DOI:
10.1039/C9RA05880J
(Paper)
RSC Adv., 2019,
9, 32184-32196
The role of surface copper content on biofilm formation by drinking water bacteria†
Received
29th July 2019
, Accepted 3rd October 2019
First published on 9th October 2019
Abstract
Copper pipes are conventionally used to supply tap water. Their role in biofilm prevention remains to be understood. This study evaluates the ability of selected surface materials with different copper contents (0, 57, 79, 87, 96, 100% of copper) to control biofilm formation and regrowth. Further experiments were performed to assess copper leaching and corrosion under conditions mimicking real plumbing systems. Acinetobacter calcoaceticus and Stenotrophomonas maltophilia isolated from a drinking water distribution system were used as model bacteria. All the copper materials showed positive results on the control of single and dual species biofilms presenting high reductions of bacterial culturability > 4 log CFU per cm2. The antimicrobial action of the selected materials seem not to be related to copper leaching or to the formation of reactive oxygen species. However, bacterial-copper contact demonstrated damage to bacterial membranes. The alloy containing 96% copper was the most promising surface in reducing biofilm culturability and viability, and was the only surface able to avoid the regrowth of single species biofilms when in contact with high nutrient concentrations. The alloy with 87% copper was shown to be unsuitable for use in chlorinated systems due to the high copper leaching observed when exposed to free chlorine. The presence of viable but non-culturable bacteria was remarkable, particularly in dual species biofilms. The overall results provide novel data on the role of copper alloys for use under chlorinated and unchlorinated conditions. Copper alloys demonstrated comparable or even higher biofilm control effects than elemental copper surfaces.
Introduction
Drinking water distribution systems (DWDS) are far from being microbiologically safe.1 This is due to the ability of microorganisms to colonize DWDS and form biofilms. These biological structures provide the colonizing microorganisms with improved protection from environmental stress.2 Antimicrobial products, particularly chlorine-based disinfectants, are used in several countries for drinking water disinfection and biofilm control in DWDS.3 Although chlorination is widespread in drinking water disinfection, there are no standardized strategies with reliable efficacy in biofilm control.3 The formation of disinfection by-products (DBPs) in drinking water also constitutes a global concern due to inherent risks for human health and the environment.4,5 Therefore, there is a global concern on developing alternative strategies to control biofilms in DWDS, minimizing the environmental and public health impacts from current disinfection strategies. A pioneer study6 tested preventive strategies to identify materials that do not promote or can even suppress biofilm formation. Different materials were ranked according to their biofilm growth propensity, which unfortunately led to the conclusion that no material was found able to completely avoid biofilm formation.6 More recently, Zhang et al.7 found that the type of material can affect biofilm disinfection efficiency, demonstrating that chlorine decay rate was higher on stainless steel pipes than on ductile iron pipe. The lowest chlorine decay rate was obtained in polyethylene pipes. Moreover, some surfaces are recognized for their antimicrobial properties, particularly copper and silver.8,9
Copper has been used in drinking water systems worldwide and there are several works evaluating copper in terms of antimicrobial properties and biofilm formation in DWDS.8,10 The most consistent effect was the slower biofilm formation dynamics when copper was used as plumbing material.11–13 However, the ability to reduce biofilm formation was typically more evident in the first months of operation. After longer periods (90 to 200 d) the biofilms had similar characteristics to those formed on other materials, such as stainless steel and polyethylene.12,13 Lu et al.14 compared the effects of the upstream use of cooper with that of unplasticized polyvinyl chloride (uPVC) in biofilm formation and found decreased microbial diversity when copper was applied. van der Kooij et al.15 also found that copper was able to inhibit the recovery of Legionella pneumophila in plumbing systems until 2 years of operation. Oppositely, Gião et al.16 demonstrated that copper was ineffective in L. pneumophila control. Rhoads et al.17 explained the higher incidence of copper antimicrobial properties in the earlier periods of system operation – due to the pipe age and decrease in copper ion release. However, the same authors reinforced that the mechanisms promoting microbial survival on cooper plumbing pipes are not completely understood.
Even if copper has limited effects in biofilm prevention, copper antimicrobial properties are of potential interest for the control of established biofilms.18,19 Nevertheless, there are limitations on the application of copper in plumbing systems that must be overcome. The occurrence of corrosion and the consequent leaching of copper to the bulk water is potentially critical when using copper pipes.20 This phenomenon is responsible for structural damage (pinhole leaks), aesthetic problems (metallic taste and “blue water” phenomenon) and human health risks from released copper into the drinking water.21,22 Taking into account these adverse consequences, the levels of copper at the tap are regulated: maximum limit of 1.3 mg L−1 according to the US EPA lead and copper rule;23 2 mg L−1 recommended by the World Health Organization24 and by the EU Directive 98/83/EC.25 The interest in using plastic materials emerged as a response to these limitations and to the high cost of copper materials. Nevertheless, the use of plastics is controversial as they are associated to chemical leaching to the drinking water and changes in water odor.26 The possibility of using less expensive copper alloys in plumbing systems would certainly boost the interest for their use. However, research on the use of copper alloys in plumbing systems is scarce.22 The variability in the composition of copper alloys and consequently on the material characteristics does not facilitate the experimental design and the understanding of copper leaching phenomena.27 Most of the works published about the use of copper in plumbing systems used elemental copper surfaces14,16,28–30 and did not present new alternatives to improve biofilm prevention or to reduce possible leaching problems.
Taking into account the limited knowledge on the role of copper alloys in biofilm control, this study aims to understand the effects of selected alloys (0, 57, 76, 87, 96 and 100%) on biofilm formation and regrowth using conditions intended to simulate drinking water. Additionally, copper leaching and surface corrosion in the presence of residual chlorine and the formation of reactive oxygen species were evaluated.
Experimental
Bacteria and culture conditions
Acinetobacter calcoaceticus and Stenotrophomonas maltophilia, previously isolated from DW,31 were grown overnight at 25 °C and under agitation (120 rpm) in R2A broth medium according to Gomes et al.32 Afterwards, bacterial cells were harvested by centrifugation (Eppendorf centrifuge 5810R) at 3777 × g, 15 min, and resuspended in synthetic tap water (STW) composed by 100 mg L−1 NaHCO3 (Fisher Scientific, Leicestershire, UK), 13 mg L−1 MgSO4·7H2O (Merck, Darmstadt, Germany), 0.7 mg L−1 K2HPO4 (Applichem Panreac, Darmstadt, Germany), 0.3 mg L−1 KH2PO4 (CHEM-LAB, Zedelgem, Belgium), 0.01 mg L−1 (NH4)2SO4 (Labkem, Barcelona, Spain), 0.01 mg L−1 NaCl (Merck, Darmstadt, Germany), 0.001 mg L−1 FeSO4·7H2O (VWR PROLABO, Leuven, Belgium), 1 mg L−1 NaNO3 (Labkem, Barcelona, Spain), 27 mg L−1 CaSO4 (Labkem, Barcelona, Spain), 1 mg L−1 humic acids (Sigma-Aldrich, Steinheim, Germany).33,34 The cell density was adjusted to 1 × 106 CFU per mL for further experiments.
Surfaces for biofilm tests
Four copper alloys were tested (Table 1). Elemental copper (100% Cu) was used as positive control and stainless steel AISI 316 (SS316) was used as negative control (0% Cu). Coupons (0.2 × 1 × 1 cm) of these surface materials (Fig. 1, Tables S.1 and S.2†) were used as substratum for biofilm formation. In order to clean and sterilize copper materials, coupons were degreased in absolute ethanol (Panreac Applichem, Darmstadt, Germany) and cleaned for 5 min in an ultrasound bath (70 W, 35 kHz, Ultrasonic Bath T420, Elma, Singen, Germany). To remove surface oxides, each coupon was exposed to HCl (39% sp gr 1.19 from Fisher, Leicestershire, UK) diluted two times in water, with 2 min exposure. To conclude oxides removal, coupons were washed in distilled water, dried with paper towel and abraded with abrasive paper P1000 until achieving a homogeneous appearance.35 Afterwards, the coupons were rinsed thoroughly with distilled water and dried with paper. After this process, coupons were washed for 20 min with ethanol at 70% (v/v) and further sterilized by ultraviolet (UV) for 45 min on each coupon face. To assess the efficiency of sterilization, two coupons of each alloy were inserted in 24 wells microtiter plates containing fresh R2A broth medium and incubated overnight in order to assess if some contamination remained, detecting microbial growth.
Table 1 Composition of materials used as substrate for biofilm formation (data provided by the suppliers: Novacimnor, Porto, Portugal; Universal AFIR, Porto, Portugal and Neves & Neves, Trofa, Portugal)
Material (US standard) |
Surface material composition (%) |
Cu |
Fe |
Ni |
Mn |
Sn |
Al |
Zn |
Cr |
Other |
AISI 316 |
— |
62 |
10–14 |
2 |
— |
— |
— |
16–18 |
C, Si, P, S, Cr Mo, N |
C11000 |
100 |
— |
— |
— |
|
|
|
|
— |
C18000 |
96 |
— |
4 |
— |
|
|
|
|
— |
C90800 |
87 |
0.03 |
— |
— |
12 |
— |
0.03 |
— |
Pb (0.62), Zn (0.03), P (0.32) |
C95500 |
79 |
— |
5 |
1 |
|
10 |
|
|
— |
C38500 |
57 |
5 |
— |
— |
|
|
39 |
|
Pb (0.03) |
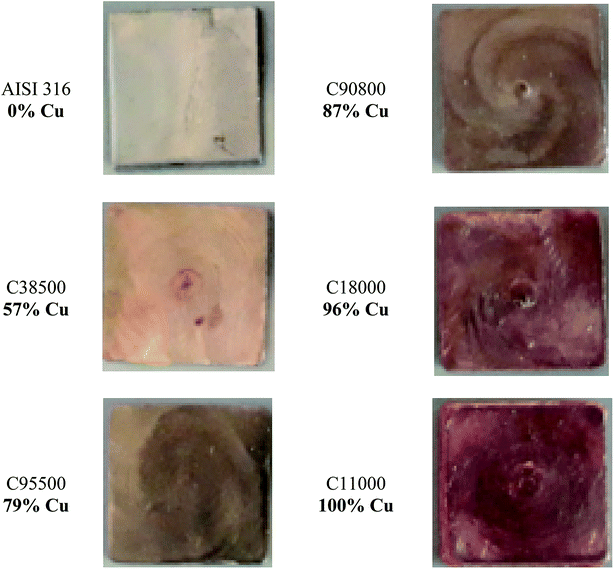 |
| Fig. 1 Materials used for biofilm formation. | |
Biofilm formation
Single and dual species biofilms were formed on coupons of each material (Fig. 1) inserted in 24 wells microtiter plates. Six coupons of each material were inserted in 24 wells microtiter plates and a bacterial suspension, prepared as described above, was added to each well. For single species biofilms, 1 mL of bacterial suspension was added to each well. To prepare dual species biofilms, 500 μL of each bacterial suspension was added to each well in order to keep the same final bacterial concentration. Microtiter plates were then incubated at 25 °C and 120 rpm for 24 h. After this period of incubation, two coupons of each material were removed to assess biofilm culturability. The remaining coupons were transferred to a new microtiter plate with new sterile STW, and incubated for additional 24 h at 25 °C and under agitation (120 rpm). After this incubation period (48 h of biofilm formation), two additional coupons of each material were removed to assess biofilm culturability and viability. The remaining colonized coupons were inserted in new 24 wells microtiter plates containing R2A in order to evaluate the ability of bacteria to regrowth from a sudden increase in nutrient availability. For this, coupons in R2A broth were incubated for additional 24 h at 25 °C and 120 rpm. Three independent experiments with duplicates were performed for each condition tested.
Biofilm culturability
Culturability was assessed for 24 and 48 h old biofilms. Colonized coupons were washed in 1 mL of STW in new 24 wells microtiter plates in order to remove weakly or non-adhered bacteria. Each coupon was inserted in a 50 mL centrifuge tube containing 3.5 mL of saline water (8.5 g L−1 of NaCl) after being scrapped with a micropipette tip while 1 mL of saline water was dispensed on the coupon to help the removal of the scrapped cells.32 Then, 0.5 mL of thiosulphate (0.5% w/v) was added to the tube containing the scrapped suspension in order to neutralize the effects from the copper ions released.36 Tubes containing coupons were then vortexed for 2 min to complete the removal of adhered bacteria and to dissociate possible bacterial aggregates.32 Serial dilutions from the obtained suspension were then performed and plated on R2A agar (Oxoid, Hampshire, UK) plates. Colony forming units (CFU) were enumerated after incubation for 48 h at 25 °C. For dual species biofilms, the number of CFU per cm2 was also assessed for each bacterium. This was possible because the selected bacteria had clearly distinct colony morphologies when grown on R2A agar. The detection limit for CFU enumeration was 2 log CFU per cm2.
Biofilm viability
The viability of 48 h old biofilms was assessed through the Live/Dead Bac light bacterial viability kit (Invitrogen Life Technologies, Alfagene). This kit is composed of two nucleic acid-binding stains: SYTO 9™ that penetrates all bacterial membranes and stains cells green and propidium iodide (PI) that only penetrates bacteria with damaged membranes and stains bacteria red. Biofilm samples, resuspended as described previously, were filtered through a Nucleopore (Whatman, Middlesex) black polycarbonate membrane with 0.22 μm pore size. Filtered samples were stained with 250 μL of SYTO 9™ and 50 μL of PI and left in the dark for 10 min. Samples were analyzed using a LEICA DM LB2 epifluorescence microscope connected to a Leica DFC300 FX camera (Leica Microsystems Ltd, Heerbrugg). The optical filter combination for optimal viewing of the stained preparations consisted of a 515–560 nm excitation filter combined with a dichromatic mirror at 580 nm and a suppression filter at 590 nm. Viable (green stained) and non-viable (red stained) cells were assessed from counts of a minimum of 20 fields of view. The total number of cells corresponds to the sum of viable (green stained) and non-viable (red stained) cells.
Viable but non-culturable bacteria were determined by the differences between the viable bacteria (green stained) and the numbers of CFU grown on R2A plates – for the same sample. The detection limit for this microscopic method is 5 log cells per cm2.
Biofilm regrowth
The regrowth of 48 h biofilm in terms of CFU numbers was evaluated after inserting the colonized coupons in a new microtiter plate containing medium with higher content of nutrients: R2A broth. The biofilm in R2A were incubated for additional 24 h at 25 °C under agitation (120 rpm) and regrowth was assessed in terms of culturability in R2A agar plates, as described previously.
Reactive oxygen species
The formation of reactive oxygen species (ROS) was evaluated when A. calcoaceticus and S. maltophilia were in contact with the different surface materials for 24 h. Dichloro-dihydro-fluorescein diacetate, H2DCFDA, (from Sigma-Aldrich, Steinheim, Germany) was used to determine ROS formation. This molecule is oxidized by ROS inside cell membrane resulting in a fluorescent molecule – 2′,7′-dichlorofluorescein.37 Bacteria were grown overnight at 25 °C and 120 rpm. Bacterial suspensions were centrifuged (3777 × g for 15 min), resuspended in phosphate buffered saline (PBS) and the bacterial concentration adjusted to 5 × 107 CFU per mL. H2DCFDA prepared in absolute ethanol, was added to the suspensions to a final concentration of 10 μM, and incubated in the dark for 20 min. Afterwards, bacteria were washed by centrifugation (3777 × g for 15 min) to remove the excess of H2DCFDA and resuspended in STW.38 A volume of 1 mL of bacterial suspension was added to each well of a 24 wells microtiter plate, containing coupons of the different materials. Two controls were performed: only with STW (a negative control without bacterial growth) and with bacteria not marked with H2DCFDA (to evaluate the possible autofluorescence of bacteria unrelated to the formation of ROS). Plates were incubated for 24 h at 25 °C and 120 rpm. Then coupons were vortexed in 5 mL of PBS for 2 minutes and the bacteria were sampled and analyzed in a 96-well microtiter plate using a FluoStar Omega (BMG Labtech, Madrid, Spain) reader under fluorescence (excitation wavelength: 485 nm and emission: 530 nm).
Copper materials corrosion and leaching
Corrosion of copper and copper alloys was evaluated according to the standard practice for preparing, cleaning and evaluating corrosion test specimens.35 Coupons were cleaned as described previously and dried at 60 °C for 30 min. Corrosion was evaluated for 6 months in STW without chlorine and in STW with 1 mg L−1 of free chlorine (residual concentration commonly present in drinking water39). Chlorine was replenished every week in order to keep a constant concentration, adding 0.23 mg L−1 of free chlorine according to the chemical stability studies (at 25 °C) performed by Meireles et al.40 Several time points were analyzed: 14 d, 21 d, 1 month, 2 months, 3 months, 4 months, 5 months and 6 months. Coupons were inserted in 12 wells microtiter plates and incubated at 25 °C under static conditions. Two coupons were removed in each time point, sonicated for 10 min in order to remove corrosion products. Then, the remaining corrosion product were removed with HCl (39% VWR chemicals, Fontenay-sous-Bois) diluted twice in water for 2 min at 25 °C.35 The coupons were weighed after being washed with distilled water and dried. This procedure was repeated three times until complete removal of corrosion products. The corrosion rate is given by eqn (1). |  | (1) |
where CR is defined in mils per year, W is the mass loss in g, A is the coupon area in cm2, T is the exposure time in hours and D is the density of the material in g cm−3.
To assess copper leaching, bulk water was collected at each time point and copper concentration was determined by flame emission and atomic absorption spectroscopy with hollow-cathode lamp using a GBC AAS 932 plus device with GBC Avante 1.33 software.
Statistical analysis
IBM® SPSS® Statistics (Statistical Package for the Social Sciences) version 24.0 was used for the statistical analysis of data applying the one-way analysis of variance (ANOVA). The comparisons between and within experimental groups were carried out using Tukey test. Statistical calculations were based on confidence level ≥95% (P < 0.05) which was considered statistically significant.
Results
Copper effect on single and dual species biofilms
Culturable bacteria.
Biofilm formation was found in all the surface materials tested. However, copper and copper alloys decreased significantly the culturability of both bacteria in single and dual species biofilms (P < 0.05). S. maltophilia in single species biofilms (Fig. 2B) were more tolerant to copper contact than A. calcoaceticus (Fig. 2A). In fact, no CFU of A. calcoaceticus single species biofilms were found on copper materials after 24 and 48 h incubation (Fig. 2A). On the other hand, significant reductions (>3.5 log CFU per cm2) were observed in S. maltophilia culturability for 24 h old biofilms formed on the copper alloys (P < 0.05). Nevertheless, after 48 h it was observed increased S. maltophilia biofilm control resultant from the use of the copper materials. Alloys with 57 and 79% copper reduced completely the culturability of 48 h old S. maltophilia single species biofilms. All the other copper materials had similar ability to reduce culturability of 48 h old S. maltophilia single species biofilms (>3.9 log CFU per cm2) (P > 0.05).
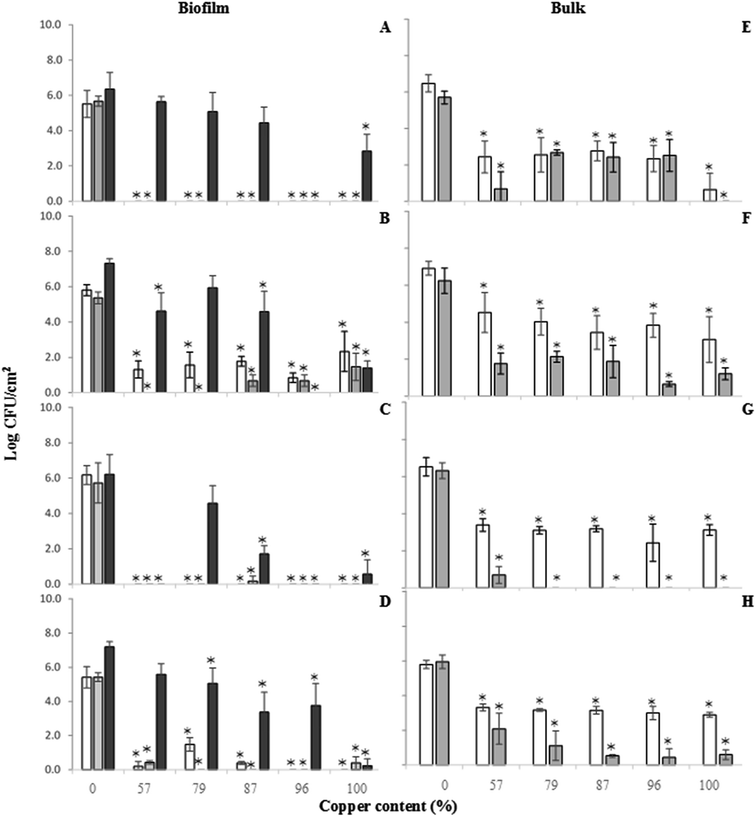 |
| Fig. 2 Log CFU per cm2 of biofilms formed on surfaces with different copper content (0, 57, 79, 87, 96 and 100% Cu) and the corresponding bulk phase culturability. (□) 24 h biofilms formed using STW, ( ) 48 h biofilm formed using STW, (■) biofilm regrowth in the presence of high nutrient levels (R2A broth). Left figures (A–D) correspond to biofilm culturability. Right figures (E–H) correspond to bulk phase culturability. (*) Differences statistically significant (P < 0.05) in comparison to SS316 (0% of copper). A. calcoaceticus in single species biofilms (A), S. maltophilia in single species biofilms (B), A. calcoaceticus in dual species biofilms (C), S. maltophilia in dual species biofilms (D), A. calcoaceticus present in bulk phase from single species biofilms (E), S. maltophilia present in bulk phase from single species biofilms (F), A. calcoaceticus in bulk phase from dual species biofilms (G) and S. maltophilia in bulk phase from dual species biofilms (H). | |
Regarding dual species biofilms (Fig. 2C and D), all copper surfaces were very effective in reducing 24 and 48 h old A. calcoaceticus culturability (>5.6 log CFU per cm2). For S. maltophilia, all reductions obtained were >5 log CFU per cm2. The exception was the alloy with 79% copper that reduced S. maltophilia culturability of 24 h old dual species biofilms in 3.93 log CFU per cm2. Also, in dual species biofilms it was possible to observe an increase of copper efficiency along time. Higher reductions of S. maltophilia culturability were observed after 48 h of contact with the copper alloys.
Viable and total bacteria.
The viability of 48 h old biofilms grown on the copper alloys was further characterized, in addition to the total number of adhered cells (Fig. 3). The total number of adhered A. calcoaceticus in single species biofilms slightly decreased on copper materials (P < 0.05) (Fig. 4A). Viable A. calcoaceticus was observed in all the biofilms formed. However, all the copper materials significantly reduced the number of viable A. calcoaceticus compared to SS316 (P < 0.05) (Fig. 4A). The lowest reduction (2.08 log cells per cm2) was obtained with the 57% copper alloy (P < 0.05). On the other hand, the highest reduction of A. calcoaceticus viability (4.68 log cells per cm2) was obtained using the 79% copper alloy.
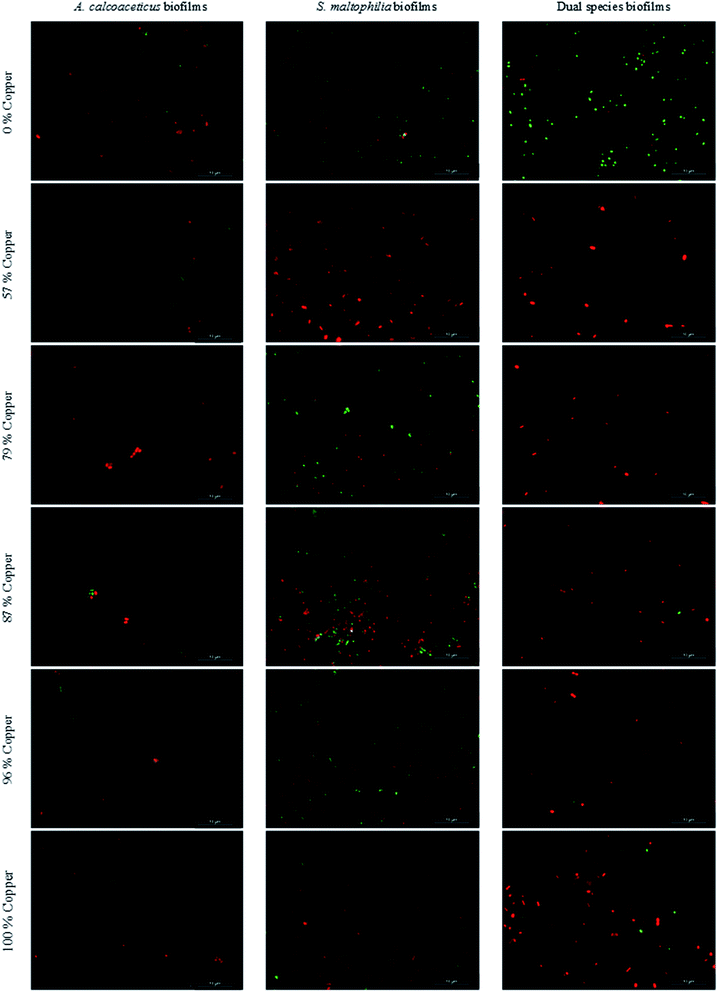 |
| Fig. 3 Viability of 48 h old biofilms formed on surface materials with different copper content (0, 57, 79, 87, 96 and 100% Cu). Images from fluorescence microscopy using a LEICA DM LB2 epifluorescence microscope connected to a Leica DFC300 FX camera (Leica Microsystems Ltd, Heerbrugg). Magnification of ×1000, scale bar of 10 μm. Bacteria were stained with using Live/Dead Baclight® kit. Red stained bacteria correspond to non-viable bacteria (with membrane damaged) and green stained bacteria correspond to viable bacteria (without membrane damaged). Images demonstrate a decrease in green stained bacteria on copper materials for all the biofilms tested and in comparison to the biofilms formed on SS316. The total numbers of A. calcoaceticus decreased on the copper materials in comparison to SS316. The total numbers of S. maltophilia was not remarkably influenced by the type of copper material, even if a decrease in viability (green stained bacteria) was observed for biofilms formed on 57 and 100% copper materials. | |
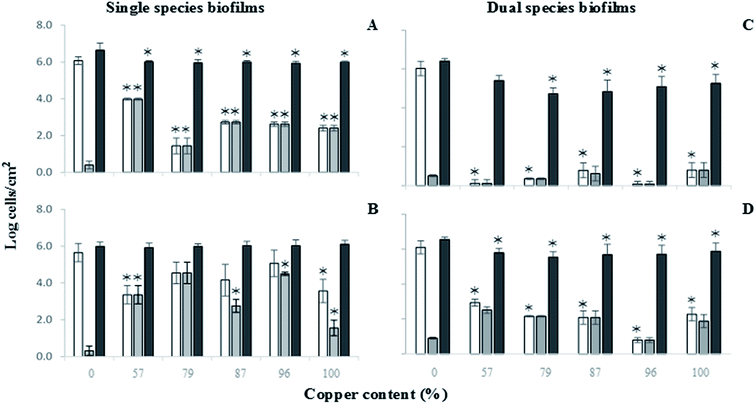 |
| Fig. 4 Viability (log cells per cm2) of 48 h biofilms formed on surface materials with different copper content (0, 57, 79, 87, 96 and 100% Cu). (□) Viable cells (VC), ( ) viable but non-culturable (VBNC) bacteria, (■) total cells (TC). Left figures (A and B) correspond to single species biofilm viability. Right figures (C and D) correspond to dual species biofilm viability. (*) Differences statistically significant (P < 0.05) in comparison with SS316 (0% copper). | |
The total number of S. maltophilia in 48 h old single species biofilms was not affected by the surface material used (Fig. 4B) (P > 0.05). Nevertheless, reductions of viable cells were observed for all the copper materials, even if at a lower extent than that observed for A. calcoaceticus (P < 0.05). The higher reductions were obtained with 57% copper alloy (2.29 log cells per cm2) and elemental copper (100% Cu) (2.08 log cells per cm2) (P < 0.05).
A slight decrease in the number of total adhered bacteria caused by copper materials was observed for A. calcoaceticus in 48 h old dual species biofilms (Fig. 4C) (P < 0.05). The exception was the 57% copper alloy (P > 0.05). High viability reductions of A. calcoaceticus in dual species biofilms (>5.2 log cells per cm2) were obtained for all copper materials tested.
The total number of S. maltophilia in dual species biofilms was slightly reduced when the copper surface materials were used (Fig. 4D) (P < 0.05). S. maltophilia in dual species biofilms was also more susceptible than in single species biofilms (P < 0.05). The higher reduction in viable S. maltophilia was obtained using 96% copper alloy (5.31 log cells per cm2) (P < 0.05), followed by alloys with 87, 79, 100 and 57% copper, with reductions of 4.01, 3.94, 3.82 and 3.16 log cells per cm2, respectively.
Viable but non-culturable bacteria.
The presence of viable but non-culturable (VBNC) bacteria was determined from the difference between viable cells and CFU. In general, copper surface materials increased the numbers of VBNC of A. calcoaceticus in single species biofilms compared to SS316. The higher amount of VBNC was found when using 57% copper alloy (P < 0.05). It was observed that the number of VBNC follows the same tendency found for viable cells, regardless the copper alloy. Also, in S. maltophilia biofilms it was observed an increase in VBNC counts from biofilm growth on the copper materials in comparison to SS316. The higher numbers of VBNC (4.55 and 4.50 log cells per cm2) were found on 79 and 96% copper alloys, respectively. Also, in dual species biofilms the numbers of A. calcoaceticus in VBNC state was similar to the numbers of viable cells (P > 0.05). However, in this particular case, the numbers of A. calcoaceticus in VBNC state was similar on SS316 and on the copper materials. It was also observed that VBNC of A. calcoaceticus in dual species biofilms was much lower than for the same bacterium in single species biofilms. On the other hand, dual species biofilms formed on copper materials were composed of higher amounts of VBNC S. maltophilia than these biofilms formed on SS316 (P < 0.05), with the exception of 96% copper alloy where the numbers of VBNC S. maltophilia was similar to these observed on SS316 (P > 0.05). Also, in this situation the VBNC follows the same tendency observed for viable cell counts (P > 0.05). In general, the number of VBNC bacteria was lower in dual species biofilms formed on copper alloys than in single species biofilms.
Bacterial regrowth on copper materials.
It was observed that A. calcoaceticus single species biofilms (Fig. 2A) were unable to recover its culturability when biofilms formed on 96% copper alloy were exposed to a sudden increase of nutrients (R2A broth). On the other hand, the regrowth of A. calcoaceticus culturability was similar on SS316 (0% Cu) and on 57 and 79% copper alloys (P > 0.05). The elemental copper surface (100% Cu) was able to reduce the A. calcoaceticus regrowth in 3.52 log CFU per cm2 compared to SS316.
Regarding S. maltophilia single species biofilms, it was possible to observe that only 96% copper alloy was able to completely inhibit regrowth (Fig. 2B). Elemental copper had also an important role in the impairment of S. maltophilia regrowth with a difference of 5.93 log CFU per cm2 compared to SS316 (P < 0.05). Alloys with 57 and 87% of copper caused impairment of S. maltophilia biofilm regrowth in 2.71 and 2.74 log CFU per cm2, respectively (P > 0.05).
In dual species biofilms, regrowth of A. calcoaceticus only was found on materials with 79, 87 and 100% copper (Fig. 2C). These alloys reduced A. calcoaceticus regrowth in 1.64, 4.51 and 5.66 log CFU per cm2 compared to SS316, respectively. No regrowth was found on alloys with 57 and 96% copper. S. maltophilia regrowth in dual species biofilms occurred in all the copper materials tested (Fig. 2D). The lowest S. maltophilia regrowth was observed for elemental copper (100% Cu) with a reduction of 6.96 log CFU per cm2 compared to SS316 (P < 0.05). Reductions of 1.61, 2.14, 3.83, and 3.43 log CFU per cm2 on the ability of S. maltophilia to recover were observed when using 57, 79, 87 and 96% copper alloys, respectively (P > 0.05).
Copper effects in the bulk bacteria
The culturability of bacteria present in the bulk phase was also analyzed at 24 and 48 h. Bacteria detected in the bulk phase after 24 h of incubation in the presence of copper materials correspond essentially to culturable non-adhered bacteria. On the other hand, bacteria detected in the bulk phase at 48 h correspond to culturable biofilm-released bacteria. All the copper materials reduced the numbers of A. calcoaceticus CFU detected in the bulk phase (Fig. 2E) (P < 0.05). Regarding the effects on the released bacteria for 48 h, no A. calcoaceticus CFU were observed in the bulk phase when elemental copper (100% Cu) was used. The 57% copper alloy caused 5.02 log CFU per cm2 reduction (P < 0.05) in bulk phase when compared with SS316. All the other materials caused similar A. calcoaceticus CFU reductions when analyzing the bulk phase (>3.80 log CFU per cm2) (P > 0.05).
Concerning S. maltophilia single species biofilms (Fig. 2F), it was possible to observe that all the copper materials had similar impact on the non-adhered bacteria (24 h), being the numbers of CFU in the bulk phase lower on copper materials than on SS316 (P > 0.05). The 96% copper alloy was the one causing the highest CFU reduction (5.59 log CFU per cm2) in the bulk phase for the 48 h analysis. The other copper alloys caused similar CFU reductions (between 4.11 and 5 log CFU per cm2) (P > 0.05).
Fig. 2G and H show culturability results of A. calcoaceticus and S. maltophilia in the bulk phase when dual species biofilms were present, respectively. All the copper materials were able to reduce significantly the culturability of non-adhered A. calcoaceticus (Fig. 2G) and S. maltophilia (Fig. 2H) for 24 h analysis (P < 0.05). No A. calcoaceticus were found in the bulk water for the 48 h analysis, for all the copper materials tested. The culturability of released S. maltophilia (48 h – Fig. 2H) was also significantly reduced when copper surface materials were used (P < 0.05). The highest reductions (>5.3 log CFU per cm2) were observed for the 87, 96 and 100% copper alloys.
Formation of reactive oxygen species
The generation of ROS by A. calcoaceticus and S. maltophilia biofilms was monitored using DCFH-DA. This molecule reacts with ROS present inside the cells and is converted into DCF (a fluorescent by-product). Therefore, fluorescence was evaluated to monitor ROS formation. It is possible to observe higher formation of ROS in 24 h old S. maltophilia biofilms than in A. calcoaceticus (Fig. 5) (P < 0.05). The formation of ROS inside S. maltophilia increased when exposed to materials with higher copper content (87, 96 and 100% Cu) (P < 0.05). Regarding A. calcoaceticus biofilms, the highest ROS formation was observed for the alloy with the lowest copper content (57% Cu) (P < 0.05).
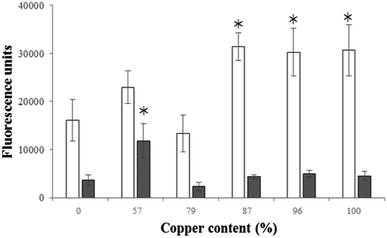 |
| Fig. 5 Reactive oxygen species (ROS) formation in A. calcoaceticus ( ) and S. maltophilia (□) from biofilms formed on the surface materials with different copper contents. (*) Differences statistically significant (P < 0.05) in comparison with SS316 (0% copper). | |
Copper materials corrosion and leaching
The corrosion rate of all copper materials was found to be higher during the first month of contact with STW (Fig. 6). Afterwards, the corrosion rate stabilized for all the materials, except for elemental copper that presented an increase in the corrosion level after 90 d in STW (Fig. 6A). In the absence of chlorine, all the materials demonstrated similar behavior in terms of copper leaching (Fig. 7): copper concentrations did not exceed the values recommended by WHO/EU Directive 98/83/EC (2 mg L−1)25 and the EPA Standard (1.3 mg L−1). No copper was detected in the bulk water for the alloy with 57% of copper ([Cu+/Cu2+] < 0.3 mg L−1). The presence of chlorine did not affect the leaching from materials with 57, 79, 96 and 100% (P > 0.05). On the other hand, the exposure of the 87% copper alloy to chlorine in STW increased significantly (P < 0.05) copper leaching (Fig. 7B). After 90 d of contact with chlorinated water, the copper concentration in stagnated water increased to values higher than those recommended by the WHO/EU Directive 98/83/EC (2 mg L−1)25 and the EPA Standard (1.3 mg L−1).
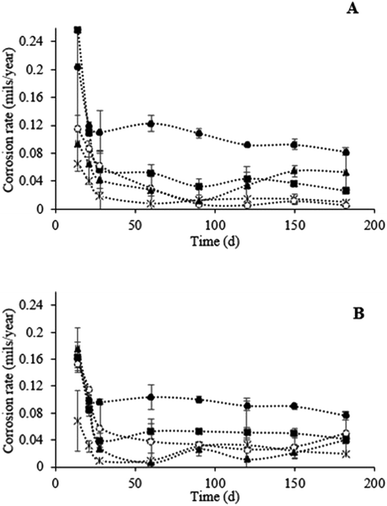 |
| Fig. 6 Corrosion rate of copper materials in STW (A) and in STW with 1 mg L−1 of free chlorine (B) for 182 days. (●) 57% copper alloy; (*) 79% copper alloy, (■) 87% copper allot; (○) 96% copper alloy; (▲) elemental copper (100% copper). The assay was performed with three replicates. | |
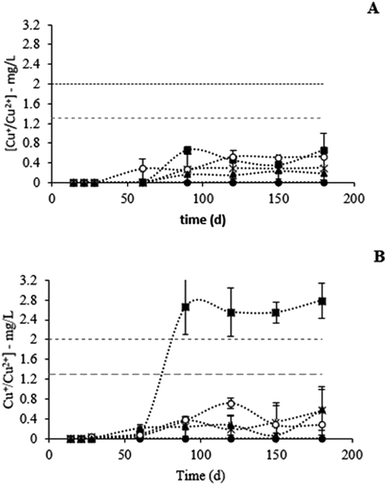 |
| Fig. 7 Copper leaching to STW in the absence (A) and presence of 1 mg L−1 of free chlorine (B) for 182 days. (●) 57% copper alloy; (*) 79% copper alloy, (■) 87% copper alloy; (○) 96% copper alloy, (▲) elemental copper (100% copper). ( ) Maximum copper concentration in DW as recommended by WHO/EU Directive 98/83/EC (2 mg L−1). ( ) Maximum copper concentration in DW as recommended by EPA Standard (1.3 mg L−1). The detection limit of the analytical method used was 0.3 mg L−1. The assay was performed with three replicates. | |
Discussion
A. calcoaceticus and S. maltophilia were used as model microorganisms from DWDS.31 These two bacteria were selected for this work taking into account their frequent presence in drinking water and their ability to infect immunocompromised consumers. In fact, Acinetobacter spp. and S. maltophilia were already described by the World Health Organization41 as causative agents of infections in immunocompromised patients through tap water. Several other works described the isolation of Acinetobacter species42–44 and S. maltophilia45–49 from drinking water. Moreover, S. maltophilia is widespread in the environment and has an intrinsic multidrug resistance profile hindering infections treatment.50–52
The selection of pipe materials is a critical aspect for the chemical and microbiological stability of plumbing systems. Several characteristics should be ensured to guarantee a safe plumbing system: controlled microbial proliferation – including biofilm development; no leaching of products to the drinking water; low installation and material costs; and high life-time.53 Copper is a metal used in plumbing systems for decades and its antimicrobial properties have been described as advantageous.54 Nevertheless, copper expensive cost is one of the main reasons to increase the use of plastic materials in plumbing systems.55,56 Copper leaching to drinking water and its possible negative effects on the water quality and public health also contributed to the misuse of this material.22,54 Nevertheless, the choice between copper or plastic pipes is still controversial.57 Although plastic pipes have lower installation costs, they usually have a shorter life-time and can be responsible for the leaching of some chemicals to the drinking water, changing the water characteristics and causing some concern to consumers' health, including potential carcinogenic effects and the favoring the growth of pathogens.26,55,58 The present work aims to screen alloys with different copper contents (0, 57, 79, 87, 96 and 100%) in order to understand the possibility of using less expensive copper materials in plumbing systems to reduce biofilm development – guaranteeing the microbial and chemical quality of the delivered water. The corrosion rate of each material was also evaluated as well as copper leaching to the bulk water.
The present results demonstrated that copper materials were consistently more efficient in controlling biofilms of A. calcoaceticus than of S. maltophilia. In fact, this effect was not evident in the bulk phase. No A. calcoaceticus CFU were detected after single species biofilm development on copper materials. This result corroborates the study of Różańska et al.59 where no CFU of Acinetobacter species were detected on copper (100% Cu) after 5 h of contact with this material. Moreover, significant reductions were observed when Acinetobacter species were in contact with 63 and 94% copper surfaces for 5 h. S. maltophilia single species biofilms were more tolerant to copper and CFU were detected in 24 h old biofilms formed on all the surface materials tested. Previous studies already described some strains of S. maltophilia tolerant to copper and able to bioremediate this metal.52,60,61 Moreover, S. maltophilia biofilms were found to be more tolerant to antimicrobials than these of A. calcoaceticus.62,63
It is important to highlight that copper materials reduced significantly the culturability for all the scenarios tested, even when using the alloy with the lowest copper content. However, the control efficiency of S. maltophilia in single and dual species biofilms was slightly increased when the contact time with copper surfaces increased for 48 h. Moreover, no relationship was observed between CFU reduction and surface copper content. Morrier et al.64 also found that antimicrobial activity of dental amalgams was not related to copper content. For instance, in the present study it was observed that, in particular cases, alloys with lower copper content demonstrated better results than those with higher content. As example, no CFU were detected for 48 h old S. maltophilia biofilms formed on 57 and 79% copper alloys. However, complete inactivation of S. maltophilia was not achieved using the higher copper content materials (87, 96 and 100%). These different behaviors may not be exclusively associated to the copper content, which reinforces the importance to evaluate the possibility of using and/or developing alloys with reduced copper content for application in plumbing systems. The presence of other metals such as zinc and lead64,65 in the alloy with 57% copper may also have an important impact in biofilm control. It is important to note that the referred alloy has high percentage of zinc (39%). The alloy containing 79% copper is composed of 10% aluminum, which may improve copper action. A recent work described the importance of dissolved aluminum in the amplification of the toxicity of transition metals, such as copper, which helps to understand the higher CFU reductions when alloys containing both metals were used66. Moreover, the hypothesis of aluminum interaction with bacterial membrane phospholipids cannot be disregarded.67
The antimicrobial action of copper surfaces has been investigated and several effects on bacteria integrity were described such as damage of membrane, copper intake (due to dissolution of copper ions from surfaces), damage caused by oxidative stress, cell death and DNA degradation.8,68 In the present work, the copper action was evaluated using biofilms developed in synthetic drinking water. Most of the previous works on copper mode of action focused the contact killing effects on dry surfaces or used planktonic bacteria.68–71 Copper leaching to STW, membrane damage assessment by propidium iodide staining and the oxidative stress effects were evaluated in order to understand how copper alloys exerted antimicrobial action. In general, higher membrane damage was found in A. calcoaceticus compared to S. maltophilia, and membrane damage was not related to surface copper content. The formation of ROS was higher in S. maltophilia than in A. calcoaceticus, regardless the surface material used. However, S. maltophilia was the bacteria more tolerant to copper. It is possible that S. maltophilia in biofilms expressed defense mechanism to survive ROS formation. In fact, previous works described efflux systems, such as SmeYZ and SmeU1VWU2X, able to improve S. maltophilia tolerance to oxidative stress.51,72 The antimicrobial action also seems to be independent from the copper leached to water, as no relationship was observed between the numbers of culturable bacteria in the bulk water and the surface copper content or its concentration in the bulk water. Based on the results from analysis of the bacteria in both bulk phase and in biofilms, it is possible to observe that the action of copper is more effective on the surface than in the bulk water. Complete inactivation of non-adhered bacteria was not obtained with any material tested. This proposes that the antimicrobial action of the copper materials was not driven by metal leaching to the bulk water. This hypothesis is reinforced by results from copper leaching in the absence of chlorine. The concentration of copper was below the maximum levels recommended by EPA23 and WHO24 (1.3 mg L−1 and 2 mg L−1) in unchlorinated STW. Higher copper concentrations were detected in STW exposed to the 87% copper alloy. In chlorinated systems, the copper leaching from this alloy is of potential concern. It exceeds the maximum concentration recommended by WHO and EPA.23,24 Therefore, the results propose that the alloy with 87% copper content should not be considered for application in chlorinated plumbing systems.
Although the evaluation of culturability is the standard method commonly applied to control the microbiological quality of drinking water,73 viability tests should also be evaluated to infer on the biofilm prevention efficacy of the materials tested. It is known that culturability analysis do not provide accurate information about the microbiological quality of drinking water. Under adverse environments, bacteria may acquire a “dormant state” – the VBNC bacteria that survive under adverse conditions and are metabolically active but unable to growth on solid growth media and form colonies. These VBNC bacteria may recover its culturability and proliferate in water when in the presence of more favorable conditions, compromising the water quality. Bacterial regrowth in drinking water is also a common problem, imposing the use of residual chlorine along DWDS. Therefore, VBNC and bacterial regrowth were also assessed in this work. Biofilm regrowth was assessed by studying bacterial ability to recover its culturability when colonized coupons were in the presence of R2A broth for 24 h. The alloy containing 96% copper was the most promising material avoiding regrowth. The exception was S. maltophilia in dual species biofilms that was able to recover on this alloy. Nevertheless, this result seems not be related to the number of VBNC, as viable and VBNC bacteria were detected in all the conditions tested. Viable counts and VBNC detected in the alloy with 96% copper did not recover its culturability, contrary to the observed with other alloys. This proposes that the extent of injury of VBNC bacteria in the presence of 96% copper alloy is harder to be overcome, even when exposed to high nutrient conditions. Dwidjosiswojo et al.74 and Bedard et al.75 found that VBNC of P. aeruginosa exposed to copper ions recovered culturability when copper chelators were used. Dwidjosiswojo et al.74 also demonstrated that copper in plumbing systems was responsible for the induction of a VBNC state in P. aeruginosa. Nevertheless, their study demonstrated that copper at concentrations similar to those detected in plumbing systems was unable to damage the bacterial membrane. Gião et al.16 also found that copper increased the number of Legionella pneumophila in VBNC state. The efficiency of copper alloys seems not to be dependent on copper leaching to the bulk water or even on the ROS production but depends on the contact time, as it was observed by the reduction of CFU of S. maltophilia single and dual species biofilms after 48 h in contact with copper materials.
Conclusions
In conclusion, all the copper surface materials reduced culturability and viability of A. calcoaceticus and S. maltophilia from single and dual species biofilms. The most promising results were obtained using the 96% copper alloy. This had antimicrobial effects and avoided biofilm regrowth. Additionally, it presented low corrosion rate and leaching, even in the presence of chlorine. Bacterial membrane damage and the formation of ROS was verified for all the selected copper alloys. It is important to highlight that alloys containing lower copper content were also efficient in impairing biofilm formation, with results comparable to elemental copper. These alloys are potentially relevant for plumbing systems, helping to reduce installation costs due to the lower copper content. The exception was the 87% copper alloy that was prone to prohibitive leaching in the presence of chlorine. The impact of copper alloys in DW biofilm control intensifies the need for further research about the combined effect of different metal alloys in biofilm development and DW quality.
Conflicts of interest
Authors declare no conflict of interest.
Acknowledgements
This work was the result of the projects: UID/EQU/00511/2019 – Laboratory for Process Engineering, Environment, Biotechnology and Energy – LEPABE funded by national funds through FCT/MCTES (PIDDAC); POCI-01-0145-FEDER-030219; POCI-01-0145-FEDER-006939, funded by FEDER funds through COMPETE2020 – Programa Operacional Competitividade e Internacionalização (POCI) and by national funds (PIDDAC) through FCT/MCTES; NORTE-01-0145-FEDER-000005 – LEPABE-2-ECO-INNOVATION, supported by Norte Portugal Regional Operational Programme (NORTE 2020), under PORTUGAL 2020 Partnership Agreement, through the European Regional Development Fund (ERDF). Grants attributed by the Portuguese Foundation for Science and Technology – FCT – to Inês Gomes (SFRH/BD/103810/2014) and to Manuel Simões (SFRH/BSAB/150379/2019).
References
-
NRC, Drinking water distribution systems: Assessing and reducing risks, N.R.C., Washington, DC, 2006 Search PubMed.
- M. M. Critchley, N. J. Cromar, N. McClure and H. J. Fallowfield, Water Sci. Technol.: Water Supply, 2002, 2, 81–87 CAS.
- L. C. Simões and M. Simões, RSC Adv., 2013, 3, 2520–2533 RSC.
- D. Stalter, E. O′Malley, U. von Gunten and B. I. Escher, Water Res., 2016, 91, 19–30 CrossRef CAS.
- M. J. Plewa, J. E. Simmons, S. D. Richardson and E. D. Wagner, Environ. Mol. Mutagen., 2010, 51, 871–878 CrossRef CAS.
- J. Rogers, A. B. Dowsett, P. J. Dennis, J. V. Lee and C. W. Keevil, Appl. Environ. Microbiol., 1994, 60, 1842–1851 CAS.
- C. Zhang, C. Li, X. Zheng, J. Zhao, G. He and T. Zhang, Int. J. Environ. Sci. Technol., 2017, 14, 85–94 CrossRef CAS.
- G. Grass, C. Rensing and M. Solioz, Appl. Environ. Microbiol., 2011, 77, 1541–1547 CrossRef CAS.
- D. Roe, B. Karandikar, N. Bonn-Savage, B. Gibbins and J. B. Roullet, J. Antimicrob. Chemother., 2008, 61, 869–876 CrossRef CAS.
- A. A. Morvay, M. Decun, M. Scurtu, C. Sala, A. Morar and M. Sarandan, Water Sci. Technol.: Water Supply, 2011, 11, 252–257 CAS.
- J. Yu, D. Kim and T. Lee, Water Sci. Technol., 2010, 61, 163–171 CrossRef CAS.
- C. Jungfer, F. Friedrich, J. Varela Villarreal, K. Brandle, H. J. Gross and U. Obst,
et al.
, Biofouling, 2013, 29, 891–907 CrossRef CAS.
- M. J. Lehtola, I. T. Miettinen, M. M. Keinanen, T. K. Kekki, O. Laine and A. Hirvonen,
et al.
, Water Res., 2004, 38, 3769–3779 CrossRef CAS PubMed.
- J. Lu, H. Y. Buse, V. Gomez-Alvarez, I. Struewing, J. Santo Domingo and N. J. Ashbolt, J. Appl. Microbiol., 2014, 117, 905–918 CrossRef CAS.
- D. van der Kooij, H. R. Veenendaal and W. J. Scheffer, Water Res., 2005, 39, 2789–2798 CrossRef CAS.
- M. S. Gião, S. A. Wilks and C. W. Keevil, BioMetals, 2015, 28, 329–339 CrossRef.
- W. J. Rhoads, A. Pruden and M. A. Edwards, Environ. Sci. Technol., 2017, 51, 7065–7075 CrossRef CAS.
- P. M. Ashraf and L. Edwin, Int. Biodeterior. Biodegrad., 2016, 115, 39–48 CrossRef CAS.
- C.-L. Chen, J. S. Maki, D. Rittschof and S. L.-M. Teo, Int. Biodeterior. Biodegrad., 2013, 83, 71–76 CrossRef CAS.
- A. Reyes, M. V. Letelier, R. De la Iglesia, B. González and G. Lagos, Int. Biodeterior. Biodegrad., 2008, 61, 135–141 CrossRef CAS.
- D. A. Lytle and J. Liggett, Water Res., 2016, 92, 11–21 CrossRef CAS.
- I. T. Vargas, D. A. Fischer, M. A. Alsina, J. P. Pavissich, P. A. Pasten and G. E. Pizarro, Materials, 2017, 10, 1036 CrossRef.
-
EPA, Lead and copper rule revisions, U. S. Environmental Protection Agency – Office of Water, Washington DC, 2016 Search PubMed.
-
WHO, Copper in drinking water, background document for preparation of WHO guidelines for drinking water quality, World Health Organization, Geneva, 2003 Search PubMed.
-
European Committee, Council directive 98/83/EC Drinking water Directive, Official journal of the European Communities L330, 5.12.1998, p. 32 Search PubMed.
- M. Connell, A. Stenson, L. Weinrich, M. LeChevallier, S. L. Boyd and R. R. Ghosal,
et al.
, J.-Am. Water Works Assoc., 2016, 108, E192–E204 CrossRef.
- T. H. Merkel and S. O. Pehkonen, Corros. Eng., Sci. Technol., 2006, 41, 21–37 CrossRef CAS.
- H. Y. Buse, B. J. Morris, I. T. Struewing and J. G. Szabo, Appl. Environ. Microbiol., 2019, 85, e02956-18 CrossRef.
- J. Inkinen, B. Jayaprakash, M. Ahonen, T. Pitkanen, R. Makinen and A. Pursiainen,
et al.
, J. Appl. Microbiol., 2018, 124, 611–624 CrossRef CAS PubMed.
- H. J. Jang, Y. J. Choi and J. O. Ka, J. Microbiol. Biotechnol., 2011, 21, 115–123 CrossRef CAS.
- L. C. Simões, M. Simões, R. Oliveira and M. J. Vieira, J. Basic Microbiol., 2007, 47, 174–183 CrossRef PubMed.
- I. B. Gomes, L. C. Simões and M. Simões, Sci. Total Environ., 2018, 643, 1348–1356 CrossRef CAS.
-
EPA, Development and testing of methods to decontaminate a building's plumbing system impacted by a water contamination event: Decontamination of Bacillus spore, United States Environmental Protection Agency, USA, 2011 Search PubMed.
- K. Y. Patterson, P. R. Pehrsson and C. R. Perry, J. Food Compos. Anal., 2013, 31, 46–50 CrossRef CAS.
-
ASTM, ASTM G1-90- Standard Practice for Preparing, Cleaning, and Evaluating Corrosion Test Specimens, ASTM International, West Conshohocken, USA, 1999 Search PubMed.
-
Department of Health and social care, UK Government, Health technical memorandum 04-01: Safe water in healthcare premises. Part B: Operational management, 2016 Search PubMed.
- N. Jambunathan, Methods Mol. Biol., 2010, 639, 292–298 CrossRef.
- S. Dwivedi, R. Wahab, F. Khan, Y. K. Mishra, J. Musarrat and A. A. Al-Khedhairy, PLoS One, 2014, 9, e111289 CrossRef.
-
WHO, Chlorine in drinking water - Background document for development of WHO guidelines for drinking water quality, World Health Organization, Geneva, Switzerland, 2003 Search PubMed.
- A. Meireles, C. Ferreira, L. Melo and M. Simões, Food Res. Int., 2017, 102, 511–518 CrossRef CAS.
-
A. Glasmacher, S. Engelhart and M. Exner, Infections from HPC organisms in drinking-water amongst the immunocompromised, in Heterotrophic plate counts and drinking-water safety: The significance of HPCs for water quality and human health, ed. J. Bartram, J. Cotruvo, M. Exner, C. Fricker and A. Grasmacher, WHO/IWA, London, UK, 2003 Search PubMed.
- J. M. Bifulco, J. J. Shirey and G. K. Bissonnette, Appl. Environ. Microbiol., 1989, 55, 2214–2219 CAS.
- L. C. Simões, M. Simões and M. J. Vieira, Appl. Environ. Microbiol., 2008, 74, 1259–1263 CrossRef PubMed.
- C. Narciso-da-Rocha, I. Vaz-Moreira, L. Svensson-Stadler, E. R. B. Moore and C. M. Manaia, Appl. Microbiol. Biotechnol., 2013, 97, 329–340 CrossRef CAS PubMed.
- J. S. Cervia, G. A. Ortolano and F. P. Canonica, Clin. Infect. Dis., 2008, 46, 1485–1487 CrossRef.
- H.-Y. Shih and Y. E. Lin, Appl. Environ. Microbiol., 2010, 76, 2032–2035 CrossRef CAS.
- A. Guyot, J. F. Turton and D. Garner, J. Hosp. Infect., 2013, 85, 303–307 CrossRef CAS.
- R. I. Amoli, J. Nowroozi, A. Sabokbar and R. Rajabniya, Asian Pac. J. Trop. Biomed., 2017, 7, 826–830 CrossRef.
- S. Vincenti, G. Quaranta, C. De Meo, S. Bruno, M. G. Ficarra and S. Carovillano,
et al.
, Sci. Total Environ., 2014, 499, 47–54 CrossRef CAS PubMed.
- L. Zhang, X. Z. Li and K. Poole, Antimicrob. Agents Chemother., 2000, 44, 287–293 CrossRef CAS.
- Y.-T. Lin, Y.-W. Huang, S.-J. Chen, C.-W. Chang and T.-C. Yang, Antimicrob. Agents Chemother., 2015, 59, 4067–4073 CrossRef CAS.
- P. Mukherjee and P. Roy, Front. Microbiol., 2016, 7, 967 Search PubMed.
- Y. Lee, Int. J. Environ. Res. Public Health, 2013, 10, 4143–4160 CrossRef.
- A. Armanious and K. Johannsen, Mater. Corros., 2012, 63, 438–444 CrossRef CAS.
- K. M. Kelley, A. C. Stenson, R. Dey and A. J. Whelton, Water Res., 2014, 67, 19–32 CrossRef CAS.
-
M. Connell, A. Stenson and A. Whelton, Pipeline AL/MS AWWA Section Newsletter, 2013, vol. 1, pp. 15–20 Search PubMed.
- J. Lee, J. Water Supply: Res. Technol.--AQUA, 2015, 64, 326–332 CrossRef.
- V. Lund, M. Anderson-Glenna, I. Skjevrak and I.-L. Steffensen, J. Water Health, 2011, 9, 483–497 CrossRef CAS.
- A. Różańska, A. Chmielarczyk, D. Romaniszyn, G. Majka and M. Bulanda, Antimicrob. Resist. Infect. Contr., 2018, 7, 10 CrossRef.
- J. S. Brooke, Clin. Microbiol. Rev., 2012, 25, 2–41 CrossRef CAS.
- S. Tang, H. Yin, S. Chen, H. Peng, J. Chang and Z. Liu,
et al.
, J. Hazard. Mater., 2016, 308, 335–342 CrossRef CAS.
- I. B. Gomes, M. Simões and L. C. Simões, Sci. Total Environ., 2016, 565, 40–48 CrossRef CAS.
- I. B. Gomes, M. Lemos, L. Mathieu, M. Simões and L. C. Simões, Sci. Total Environ., 2018, 631–632, 987–993 CrossRef CAS.
- J. J. Morrier, G. Suchett-Kaye, D. Nguyen, J. P. Rocca, J. Blanc-Benon and O. Barsotti, Dent. Mater., 1998, 14, 150–157 CrossRef CAS.
- M. Yasuyuki, K. Kunihiro, S. Kurissery, N. Kanavillil, Y. Sato and Y. Kikuchi, Biofouling, 2010, 26, 851–858 CrossRef CAS.
- S. C. Londono, H. E. Hartnett and L. B. Williams, Environ. Sci. Technol., 2017, 51, 2401–2408 CrossRef CAS.
- R. G. Piña and C. Cervantes, BioMetals, 1996, 9, 311–316 CrossRef.
- C. E. Santo, E. W. Lam, C. G. Elowsky, D. Quaranta, D. W. Domaille and C. J. Chang,
et al.
, Appl. Environ. Microbiol., 2011, 77, 794–802 CrossRef CAS PubMed.
- P. Bleichert, C. Espírito Santo, M. Hanczaruk, H. Meyer and G. Grass, BioMetals, 2014, 27, 1179–1189 CrossRef CAS.
- A. Parra, M. Toro, R. Jacob, P. Navarrete, M. Troncoso and G. Figueroa,
et al.
, Braz. J. Microbiol., 2018, 49, 113–118 CrossRef CAS.
- S. L. Warnes, V. Caves and C. W. Keevil, Environ. Microbiol., 2012, 14, 1730–1743 CrossRef CAS.
- C. J. Wu, T. T. Chiu, Y. T. Lin, Y. W. Huang, L. H. Li and T. C. Yang, Antimicrob. Agents Chemother., 2018, 62, e02114-17 CrossRef.
-
WHO, Guidelines for drinking-water quality. Addendum: Microbiological agents in drinking water, WHO, Geneva, Switzerland, 2002 Search PubMed.
- Z. Dwidjosiswojo, J. Richard, M. M. Moritz, E. Dopp, H. C. Flemming and J. Wingender, Int. J. Hyg. Environ. Health, 2011, 214, 485–492 CrossRef CAS.
- E. Bedard, D. Charron, C. Lalancette, E. Deziel and M. Prevost, FEMS Microbiol. Lett., 2014, 356, 226–234 CrossRef CAS.
Footnote |
† Electronic supplementary information (ESI) available. See DOI: 10.1039/c9ra05880j |
|
This journal is © The Royal Society of Chemistry 2019 |