 nm ± 1.7 nm, in accordance with the reported by others authors,30,33 and remains practically constant to concentrations ≤ 100 mM. When diluted solutions are re-concentrated by evaporation (at 40 °C under vacuum) there is an increase of the viscosity, precluding complete solvent evaporation. From these results is evident that solutions at low concentration of TiBALDH (≤100 mM) contain stable titanium species (complexes and/or oxide) and the intermolecular interactions are negligible, meanwhile in concentrated solutions, lactates could promote the formation and growth of micelles templated by self-assembly of ligands (MTSALs),22,34,35 which would explain its reversible nature. This behavior is similar to the vitreous transition of polymers or ionic liquids, probably hydrogen bonds hamper the total water evaporation resulting in a very stable structure.36,37 In addition, scattering of TiO2 nanoparticles was followed by absorbance measurements at 500 nm, kept constant in all the concentration interval, suggesting that the number of particles did not change with TiBALDH concentration.
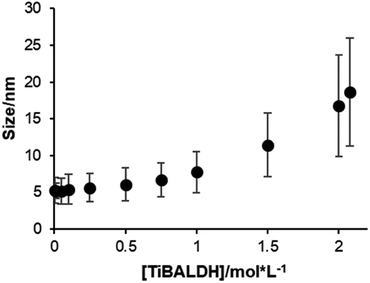 |
| Fig. 1 Size of particles measured by DLS of aqueous solutions of TiBALDH at different concentrations. | |
The changes in size by diluting the TiBALDH solution can be observed immediately after dilution. Nevertheless, the concentration of soluble Ti(IV) changes along time, suggesting that the rate to reach the equilibrium is slow. Fig. 2 shows the relative concentration of Ti(IV) at different times after dilution. Evidently, the concentration of Ti(IV) decreases immediately after dilution and the new equilibrium is reached after 10 h. This behavior can be explained in terms of the eqn (1) because the titanium complex [Ti4O4(lactate)8]8− transforms to TiO2, decreasing the amount of soluble Ti(IV). Then if TiBALDH is diluted, more TiO2 is formed. Since experimental data do not fit with any simple kinetic equilibrium, we consider that there exists more than one step towards equilibrium.
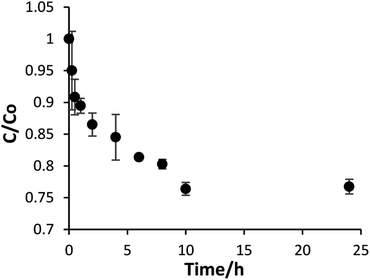 |
| Fig. 2 Variation of the soluble Ti(IV) along time after dilution from commercial TiBALDH to a final concentration of 50 mM. | |
Then, reaction with diluted TiBALDH should be prepared at least 10 h before its use in order to ensure the reproducibility in each experiment.
To gain insights on the molecular composition of the chemical species in the diluted solution of TiBALDH, measurements by mass spectrometry were performed. In solutions of TiBALDH 50 mM, it was not possible to perform the characterization because of the saturation of signals. Thus, more diluted TiBALDH solutions (0.1 mM) were examined, the mass spectrum (ESI S1†) shows a diversity of species with different ratio m/z. Remarkably, differences in mass of 90 and 72 Da are typical, which can be attributed to lactic acid and polylactide (monomer: 72 Da) respectively. Then, TiO2 nanoparticles should be capped (stabilized) by lactate ligands as other authors reported. Values of mass > 500 Da were typical and the peak corresponding to [Ti4O4(lactate)8]8− was not observed, probably due to the high electric charge, which implies a peak m/z of ∼120 (molecular mass ∼968 Da). In the solid-state, complexes of titanium or zirconium lactate have been characterized.38,39 However, in aqueous solution, the lactate complexes possibly cannot exist as monomers; instead, they form micelles templated by self-assembly of ligands (MTSALs),22,35 which probably hamper reactions of condensation of the metal centers. Then, effectively at the equilibrium there are titanium–oxolactates species and TiO2 nanoparticles, but only the first ones could change in size.
Ions effects
Considering the slow rate to reach the equilibrium after each dilution, all further experiments were made using TiBALDH (50 mM) as standard concentration and aging for 24 h. The influence of different salts in the size (stability) of the particles was measured by DLS in presence of acetate (AB), phosphate (PB) and tris (TB), citrate (CB) and MES (MB) buffer and compared with water as the negative control (NC). After incubation with the buffers, no changes were observed with AB, TB, CB, and MB regarding water, only phosphate ions showed an important shift in the size of the particle as is observed in Fig. 3a. This behaviour agreed with the reported by other authors that claim that peptides in presence of PB are able to induce TiO2 biomineralization from TiBALDH.27,33,40–43 However, the final product usually is amorphous and contaminated with phosphorous, in our case, we observed the formation of a precipitate, which was not further analysed because is out of the scope of this work.
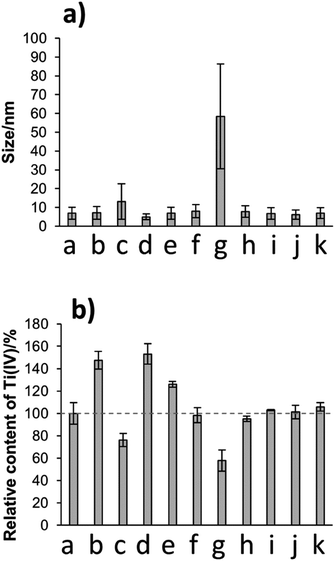 |
| Fig. 3 (a) Size of particles in solutions of TiBALDH 50 mM in the selected aqueous systems. (b) Relative content of soluble Ti(IV) in TiBALDH 50 mM in the correspondent aqueous system: (a) deionized water (NC), (b) water pH 4.0, (c) water pH 8.5, (d) acetate buffer (AB), (e) citrate buffer (CB), (f) MES buffer (MB), (g) phosphate buffer (PB), (h) tris buffer (TB), (i) Na2SO4, (j) NaNO3, (k) NaCl. | |
Also, the amount of Ti(IV) free (soluble) was quantified by colorimetric assay,32 as is shown in Fig. 3b the amount of Ti decreases visibly in PB comparing with the NC (water), implying that Ti species were employed in the formation of titanium oxide/phosphate titanium oxide. Whereas in other systems buffer no change significantly in size was observed, remarkably the amount of Ti soluble rise for AB and CB. Apparently, the addition of these ligands (acetate and citrate) is able to dissolve titanium oxide. Such comportment is concentration-dependent in the case of citrate, even at concentrations as low as 0.005 mM and can take just a few minutes.44
Changes on pH were important only to value 8.5, where a size shift is detected. When the pH is basic, the ammonium ions are transformed into ammonia gas, which promotes the condensation of complexes according to (1). To contrast the changes in each value pH, we take the quantity of soluble Ti(IV) in the solution of TiBALDH 50 mM in water as a reference, and the variations in the content of Ti(IV) are seen in Fig. 3b. In the system at pH 8.5, the amount of soluble Ti(IV) resulted lower than in water. This is consistent with the increase of size because the soluble Ti(IV) reacts to form insoluble TiO2. On the other hand, the amount of soluble Ti(IV) increased in the systems in acid medium (pH 4.0), AB (pH 4.5) and CB (pH 5.5). In these systems, although there is no change in the particle size, the pH can affect the solubility of TiO2 by changing the chemical equilibrium to the left of eqn (1). The presence of neutral salts (Na2SO4, NaNO3, NaCl) did not has any influence in the equilibrium of TiBALDH.
According to these results, at equilibrium, both titanium lactate complexes and TiO2 nanoparticles are present in TiBALDH solutions, and they are dependent on the concentration and pH. We tried to crystalize TiBALDH to perform a characterization by X-ray diffraction, but it was impossible because the reason mentioned above. For that reason, we characterize the TiO2 nanoparticles using diluted TiBALDH solutions (50 mM) and transmission electron microscopy (TEM). For the solutions of TiBALDH studied here, only in the acid one presents nanoparticles of anatase (Fig. 4). The particles observed in the solutions with AB, CB, and water (pH 4.0) had a size of ∼5 nm, which corresponds to the one obtained by DLS. Although there is a chemical equilibrium between TiO2 particles and lactate–titanium complexes, the last ones were not identified by TEM, because sample is heated under electron beam burning it (at least under mild conditions).
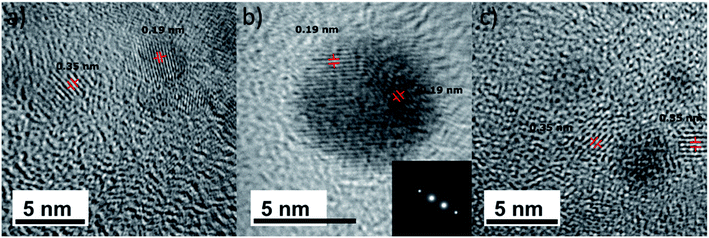 |
| Fig. 4 TEM images of the TiO2 particles found in samples of TiBALDH 50 mM in (a) acetate buffer, (b) citrate buffer, and (c) water (pH 4). | |
Phosphate influence
Although the phosphate ions are able to react with TiBALDH being the most common system used for biomineralization reactions, their influence is a tricky issue. In some reports, it was mentioned that phosphate ions promote TiBALDH precipitation,33,40,41 while in others no changes were reported using only PB and the mineralizing activity was attributed exclusively to the biomolecule employed.27,42,43 To establish the conditions where the PB has not effect TiBALDH stability we monitored the change in the particle size of solutions to different concentrations of TiBALDH and PB. From Fig. 5 it is clear that at low TiBALDH concentration the size is proportional to PB concentration, whereas at high TiBALDH concentration PB has null effect on the size. This effect is most important between 0.10–0.15 M of TiBALDH and depends on PB concentration, very probably the rapid reaction of Ti species with PO43− forms aggregates amorphous of phosphate titanium oxides.33,45 As the PB concentration decreases and the TiBALDH concentration increases, the size particle diminished until recovered its original size when TiBALDH concentration is 1.0 M, due to there are numerous species able to form hydrogen bonding that hampered the reaction with PO43− (low availability of free Ti(IV)). This result demonstrates that PB is not adequate to use as a buffer system in biomineralization reactions with TiBALDH because of its high reactivity.
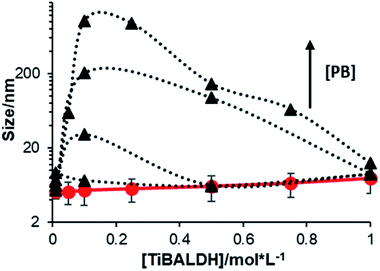 |
| Fig. 5 Particle size in the solution of TiBALDH in water (circles) and in phosphate buffer (triangles): 0.01 M, 0.02 M, 0.05 M, and 0.1 M. | |
Another source of phosphates was employed to react with TiBALDH to probe that any phosphate group is able to mineralize TiBALDH to TiO2. We proposed the circular dsDNA (M13mp18, 7249 base pairs) as a phosphate source because it is an easily replicative DNA in the laboratory, and it has a circular form that can be useful to guide nanostructures. In water and room temperature, the mixture of TiBALDH with DNA results in formation of TiO2 crystalline structures, which grows around DNA, resembling the shape of supercoiling DNA visibly wider than DNA helix, ∼100 nm vs. 2 nm, due to the formation of TiO2 crystallite around it (Fig. 6). The particles in diluted TiBALDH of 5.2 nm disappeared after the mixture with DNA, indicating that all TiO2 particles condensed in a hybrid structure DNA-TiO2. Nithiyanantham et al.46 reported the synthesis of similar structures using DNA and Ti alkoxide in a mixture of water/ethanol. Ti alkoxides are very reactive in that conditions forming hydroxy(oxo)titanium aggregates, however in presence of DNA and further annealing result in TiO2 wire-like structures, showing the scaffolding role of the DNA. However, our results show that phosphate groups biomineralize crystalline TiO2 and the DNA backbone acts as a scaffold (ESI S2†).
The reactivity of TiBALDH in the presence of phosphate groups can be useful to bioencapsulation as has been suggested by Kessler.47 Proteins or even macro-arrangements of proteins as viruses can be phosphorylated and then covered with TiO2 using TiBALDH, all at room temperature and aqueous media, which would ensure the molecular structure is preserved.
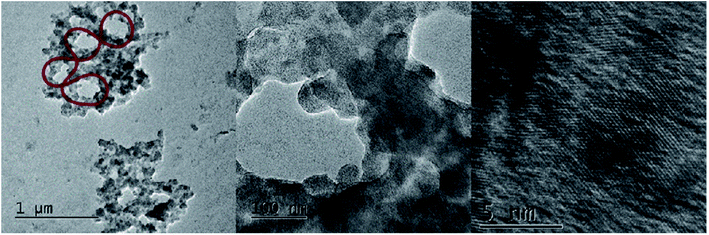 |
| Fig. 6 Nanostructures of TiO2 obtained by the reaction of TiBALDH and circular dsDNA (a representation of supercoiled circular DNA is superposed in red). | |
pH influence
Finally, the stability of TiBALDH versus pH was studied using DLS for monitoring any size particle changes. At acid value pH, the particle size remains the same as that at neutral pH (5.2 ± 1.7 nm), but the chemical equilibrium changes since the soluble Ti(IV) increases. If the pH corresponds to basic values, size particles increase up to form precipitates or a gel. In Fig. 7 it can be seen a region where the gel is formed (squares), the pH to form a gel depends on the TiBALDH concentration, in concordance with the decrease of reactivity in high concentrated TiBALDH as seen for PB. When the concentration of TiBALDH increase, a large amount of NaOH was necessary to reach the alkaline pH, and the ammonia odor is remarked; this is because the sodium hydroxide neutralizes ammonium ions and ammonia is released promoting the condensation of titanium complexes, as well as the autoesterification between lactate groups.48 The gel is similar to guar fluids formed in fracking processes, possibly because lactate ligands act as cross-linking agents.49 The process to form the gel also depends on the time; after 8 hours, the gelatinous solution is formed. This observation is in agreement with a recent kinetic study of TiBALDH, where the equilibrium also is reached several hours after the alkalization of the medium.31 The white gel was dried at 80 °C for 24 h to characterize the white particles formed under basic ambient. The solid showed anatase patterns as can be seen by X-ray diffraction (ESI S3†), confirming the identification of anatase nanoparticles in the solution equilibrium. Thus, the anatase phase is present in TiBALDH solutions, but the characteristics of these particles depend on the medium.
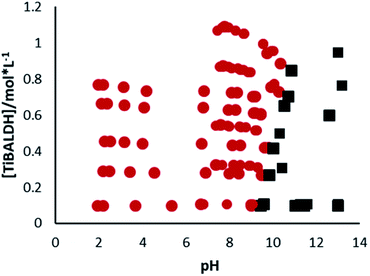 |
| Fig. 7 Aqueous phase diagram of TiBALDH vs. pH. Circles indicate each experiment resulting in a solution, and squares indicate experiments resulting in a gel. | |
The results presented in this work contribute to elucidate the chemical equilibrium of TiBALDH, which seems to be a multiple-step process. These complex species must be considered to find easier routes for the TiO2 synthesis at room temperature. Furthermore, the chirality of the lactate ligands can be useful to direct synthesis mechanisms and biocompatibility. In the fracking industry can be used to minimize the waste of hydraulic fluids. Even more, this titanium reagent can be used as a precursor for the synthesis of black titanium dioxide because it has an organic source, and also for encapsulation of biomolecules because it is stable in some aqueous buffered systems and the anatase phase is formed at room temperature.
Conclusions
TiBALDH solutions have a chemical equilibrium between titanium lactate complexes and anatase nanoparticles. This equilibrium is directly affected by TiBALDH concentration and pH, suggesting that polymerization occurs. The time to reach the equilibrium after dilution of TiBALDH is around 10 hours, which implies that the chemical compositions of fresh solutions of TiBALDH can vary along time. According to the results, TiBALDH concentrations ≤ 100 mM and pH < 8 should be used to ensure the lowest variation in the chemical equilibrium. Phosphate ions react with TiBALDH even at concentrations of 10 mM, and this buffer system must be avoided for biomineralization experiments. The presence of crystalline TiO2 opens the possibility to use TiBALDH solutions in photocatalysis just diluting in the adequate buffer system or for the synthesis of hybrid structures with phosphate-containing biomolecules.
Conflicts of interest
There are no conflicts to declare.
Acknowledgements
This work was partially supported by a grant from CONACYT, México (CB-2014-01-237256). A. H.-G. gratefully acknowledge the support from Consejo Nacional de Ciencia y Tecnología (CONACyT) for the scholarship granted in pursuit of his doctoral studies (265459). We thank Laboratorio Central de Microscopía Electrónica (UAM-I) for TEM images, Laboratorio de Difracción de Rayos X (T-128) UAM-I for XRD measurements and Laboratorio Divisional de Espectrometría de Masas (UAM-I) for the mass spectra.
References
- M. Sarikaya, Biomimetics: Materials fabrication through biology, Proc. Natl. Acad. Sci. U. S. A., 1999, 96(25), 14183 CrossRef CAS.
- I. A. Banerjee, Cu nanocrystal growth on peptide nanotubes by biomineralization: size control of Cu nanocrystals by tuning peptide conformation, Proc. Natl. Acad. Sci. U. S. A., 2003, 100(25), 14678 CrossRef CAS.
- C.-Y. Chiu, Y. Li and Y. Huang, Size-controlled synthesis of Pd nanocrystals using a specific multifunctional peptide, Nanoscale, 2010, 2(6), 927 RSC.
- R. R. Naik, S. J. Stringer, G. Agarwal, S. E. Jones and M. O. Stone, Biomimetic synthesis and patterning of silver nanoparticles, Nat. Mater., 2002, 1(3), 169 CrossRef CAS.
- R. Djalali, Y.-f. Chen and H. Matsui, Au Nanowire Fabrication from Sequenced Histidine-Rich Peptide, J. Am. Chem. Soc., 2002, 124(46), 13660 CrossRef CAS.
- S.-Y. Lee, X. Gao and H. Matsui, Biomimetic and Aggregation-Driven Crystallization Route for Room-Temperature Material Synthesis: Growth of β-Ga2O3Nanoparticles on Peptide Assemblies as Nanoreactors, J. Am. Chem. Soc., 2007, 129(10), 2954 CrossRef CAS.
- L. Yang, Q. Shen, J. Zhou and K. Jiang, Biomimetic synthesis of CdS nanocrystals in aqueous solution of pepsin, Mater. Chem. Phys., 2006, 98(1), 125 CrossRef CAS.
- C.-L. Chen and N. L. Rosi, Peptide-Based Methods for the Preparation of Nanostructured Inorganic Materials, Angew. Chem., Int. Ed., 2010, 49(11), 1924 CrossRef CAS.
- A. Kuzyk, R. Schreiber, Z. Y. Fan, G. Pardatscher, E. M. Roller, A. Hogele, F. C. Simmel, A. O. Govorov and T. Liedl, DNA-based self-assembly of chiral plasmonic nanostructures with tailored optical response, Nature, 2012, 483(7389), 311 CrossRef CAS.
- W. Zhang, D. Zhang, T. X. Fan, J. Ding, J. J. Gu, Q. X. Guo and H. Ogawa, Biomimetic zinc oxide replica with structural color using butterfly (Ideopsis similis) wings as templates, Bioinspiration Biomimetics, 2006, 1(3), 89 CrossRef CAS.
- Z. Liu, J. Qiao, Z. Niu and Q. Wang, Natural supramolecular building blocks: from virus coat proteins to viral nanoparticles, Chem. Soc. Rev., 2012, 41(18), 6178 RSC.
- A. Hernández-Gordillo, A. Campero and L. I. Vera-Robles, Mesoporous TiO2 synthesis using a semi-hard biological template, Microporous Mesoporous Mater., 2018, 270, 140 CrossRef.
- V. Puddu, H. Choi, D. D. Dionysiou and G. L. Puma, TiO2 photocatalyst for indoor air remediation: influence of crystallinity, crystal phase, and UV radiation intensity on trichloroethylene degradation, Appl. Catal., B, 2010, 94(3–4), 211 CrossRef CAS.
- C. Liu, T. Li, Y. Zhang, T. Kong, T. Zhuang, Y. Cui, M. Fang, W. Zhu, Z. Wu and C. Li, Silver nanoparticle modified TiO2 nanotubes with enhanced the efficiency of dye-sensitized solar cells, Microporous Mesoporous Mater., 2019, 287, 228 CrossRef CAS.
- Y. Qian, J. Du and D. J. Kang, Enhanced electrochemical performance of porous Co-doped TiO2 nanomaterials prepared by a solvothermal method, Microporous Mesoporous Mater., 2019, 273, 148 CrossRef CAS.
- M. B. Dickerson, K. H. Sandhage and R. R. Naik, Protein- and Peptide-Directed Syntheses of Inorganic Materials, Chem. Rev., 2008, 108(11), 4935 CrossRef CAS.
- S. Ahn, S. Park and S.-Y. Lee, Oligo(L-lysine)-induced titanium dioxide: effects of consecutive lysine on precipitation, J. Cryst. Growth, 2011, 335(1), 100 CrossRef CAS.
- Y. Jiang, D. Yang, L. Zhang, L. Li, Q. Sun, Y. Zhang, J. Li and Z. Jiang, Biomimetic synthesis of titania nanoparticles induced by protamine, Dalton Trans., 2008, 31(31), 4165 RSC.
- K. E. Cole and A. M. Valentine, Spermidine and Spermine Catalyze the Formation of Nanostructured Titanium Oxide, Biomacromolecules, 2007, 8(5), 1641 CrossRef CAS.
- S. Park, H. Lee and S.-Y. Lee, Effect of peptide conformation on TiO2 biomineralization, Dalton Trans., 2013, 42(38), 13817–13820 RSC.
- P. Cheng, J. Lin, X. Qiu, W. Zhang, J. Cheng, Y. Wang, N. Li, J. Yang and H. Yu, Viral capsid-like titania for selective enrichment of phosphorylated peptides, Chem. Commun., 2019, 55(47), 6759 RSC.
- A. Hernández-Gordillo, A. Hernández-Arana, A. Campero and L. I. Vera-Robles, Biomimetic Sol–Gel Synthesis of TiO2 and SiO2 Nanostructures, Langmuir, 2014, 30(14), 4084 CrossRef.
- S. L. Sewell and D. W. Wright, Biomimetic Synthesis of Titanium Dioxide Utilizing the R5 Peptide Derived from Cylindrotheca fusiformis, Chem. Mater., 2006, 18, 3108 CrossRef CAS.
- J. L. Sumerel, W. Yang, D. Kisailus, J. C. Weaver, J. H. Choi and D. E. Morse, Biocatalytically Templated Synthesis of Titanium Dioxide, Chem. Mater., 2003, 15(25), 4804 CrossRef CAS.
- N. Kröger, M. B. Dickerson, G. Ahmad, Y. Cai, M. S. Haluska, K. H. Sandhage, N. Poulsen and V. C. Sheppard, Bioenabled Synthesis of Rutile (TiO2) at Ambient Temperature and Neutral pH, Angew. Chem., Int. Ed., 2006, 45(43), 7239 CrossRef.
- C.-X. Zhao, L. Yu and A. P. J. Middelberg, Design of low-charge peptide sequences for high-yield formation of titania nanoparticles, RSC Adv., 2012, 2(4), 1292 RSC.
- M. B. Dickerson, S. E. Jones, G. A. Ye Cai, R. R. Naik, N. Kröger and K. H. Sandhage, Identification and Design of Peptides for the Rapid, High-Yield Formation of Nanoparticulate TiO2 from Aqueous Solutions at Room Temperature, Chem. Mater., 2008, 20, 1578 CrossRef CAS.
- Y. Yan, B. Hao, X. Wang and G. Chen, Bio-inspired synthesis of titania with polyamine induced morphology and phase transformation at room-temperature: Insight into the role of the protonated amino group, Dalton Trans., 2013, 42(34), 12179–12184 RSC.
- M. Kakihana, K. Tomita, V. Petrykin, M. Tada, S. Sasaki and Y. Nakamura, Chelating of titanium by lactic acid in the water-soluble diammonium tris(2-hydroxypropionato)titanate(IV), Inorg. Chem., 2004, 43(15), 4546 CrossRef CAS.
- G. A. Seisenbaeva, G. Daniel, J.-M. Nedelec and V. G. Kessler, Solution equilibrium behind the room-temperature synthesis of nanocrystalline titanium dioxide, Nanoscale, 2013, 5(8), 3330 RSC.
- A. Forgács, K. Moldován, P. Herman, E. Baranyai, I. Fábián, G. Lente and J. Kalmár, Kinetic Model for Hydrolytic Nucleation and Growth of TiO2 Nanoparticles, J. Phys. Chem. C, 2018, 122(33), 19161 CrossRef.
- A. V. Moharir, V. A. K. Sarma and G. S. R. Krishna Murti, Spectrophotometric determination of titanium with Tiron, Microchem. J., 1972, 17(2), 167 CrossRef CAS.
- V. Puddu, J. M. Slocik, R. R. Naik and C. C. Perry, Titania Binding Peptides as Templates in the Biomimetic Synthesis of Stable Titania Nanosols: Insight into the Role of Buffers in Peptide-Mediated Mineralization, Langmuir, 2013, 29(30), 9464 CrossRef CAS.
- B. Chakraborty and I. A. Weinstock, Water-soluble titanium-oxides: Complexes, clusters and nanocrystals, Coord. Chem. Rev., 2019, 382, 85 CrossRef CAS.
- V. G. Kessler, G. I. Spijksma, G. A. Seisenbaeva, S. Håkansson, D. H. A. Blank and H. J. M. Bouwmeester, New insight in the role of modifying ligands in the sol-gel processing of metal alkoxide precursors: A possibility to approach new classes of materials, J. Sol-Gel Sci. Technol., 2006, 40(2–3), 163 CrossRef CAS.
- V. I. Irzhak, M. E. Solov’ev and T. F. Irzhak, Architecture of Polymers: Topological Structure–Properties Relationship, Rev. J. Chem., 2018, 8(1), 76 CrossRef CAS.
- A. Hardy, J. D'Haen, M. K. Van Bael and J. Mullens, An aqueous solution–gel citratoperoxo–Ti(IV) precursor: synthesis, gelation, thermo-oxidative decomposition and oxide crystallization, J. Sol-Gel Sci. Technol., 2007, 44(1), 65 CrossRef CAS.
- M. Kakihana, K. Tomita, V. Petrykin, M. Tada, S. Sasaki and Y. Nakamura, Chelating of Titanium by Lactic Acid in the Water-Soluble Diammonium Tris(2-hydroxypropionato)Titanate(IV), Inorg. Chem., 2004, 43(15), 4546 CrossRef CAS.
- P. A. Demkowicz, PhD thesis, University of Florida, 2001.
- K. E. Cole, A. N. Ortiz, M. A. Schoonen and A. M. Valentine, Peptide- and Long-Chain Polyamine- Induced Synthesis of Micro- and Nanostructured Titanium Phosphate and Protein Encapsulation, Chem. Mater., 2006, 18(19), 4592 CrossRef CAS.
- R. E. Stote, S. F. Filocamo and J. S. Lum, Silaffin primary structure and its effects on the precipitation morphology of titanium dioxide, J. Mater. Res., 2016, 31(10), 1373 CrossRef CAS.
- S. L. Sewell and D. W. Wright, Biomimetic Synthesis of Titanium Dioxide Utilizing the R5 Peptide Derived from Cylindrothecafusiformis, Chem. Mater., 2006, 18(13), 3108 CrossRef CAS.
- N. Choi, L. Tan, J. R. Jang, Y. M. Um, P. J. Yoo and W. S. Choe, The interplay of peptide sequence and local structure in TiO2 biomineralization, J. Inorg. Biochem., 2012, 115, 20 CrossRef CAS.
- V. G. Kessler, G. A. Seisenbaeva, M. Unell and S. Håkansson, Chemically Triggered Biodelivery Using Metal-Organic Sol-Gel Synthesis, Angew. Chem., Int. Ed., 2008, 47(44), 8506 CrossRef CAS.
- N. Choi, L. Tan, J.-r. Jang, Y. M. Um, P. J. Yoo and W.-S. Choe, The interplay of peptide sequence and local structure in TiO2 biomineralization, J. Inorg. Biochem., 2012, 115, 20 CrossRef CAS.
- U. Nithiyanantham, A. Ramadoss, S. R. Ede and S. Kundu, DNA mediated wire-like clusters of self-assembled TiO2 nanomaterials: supercapacitor and dye sensitized solar cell applications, Nanoscale, 2014, 6(14), 8010–8023 RSC.
- N. G. M. Palmqvist, S. Bejai, J. Meijer, G. A. Seisenbaeva and V. G. Kessler, Nano titania aided clustering and adhesion of beneficial bacteria to plant roots to enhance crop growth and stress management, Sci. Rep., 2015, 5, 10146 CrossRef CAS PubMed.
- C. N. R. Rao, S. Natarajan and R. Vaidhyanathan, Metal Carboxylates with Open Architectures, Angew. Chem., Int. Ed., 2004, 43(12), 1466 CrossRef CAS.
- R. Barati and J.-T. Liang, A review of fracturing fluid systems used for hydraulic fracturing of oil and gas wells, J. Appl. Polym. Sci., 2014, 131(16), 40735 CrossRef.
Footnote |
† Electronic supplementary information (ESI) available. See DOI: 10.1039/c9ra05923g |
|
This journal is © The Royal Society of Chemistry 2019 |
Click here to see how this site uses Cookies. View our privacy policy here.