DOI:
10.1039/C9RA05904K
(Paper)
RSC Adv., 2019,
9, 38454-38463
Experimental and theoretical study of CO2 adsorption by activated clay using statistical physics modeling
Received
31st July 2019
, Accepted 19th October 2019
First published on 25th November 2019
Abstract
The objective of this paper was to study CO2 adsorption on activated clay in the framework of geological storage. The activation of clay was characterized via scanning electron microscopy, N2 adsorption–desorption isotherms, and X-ray diffraction. The adsorption isotherms were generated at different temperatures, namely, 298 K, 323 K, and 353 K. Based on the experimental result, a new model was simulated and interpreted using a multi-layer model with two interaction energies. The physicochemical parameters that described the CO2 adsorption process were determined by physical statistical formalism. The characteristic parameters of the CO2 adsorption isotherm such as the number of carbon dioxide molecules per site (n), the receptor site densities (NM), and the energetic parameters were investigated. In addition, the thermodynamic functions that governed the adsorption process such as the internal energy, entropy, and Gibbs free energy were determined by a statistical physics model. Thus, the results showed that CO2 adsorption on activated clay was spontaneous and exothermic in nature.
1 Introduction
Carbon dioxide is considered a major greenhouse gas and the cause of climate change.1 The IPCC estimates that CO2 concentration levels may increase to 570 ppm by the year 2100, causing an increase in the global average temperature of about 1.9 °C.2 One of the solutions to reduce carbon dioxide emissions from fixed-point sources is the development of CO2 capture and storage technologies. In order to sequestrate CO2 from fuel gases, various techniques have been studied such as chemical adsorption,3 absorption4 and use of membranes.5 Solid adsorption is the most promising for CO2 capture because of its low energy requirements, cost effectiveness, and ease of use at a variety of pressures and temperatures.6 Many different adsorbents can be used for this purpose such as clay, activated carbon zeolites, mesoporous silica, and organo-metallic structures. In this context, clay is considered a natural material for CO2 adsorption. CO2 adsorption with bentonitic clay minerals from Patagonia was studied by Venaruzzo et al. (2002).7 Equilibrium adsorption of the gas was measured at 25 °C and characterized by chemical analysis, X-ray diffraction, and N2 adsorption–desorption isotherms. This study indicated that the increase in the gas adsorption with bentonitic clay minerals was attributed to textural and structural properties. Chen et al. (2015)8 discussed the mechanism of CO2 adsorption by kaolinite via XRD, FTIR spectroscopy, and CO2 adsorption isotherm measurements. The CO2 adsorption performance of the sample was assessed on the basis of the changes in its weight. Also, the CO2 adsorption isotherm was measured using an ASAP 2020c (Micromeritics) static volumetric apparatus. Lyu et al. (2018)9 investigated the effect of CO2 adsorption on the mechanical properties of clay shale in several high-pressure containers. This study provided actual experimental data on the adsorption properties of shale with carbon dioxide. Jedli et al. (2016)10 compared the effects of CO2 adsorption on various samples such as clay, evaporates, and sandstone. The adsorbents were reacted on a batch reactor and characterized thoroughly via X-ray diffraction (XRD), scanning electron microscopy (SEM), Fourier transform infrared spectroscopy (FTIR), and nitrogen adsorption. The adsorption isotherms were measured at different temperatures and modeled with the Langmuir model. The main aim of this study, therefore, was to investigate the adsorption process of carbon dioxide on a solid surface, especially activated clay. In this context, an experiment was performed to investigate the CO2 adsorption process on activated clay. In fact, the adsorbent was characterized thoroughly via XRD (X-ray diffraction), SEM (scanning electron microscopy), and nitrogen adsorption desorption. The experimental data was modeled with statistical physics processing at 25, 50, and 75 °C. The fitting results were used to discuss the thermodynamics parameters for each adsorption isotherm type. Moreover, a detailed study of the main intrinsic features of the adsorbent was performed. Additionally, a detailed study of the physicochemical parameters of the adsorbent was established.
2 Experimental details
2.1 Materials and methods
The experimental device is presented in Fig. 1. It was essentially composed of a batch reactor with a total volume of 232 cm3, a manometer, electric heating, and a CO2 supply bottle (VMS-A S40). The raw clay was collected from a real site located in southern Tunisia and treated with H2SO4 solution (3 M).11 Characterization techniques were used to describe the sample at different observation scales. X-ray diffraction was performed on a “Philips MPD1880-PW1710’’ diffractometer with λCuKα radiation in the 2–80° interval with a step size of 0.02° and a counting time of 20 s per step. The quantification phase was performed on one sample by the Rietveld method (R-QPA) using a PANalytical X'Pert High-Score Plus Program. The surface morphologies of the adsorbent were observed by a Thermo Fisher FEI Q250 scanning electron microscope. The porous textural characterizations were determined using an ASAP 2020 micromeritics analyzer. The specific BET surface area and pore size distribution were obtained using the adsorption/desorption isotherms of nitrogen vapor at 77 K.
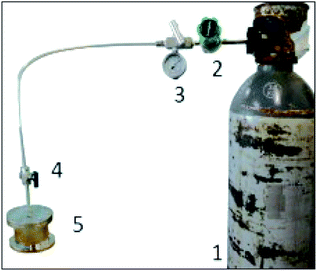 |
| Fig. 1 Schematic of the experimental study (1) gas cylinder, (2) pressure regulator, (3) pressure indicator, (4) needle valves, and (5) batch reactor. | |
2.2 Characterization of adsorbent
Table 1 shows the chemical composition of the activated clay determined by X-ray fluorescence (XRF). The XRF indicated the presence of silica (SiO2), aluminum (Al2O3), and iron oxide (Fe2O3) as main constituents, as well as traces of other oxides such as magnesium oxide (MgO), calcium oxide (CaO), and potassium oxide (K2O). From the XRD diffractograms of activated clay (Fig. 2), the characteristic reflections located at 4.45 Å and 3.33 Å were assigned to illite, whereas kaolinite was found at 7.51, 3.78, 2.56, and 2.48 Å. The characteristic reflections of dolomite were observed at 2.88 Å and those of quartz at 3.33 and 1.87 Å. The N2 adsorption–desorption isotherms of activated clay corresponded to a typical type IV isotherm, characteristic of a mesoporous material (Fig. 3). The hysteresis loop for the sample was similar to type H3, which was typical of agglomerates with plate-like particles containing slit-shaped pores. The textural properties, including the BET surface areas, pore volumes, and pore sizes of activated treated clay are presented in Table 2. The surface area and pore volume of the clay sample were 24.68 cm2 g−1 and 0.064 cm2 g−1, respectively, indicating that activated clay exhibited a low porosity. The scanning electron micrographs of the sample are provided in Fig. 4. The SEM showed a different particle morphology. In fact, the adsorbent presented a stratified form containing stacked flakes in the form of agglomerates, which displayed irregular and angular edges. The adsorption isotherms on activated clay at 25, 50, and 75 °C are depicted in Fig. 5. As expected, these isotherms were expressed as a function of the amount adsorbed and the pressure. The adsorption capacity increased with the increase in the pressure. In fact, an increase in the adsorption temperature led to a reduction in the amount of carbon dioxide adsorbent.
Table 1 Chemical analysis of activated clay
Element, wt% |
SiO2 |
Al2O3 |
CaO |
Fe2O3 |
Na2O |
K2O |
MgO |
TiO2 |
I.Lo |
Activated clay |
69.87 |
8.23 |
6.72 |
1.84 |
0.14 |
2.48 |
4.02 |
0.10 |
7.78 |
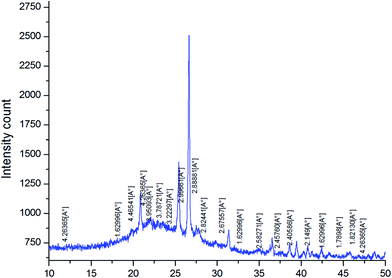 |
| Fig. 2 XRD of activated clay. | |
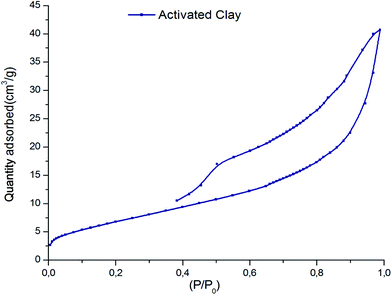 |
| Fig. 3 N2 adsorption–desorption isotherm of activated clay. | |
Table 2 Textural properties for activated clay
Concentration of H2So4 (M) |
BET surface area (m2 g−1) |
Pore volume (cm3 g−1) |
Pore size (Å) |
Activated clay |
24.68 |
0.064 |
65.71 |
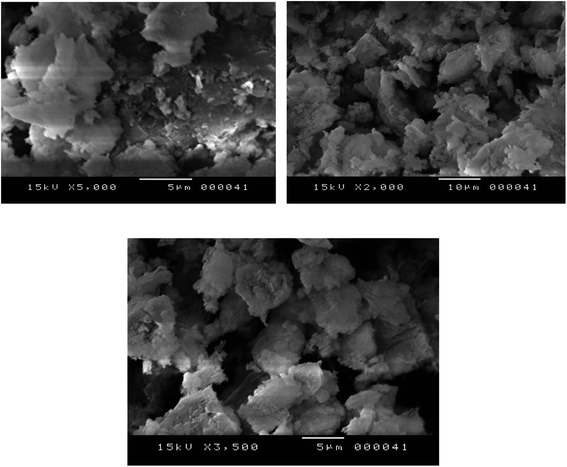 |
| Fig. 4 SEM morphologies of the activated clay. | |
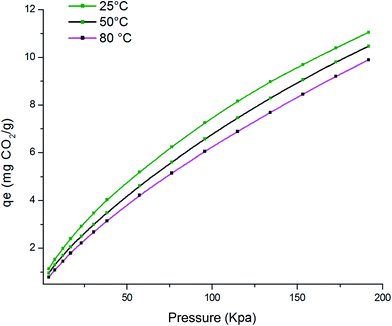 |
| Fig. 5 CO2 adsorption isotherms on activated clay. | |
3 Modeling by statistical physics of CO2 adsorption isotherms
3.1 Adsorption modeling
The analytical expression used for the adsorption isotherms was based on a statistical physics approach. The statistical physics treatment was used for the gas physical states of the adsorbate. Furthermore, the interaction between the adsorbed molecules was neglected.12 The physical interpretations of the adsorption phenomenon were provided according to the experimental study. For the state of adsorption, the equation used was:13where n, S and AnS are respectively the receptor sites, the number of molecules per site and the formed adsorbate–adsorbent complex. In the present study, we used a grand canonical partition function for the interstitial site. This function described each adsorption process for the microscopic states. The receptor site could be empty or occupied by one or more molecules. The grand canonical partition for one receptor site is expressed in the following equation:14 |
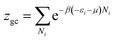 | (2) |
where (−εi) is the receptor site sorption energy, Ni is the receptor site occupation state, μ is the chemical potential, and β is defined as 1/kBT. T is the absolute temperature and kB is the Boltzmann constant. The relation between the total grand canonical partition function, Zgc, and the NM receptor sites is given by eqn (3):
The average number of sites can be described as follows:15
|
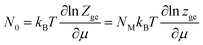 | (4) |
The average number of the adsorbed molecules is written as follows:16
|
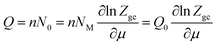 | (5) |
To develop the multilayer model during the adsorption process, we considered that the mutual interactions between the adsorbate molecules were neglected. Then, the internal degrees of freedom for the gas were neglected. However, the molecules in the first layer (N1M) were adsorbed with (−ε1) energy, whereas the molecules in the next (N2M) layers were adsorbed with (−ε2) energy, knowing that |ε1| > |ε2| > 0. Under these conditions, the global grand canonical partition function was written as:
|
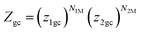 | (6) |
where
z1gc and
z2gc are respectively the partition function for site type 1 and 2. Their expressions are:
|
 | (6a) |
|
 | (6b) |
where (−
ε1) and (−
ε2) are the receptor site desorption energies, N
i is the receptor site occupation state,
μ is the chemical potential of the adsorbed site, and
β is expressed by 1/
kBT.
T is the temperature in K and
kB is the Boltzmann constant. During thermodynamic equilibrium, the equality of the different chemical potentials can be written as
μm =
μ/
n, where
μ is the chemical potential of the receptor site and
n is the number of atoms per site.
Using the first approximation for an ideal gas, μm can be expressed as follows:17
|
 | (7) |
where:
|
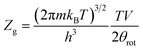 | (8) |
where
θrot,
m,
v, and
h are respectively the rotational characteristic temperature, the mass of the adsorbed gas, the volume of the gas, and Planck's constant.
Using eqn (5), the average number of occupied sites was expressed by the following equation:
|
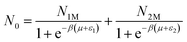 | (9) |
where
ε1m and
ε2m are the energies of an adsorbed gas atom on the two different sites, respectively. We can write:

and
Using eqn (7) and the equilibrium conditions related to the chemical potentials, the average number of occupied sites given in eqn (9) becomes:
|
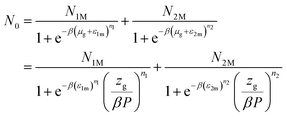 | (10) |
If we take P = kBTZgtr
eβεm, we obtain an average number of occupied sites at half N01 = N1M/2 and N02 = N2M/2. We then noted that P1 = kBTZgtr
e−βε1m and P2 = kBTZgtr
e−βε2m, which represented the pressure at half-saturation. This corresponded respectively to the first and the second type of sites. n1 and n2 are the number of atoms per site. The average number of occupied sites is written as follows:
|
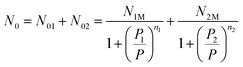 | (11) |
Using eqn (1) and the number of average occupation site, N0, the average number of remaining adsorbed molecules is:
The expression for the number of remaining adsorbed gas molecules as a function of pressure is written as follows:
|
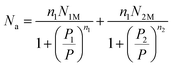 | (13) |
The experimental adsorbed quantity, Qs, indicates the number of CO2 atoms per unit formula. In our model, there were two types of sites that adsorbed two different quantities, n1 and n2, on N1M and N2M. In fact, we took the average between n1 and n2 weighted by N1M and N2M.
where
|
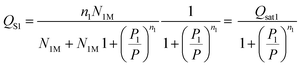 | (15) |
|
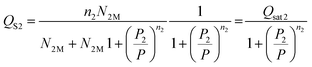 | (16) |
The analytical model expression that fitted the experimental isotherms was:
|
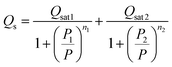 | (17) |
The final expression for the average number of adsorbed molecules (first model, namely, Model 1) can be expressed by the following equation:16
|
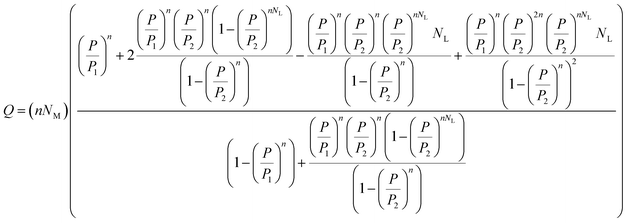 | (18) |
The final expression of model 1 contained physicochemical parameters such as n, NM, NL, P1, and P2. These parameters are defined as follows: the number of molecules per site, n, the density of the receptor sites, NM, the number of adsorbed layers, NL. From the experimental isotherm, we deduced the pressures at the half-saturation, P1 and P2.
3.2 Fitting results
The main objective of the modeling process was to select the appropriate model that better correlated with the isotherm experimental data. The CO2 adsorption isotherms on activated clay showed a saturation phenomenon at high pressures. The experimental data was used in the simulation process with different theoretical models based on a statistical physics treatment. To obtain the different parameters for the model, several iterations were used for all of experimental results. Classical and non-classical models were evaluated such as Langmuir, BET, Hill (1), and Hill (2).18,19 In addition to the first adsorption layer, the adsorption phenomenon was assumed to also increase a number of layers. Thus, the classical Hill model and the double layer Hill model, which respectively considered one energy (−ε1) (Model 2) and two energies (−ε1) and (−ε2) (Model 3)20 were tested. The most suitable model was determined according to the correlation coefficient, R2. Table 3 present the adjustment coefficient values for the different models. The fit of the experimental adsorption isotherms for activated clay at 298 K, 323 K, and 348 K showed that the model correlated well with the experimental data as illustrated in Fig. 6.
Table 3 Values for the R2 fitting coefficient
T (K) |
Monolayer with fixed parameters |
298 |
0.9964 |
323 |
0.9973 |
353 |
0.9980 |
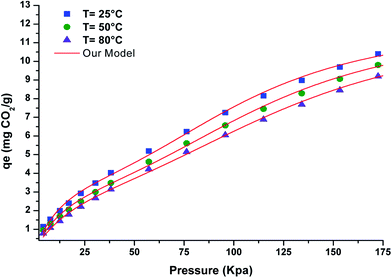 |
| Fig. 6 Experimental data for the CO2 adsorption isotherms on activated clay fitted with our model. | |
4. Results
4.1 Steric parameters
The monolayer model with two types of sites was suitable for analyzing the experimental adsorption isotherms. The model parameters gave characteristics on the number of desorbed atoms per site, the density of the receptor sites, and the desorption energies. These parameters were classified into two categories. The number of steric atoms by site was n1 and n2 and receptor site densities were NM1 and NM2. Energy parameters P1 and P2 gave the desorption energy, which characterized the bond between the atoms and receptor sites. The values for the adjusted parameters are provided in Table 4.
Table 4 Adjustment parameter values corresponding to the best fit model
Temperature (°C) |
NM1 |
NM2 |
P1 |
P2 |
n1 |
n2 |
25 |
6.23 |
10.77 |
134.17 |
53.52 |
2.55 |
0.81 |
50 |
1.73 |
8.48 |
130.30 |
49.55 |
3.16 |
0.916 |
80 |
1.58 |
6.74 |
134.01 |
45.42 |
3.35 |
1.02 |
4.1.1 n1 and n2 parameters. The parameters n1 and n2, which represented the number of atoms per site, were stoichiometric coefficients. These parameters were in accordance with the adsorption complexation expressed by eqn (1). The numbers (n1 and n2) were integers. However, the adjusted values for n1 and n2 were not integers because these yielded the average values for all of the receptor sites, which could have been a decimal number. Fig. 7 shows the variation in n1 and n2 during the adsorption process. We noted that the values for the number of CO2 atoms in the first and the second site were not identical during the adsorption phenomena. In addition, the number of molecules per site (n) was defined as the average yield for all of the receptor sites, which ranged from 1.68 to 2.18 for all of the site types. The variation in n was due to the effect of the temperature.
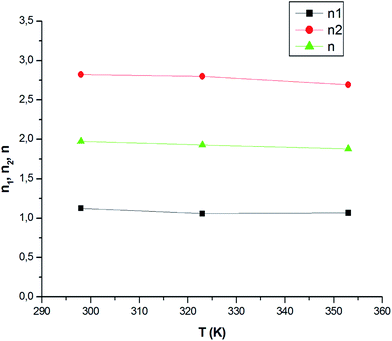 |
| Fig. 7 Variation in the number of atoms per site n1 and n2 versus the temperature. | |
4.1.2 NM1 and NM2 parameters. The parameters NM1 and NM2 presented two receptor sites, which were actually necessary for activation to separately adsorb the atoms. The evolution of the N1M and N2M adsorption was a function of the temperature as shown in Fig. 8. We saw that these parameters evolved in an opposite manner than the temperature. The temperature lowered the NM1 and NM2 densities. The thermal agitation effect prevents the occupation of the sites, and therefore, reflects the exothermic effect of adsorption for both sites.
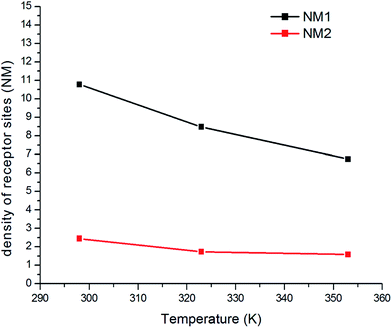 |
| Fig. 8 Variation in parameters NM1 and NM2 versus the temperature. | |
4.1.3 Saturation adsorption quantity Qsat parameters. The saturation adsorption quantity, Qsat, depended on the number of molecules per site (n) and the density of the NM receptor sites, which characterized the ability of the activated clay surface to capture the carbon dioxide molecules.16 We noted from Fig. 9 that an increase in the temperature led to a reduction in the adsorbed quantity during the first phase, Qsat1. The evolution of Qsat was consistent with the exothermic character of the CO2 adsorption process on the sample. In fact, we noted that the global adsorption was attributed to two separate contributions. Despite the difference in the site properties, the global filling was almost similar. Both sites belonged to the same unit form with a slightly larger padding requirement for the second type of site in the second phase. The maximum adsorbed Qsat increased with the increase in the temperature. The increase in n2 was already attributed to the endothermic nature of the aggregation of n2 CO2 atoms on the N2M density of the receptor site.
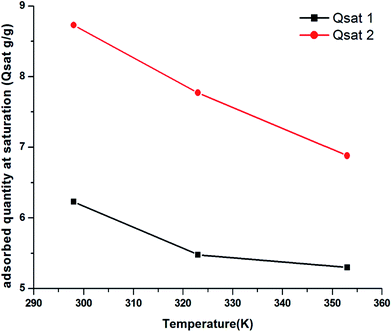 |
| Fig. 9 Variation in parameters Qsat1 and Qsat2 versus the temperature. | |
4.2 Energy parameters
The energy adsorption was an important parameter used to characterize the nature of the interaction between carbon dioxide and the sample. From the parameters P1, P2, and the vapor saturation pressure, the adsorption energy ΔE1a and ΔE2a for site 1 and 2 was expressed by the following equation:21 |
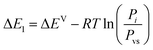 | (19) |
where i = 1 or 2 and R is the ideal gas constant, R = 8.314
472 J mol−1, and Pvs is the pressure of the saturated vapor. |
 | (21) |
Fig. 10 shows the variation in the adsorption energies versus three temperatures (298 K, 323 K, and 353 K) and Table 5 presents their value. ΔE1 and ΔE2 present the interaction between the molecules and the surface of the first and second layer, respectively. We saw that both adsorption energies increased with respect to the temperature. This was due to the effect of thermal agitation. The adsorption energy calculated at 298 K was 108.72 kJ mol−1, while the adsorption energy at 353 K was 164.50 kJ mol−1. This increase in energy was explained by the temperature effect, which agitated the atoms in the site and possibly facilitated the adsorbed atoms to be easily lodged in the volume of the site.
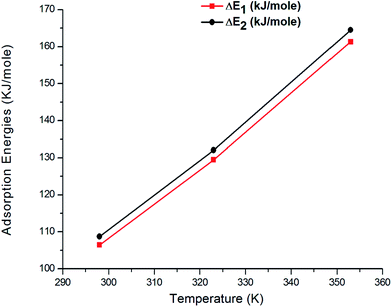 |
| Fig. 10 Variation in adsorption energies ΔE1 and ΔE2 versus the temperature. | |
Table 5 Values corresponding to the adsorption energies
T (K) |
ΔE1 (kJ mol−1) |
ΔE2 (kJ mol−1) |
298 |
106.45 |
108.72 |
323 |
129.47 |
132.60 |
353 |
161.33 |
164.50 |
4.3 Entropy
From the grand canonical partition function for the multilayer model with two energies, the entropy is expressed as follows:22 |
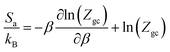 | (20) |
The adsorption entropy, Sa, was calculated using the following expression:
The entropy indicates the degree of disorder and randomness of CO2 molecules. Fig. 11 shows the evolution of entropy as a function of pressure at different temperatures. Indeed, at low pressures, the entropy increases; this is due to the availability of a large number of receptor sites on the surface of the adsorbent. Therefore, the CO2 molecules were connected to the empty sites. Then, at high pressures, we saw a reduction in the entropy configuration. This reduction was due to the decrease in the number of free sites. Consequently, at high pressures, CO2 had a low probability of selecting a receptor site since the adsorbent tended toward saturation, and therefore tended toward being ordered.
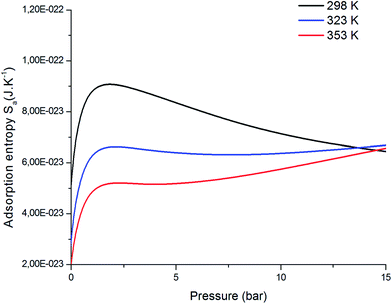 |
| Fig. 11 Evolution of the entropy, Sa, versus the pressure at different temperatures. | |
4.4 Internal energy
The internal energy is corresponding to the interactions between the adsorbate–adsorbent system. The internal energy is given by:23 |
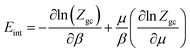 | (22) |
|
 | (23) |
Fig. 12 shows the internal energy variation of CO2 adsorption depicted at different temperatures. We can see that the internal energy values are negative for all temperatures. Moreover, an increase in the pressure was observed, which in turn shows a decrease in the enthalpy. In this study, the decreases in enthalpy present the amount of work available to introduce the atoms n1 and n2 in the receptor sites N1M and N2M. The adsorption process was exothermic in nature.
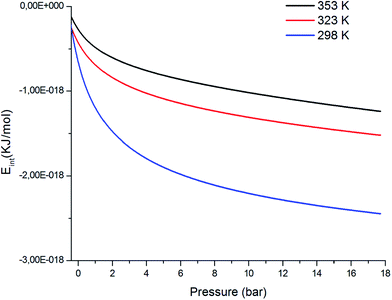 |
| Fig. 12 Evolution of the internal energy, Eint, versus the pressure at different temperatures. | |
4.5 Gibbs free energy
The Gibbs free energy, G, describes the spontaneity of the system, and is given as follows:24
Therefore, the expression for the Gibbs free energy is:
|
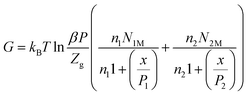 | (25) |
Fig. 13 shows the variation in the Gibbs free energy as a function of pressure for different temperatures. The Gibbs free energy, G, was negative, which reflected that the adsorption reaction was spontaneous. This thermodynamic function decreased with increasing temperature, which meant that the thermal agitation decreased the spontaneity of the adsorption process.
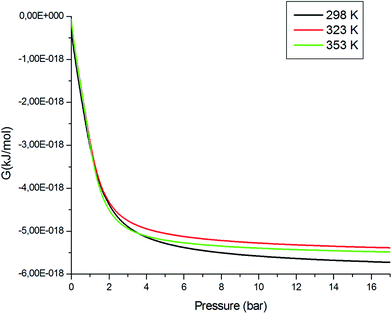 |
| Fig. 13 Evolution of the Gibbs free energy versus pressure at different temperatures. | |
4.6 Chemical potential
The chemical potential is written as follows:24 |
 | (26) |
Fig. 14 shows the evolution of the chemical potential versus the pressure. The chemical potential decreased with increasing temperature. Also, we noted that with an increasing temperature, the total number of adsorbed CO2 atoms decreased. On the other hand, if we augmented the pressure, the adsorption process progressed and the CO2 amount increased progressively in the adsorbent. Therefore, the chemical potential increased.
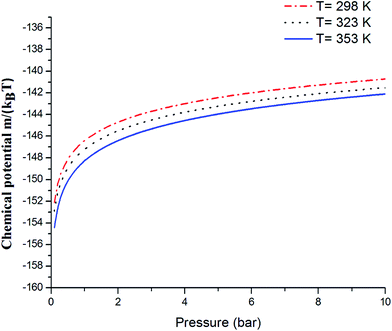 |
| Fig. 14 The variation in the chemical potential with pressure. | |
5. Conclusion
In this paper, the CO2 adsorption on activated clay was measured at different temperatures (298 K, 323 K, and 353 K) and characterized via SEM, XRD, and N2 adsorption–desorption. The adsorbent was simulated using a statistical physics model. The parameters for the carbon dioxide adsorption isotherms were theoretically interpreted using the multi-layer model with two energies. The main characteristic parameters, namely, the number of carbon dioxide molecules per site (n), the receptor site density (NM), and the energetic parameters (−ε1) and (−ε2), were interpreted at different temperatures. In fact, the thermodynamic functions, namely, the entropy, the Gibbs free energy, and the internal energy, were evaluated. The results showed that CO2 adsorption on activated clay was spontaneous and exothermic in nature.
Conflicts of interest
There are no conflicts to declare.
Acknowledgements
The authors would like to thank the USCR_Environmental for their Thermo Fisher FEI Q250 scanning electron microscope at the University of Monastir and for helpful technical assistance with equipment facilities.
References
- A. Wahby, J. Silvestre-Albero, A. Sepúlveda-Escribano and F. Rodríguez-Reinoso, CO2 adsorption on carbon molecular sieves, Microporous Mesoporous Mater., 2012, 164, 280–287 CrossRef CAS.
- I. E. A., Energy technology perspectives, http://www.iea.org/techno/etp/etp10/English.pdf, 2010 Search PubMed.
- H. Jedli, J. Brahmi, H. Hedfi, M. Mbarek, S. Bouzgarrou and K. Slimi, Adsorption kinetics and thermodynamics properties of Supercritical CO2 on activated clay, J. Pet. Sci. Eng., 2018, 166, 476–481 CrossRef CAS.
- P. R. Jeon, J. Choi, T. S. Yun and C.-H. Lee, Sorption equilibrium and kinetics of CO2 on clay minerals from subcritical to supercritical conditions: CO2 sequestration at nanoscale interfaces, Chem. Eng. J., 2014, 255, 705–715 CrossRef CAS.
- A. Guo, Y. Ban, K. Yang and W. Yanga, Metal–organic framework-based mixed matrix membranes: Synergetic effect of adsorption and diffusion for CO2/CH4 separation, J. Membr. Sci., 2018, 562, 76–84 CrossRef CAS.
- H. Jedli, A. Jbara, H. Hedfi, S. Bouzgarrou and K. Slimi, Carbon dioxide adsorption isotherm study on various cap rocks in a batchreactor for CO2 sequestration processes, Appl. Clay Sci., 2017, 136, 199–207 CrossRef CAS.
- J. L. Venaruzzo, C. Volzone, M. L. Rueda and J. Ortiga, Modified bentonitic clay minerals as adsorbents of CO, CO2 and SO2 gases, Microporous Mesoporous Mater., 2002, 56, 73–80 CrossRef CAS.
- Y.-H. Chen and De-L. Lu, CO2 capture by kaolinite and its adsorption mechanism, Appl. Clay Sci., 2015, 104, 221–228 CrossRef CAS.
- Q. Lyu, X. Long, P. G. Ranjith, J. Tan, Y. Kang and Z. Wang, Experimental investigation on the mechanical properties of a low-clay shale with different adsorption times in sub-/super-critical CO2, Energy, 2018, 15, 1288–1298 CrossRef.
- H. Jedli, Abdessalem Jbara. Hachem Hedfi. souhail Bouzgarrou. Khalifa Slimi. A laboratory study of supercritical CO2 adsorption on cap rocks in the geological storage conditions, Appl. Phys. A, 2017, 123, 254 CrossRef.
- H. Jedli, J. Brahmi, H. Hedfi, M. Mbarek, S. Bouzgarrou and K. Slimi, Adsorption kinetics and thermodynamics properties of Supercritical CO2 on activated clay, J. Pet. Sci. Eng., 2018, 166, 476–481 CrossRef CAS.
- A. Gannouni, M. Bagane and A. Bellagi, SO2 adsorption onto activated clay, Ann. Chimie Sci. Matériaux, 1994, 19, 27–37 CAS.
- S. Bouzgarrou, H. Jedli, N. Stiti, N. Hamdi, K. Slimi and M. Bagana, Experimental Adsorption and Modelisation of CO2 on adsorbents collected from elborma fieldin South Tunisia, J. Surf. Eng. Mater. Adv. Technol., 2015, 5, 52–63, DOI:10.4236/jsemat.2015.51006.
- B. Diu, C. Guthmann, D. Lederer and B. Roulet, Physique Statistique, Hermann, Paris, 1989 Search PubMed.
- C. Briki, M. Bouzid, M. Houcine Dhaou, A. Jemni and A. Ben Lamine, Experimental and theoretical study of hydrogen absorption by LaNi3.6Mn0.3Al0.4Co0.7 alloy using statistical physics modeling, Int. J. Hydrogen Energy, 2018, 43(20), 9722–9732 CrossRef CAS.
- F. Aouaini, S. Bouzgarrou, N. Khemiri, M. Ben Yahia, E. S. Almogait, F. F. AlHarbi, A. H. Almuqrin and A. Ben Lamine, Study of the CO2 adsorption isotherms on El Hicha clay by statistical physics treatment: microscopic and macroscopic investigation, Sep. Sci. Technol., 2019, 54(16), 1–12 CrossRef.
- B. Diu, C. Guthmann, D. Lederer and B. Roulet, Physique statistique, Hermann, Paris, 1989 Search PubMed.
- S. Brunauer, L. S. Deming and E. Teller, On a theory of the van der Waals adsorption of gases, J. Am. Chem. Soc., 1940, 62, 1723–1732 CrossRef CAS.
- S. Bouzgarrou, H. Jedli, N. Stiti, N. Hamdi, K. Slimi and B. Mohamed, Experimental Adsorption and Modelisation of CO2 on Adsorbents Collected from Elborma Field in South Tunisia, J. Surf. Eng. Mater. Adv. Technol., 2015, 5, 52–63 CAS.
- R. B. Anderson, Modifications of the Brunauer, Emmett and Teller equation, J. Am. Chem. Soc., 1946, 68(4), 686–691 CrossRef CAS.
- F. Aouaini, S. Knani, M. Ben Yahia and A. Ben Lamine, Statistical research of water vapor sorption isotherm in food materials: steric and energetic interpretations, Sens. Lett., 2015, 131, 1–10 Search PubMed.
- M. Hadi, M. R. Samarghandi and G. McKay, Equilibrium two-parameter isotherms of acid dyes sorption by activated carbons: study of residual errors, J. Chem. Eng. Data, 2010, 160, 408–416 CrossRef CAS.
- L. Couture and R. Zitoun, Physique statistique, Ellipses, 1992, p. 375 Search PubMed.
-
(a) I. Rubinstein and L. Rubinstein, Partial differential equation in classical mathematical physics, The Hebrew University of Jerusalem, 1998, p. 18e64 Search PubMed;
(b) N. Bouaziz, M. Bouzid and A. Ben Lamine, Theoretical study of hydrogen absorption and desorption in Ti1-xZrx Mn1.4 using statistical physics treatment: Microscopic investigation and thermodynamic potential interpretation, Int. J. Hydrogen Energy, 2018, 43(3), 1615–1633 CrossRef CAS.
|
This journal is © The Royal Society of Chemistry 2019 |